DOI:
10.1039/D2SC07023E
(Edge Article)
Chem. Sci., 2023,
14, 4397-4400
A convergent fragment coupling strategy to access quaternary stereogenic centers†
Received
22nd December 2022
, Accepted 25th February 2023
First published on 27th February 2023
Abstract
The formation of quaternary stereogenic centers via convergent fragment coupling is a longstanding challenge in organic synthesis. Here, we report a strategy for the formation of quaternary stereogenic centers in polycyclic systems based upon the semi-pinacol reaction. In the key transformation, two fragments of a similar size and complexity are joined by a 1,2-addition of an alkenyl lithium to an epoxy ketone, and the resulting epoxy silyl ether undergoes a semi-pinacol rearrangement catalyzed by N-(trimethylsilyl)bis(trifluoromethanesulfonyl)imide (TMSNTf2) or trimethylsilyl trifluoromethanesulfonate (TMSOTf). Polycyclic scaffolds were generated in high yields and the reaction conditions tolerated a variety of functional groups including esters, silyl ethers, enol ethers, and aryl triflates. This method provides a useful strategy for the synthesis of complex polycyclic natural product-like scaffolds with quaternary stereogenic centers from simplified fragments.
Convergent fragment coupling is a strategic approach that can rapidly generate complex molecules by joining fragments of a similar size and complexity.1 The independent synthesis of each fragment can be completed in parallel and this approach reduces the potential for competitive functional group reactivity. Many elegant syntheses have been developed utilizing a fragment coupling as a key strategic disconnection, often employing well established chemistry such as the Michael addition, the Diels–Alder reaction, transition metal-catalyzed cross-coupling, and 1,2-nucleophilic addition.2 A major limitation to these approaches is the challenge of accessing stereogenic quaternary carbons, which are common motifs in natural products.3 Stereocontrol at attached-ring quaternary centers represents an especially difficult task due to the lack of well-defined stereocontrol elements, in contrast to the more rigid ring topologies of bridging, spirocyclic, and fused ring systems.4
A powerful method to form quaternary stereogenic centers is the semi-pinacol rearrangement, a stereospecific reaction that can convert epoxy alcohols to α-quaternary ketones (Fig. 1a).5 The strategic application of the semi-pinacol rearrangement in total synthesis has predominately focused on skeletal rearrangement (for example, Fig. 1b) rather than as part of a fragment coupling tactic.6,7 There have been relatively few applications of the semi-pinacol rearrangement as fragment coupling strategies in total synthesis,8 which motivated us to develop a general set of conditions based upon our work towards the C19 diterpenoid alkaloids (Fig. 1c).8b
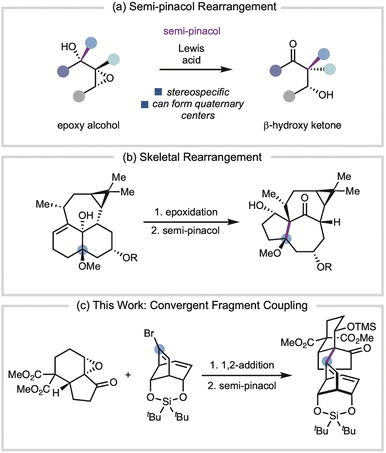 |
| Fig. 1 Synthetic strategies using the semi-pinacol rearrangement. | |
Our initial studies began with the investigation of the semi-pinacol rearrangement of epoxide 2a-OH, an intermediate in our synthesis of the diterpenoid alkaloid (−)-talatisamine.8b Treatment alcohol 2a–OH with 1.2 equiv. of TMSOTf at −10 °C resulted only in silylation of the tertiary alcohol to give 2a in 97% yield (Table 1a, entry 1). However, performing the reaction with 1.5 equiv. of TMSOTf at 21 °C provided β-silyoxyketone 3a in 33% yield along with several other side products (entry 2). Although the yield of 3a was low, this result did confirm the feasibility of migrating the bicyclic fragment.
Table 1 Optimization of the reaction conditions
110 mol% of 2,6-(t-Bu)2-4-MePyr.
110 mol% of 2,6-(t-Bu)2-4-MePyr on 4.3 g scale.
|
|
Since migration occurred after silyl ether formation when excess TMSOTf was used, we posited that use of 2a as a substrate in conjunction with a stronger Lewis acid could allow the reaction to be conducted at low temperature, and could potentially improve the yield of 3a (Table 1b).9 When 2a was treated with 1.0 equiv. of TMSNTf2 at −78 °C for 45 minutes, starting material was consumed, but low yields of 3a were again observed (entry 1). Close monitoring of the reaction at early timepoints revealed that the reaction was very fast; indeed, stopping the reaction after 60 seconds improved the yield to 50% (entry 2). Further improvement was obtained by lowering the reaction temperature to −94 °C and quenching 10 seconds after addition of TMSNTf2 (entry 3). Although we were pleased with this discovery, it was not well suited for up-scaling.
Given that the use of silyl ether 2a negated the need for stoichiometric TMSNTf2, we evaluated using this Lewis acid catalytically. Gratifyingly, treatment of 2a with 10 mol% TMSNTf2 at −78 °C and then warming to 0 °C over 2.5 hours gave 3a in 99% yield (entry 4). The optimal conditions for gram scale were treatment of 2a with 10 mol% TMSNTf2 at −78 °C for 15 minutes without warming, which provided semi-pinacol product 3a in a 97% yield (entry 5). To our knowledge, TMSNTf2 has not previously been used as a catalyst for the semi-pinacol reaction.
With optimized conditions in hand, we examined the scope of the convergent fragment coupling strategy (Fig. 2). Although the yields of the alkenyl lithium 1,2-addition were substrate dependent, the semi-pinacol rearrangement gave consistently high yields. Simple linear alkenes, including an allylic silane and enol ether, migrated with high yields (3c, 3d). Cyclic and bicyclic alkenyl substrates also provided the rearrangement products (3e–3l) in excellent yields, showcasing the ability for this reaction to form attached-ring motifs bearing hindered quaternary centers. The fragment coupling tolerated substrates bearing TIPS- and PMB-protected alcohols (2g, 2i), functional groups that could be useful in natural product synthesis applications. Aromatic substrates were also found to be viable in this reaction, with products 3m–3o obtained in high yields. In addition to the gram scale reaction of 2a, the semi-pinacol rearrangement of aryl bromide 2n was carried out on gram scale, providing 3n in 73% yield. We were able to effect the migration of large polycyclic alkenes (3p–3q) in yields greater than 90%. Notably, these conditions are relatively mild and tolerated allyl ethers and enol ethers (3d, 3g, 3i), which are often reactive under common semi-pinacol rearrangement conditions.7c,10 A small additive screen found that the reaction tolerates acetal and ester functional groups, while an N-t-butyl-carboxylate-protected amine inhibited product formation (see ESI†).
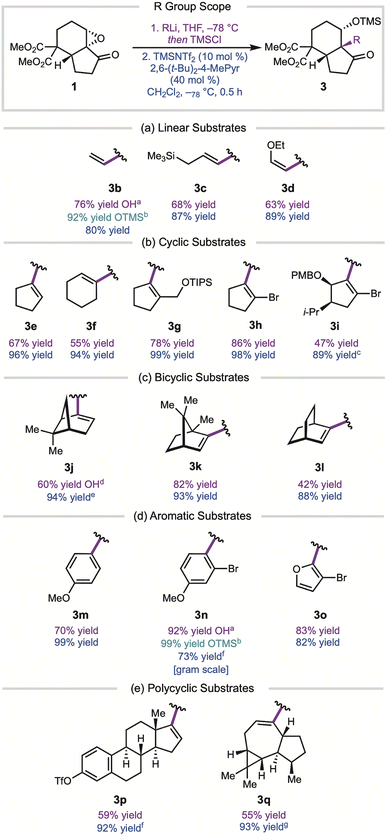 |
| Fig. 2 Scope of migrating group. Isolated yields of 1,2-addition product in purple, isolated yield of silylation (if separate step) in teal, isolated yields of semi-pinacol product in blue. aYield of 1,2-addition from Grignard reagent without TMSCl trapping. bYield of TMS protection. c50 mol% TMSNTf2, 110 mol% 2,6-(t-Bu)2-4-MePyr, −78 °C, 4 h. dYield of 1,2-addition from alkenyl lithium without TMSCl trapping. eTMSOTf (5 equiv), Et3N (6 equiv.), 0 °C to 21 °C, 1 h. f50 mol% TMSNTf2, 110 mol% 2,6-(t-Bu)2-4-MePyr, 0 °C, 0.5 h. g30 mol% TMSNTf2, 100 mol% 2,6-(t-Bu)2-4-MePyr, −78 °C, 4 h. | |
We also investigated simpler monocyclic ketones for their ability to rearrange using TMSNTf2 as the Lewis acid (Fig. 3). Using 10 mol% TMSNTf2, cyclopentanone 5a was prepared in 93% yield. Although the cyclohexyl substrate was less reactive, by increasing the catalyst loading to 50 mol%, the semi-pinacol product 5b was obtained in 79% yield.
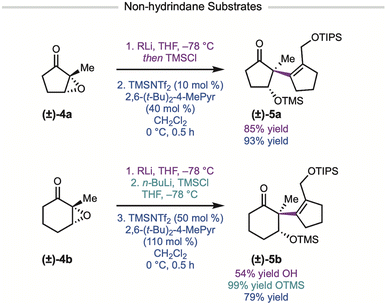 |
| Fig. 3 Substrates derived from simple epoxyketones. Isolated yields of 1,2-addition product in purple, isolated yield of silylation (if separate step) in teal, isolated yields of semi-pinacol product in blue. | |
Several of the products prepared by this method contained alkenyl or aryl bromide substituents (e.g.3h, 3i, 3n, and 3o), which can allow for further functionalization of the product. For example, treatment of ketone 3n with DavePhos-Pd-G3 in the presence of K3PO4 at 80 °C resulted in clean enolate arylation to give tetracycle 6 (Scheme 1).11 We anticipate that tetracycle 6 could serve as an intermediate for the synthesis of the denudatine-type diterpenoid alkaloids.12
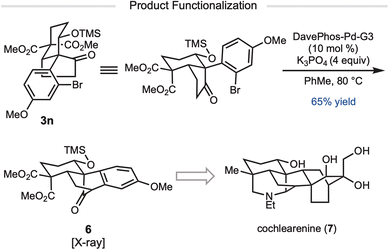 |
| Scheme 1 Intramolecular enolate arylation of 3n. | |
Conclusions
In summary, a convergent fragment coupling approach was developed to access a variety of quaternary centers in polycyclic substrates in high yields. This method is compatible with a variety of functional groups including sterically hindered alkenes, enol ethers, silyl ethers, alkenyl halides, aryl halides, and allyl silanes. The total syntheses of talatisamine and other C19 diterpenoid alkaloids have been enabled using this strategy to produce 3a in high yields on gram scale.8b
Data availability
Experimental procedures and characterization data (1H and 13C NMR) for all new compounds are provided in the ESI.†
Author contributions
Project conceptualization: A. R. W., V. W. M., and S. E. R. Funding acquisition: S. E. R. Experimental design and investigation: J. K. K., A. R. W., V. W. M., and S. E. R. Manuscript writing and editing: J. K. K. and S. E. R.
Conflicts of interest
There are no conflicts to declare.
Acknowledgements
The California Institute of Technology Center for Catalysis and Chemical Synthesis is gratefully acknowledged for access to analytical equipment. We thank the Dow Next Generation Educator Funds and Instrumentation Grants for their support of the NMR facility at Caltech and the X-ray Crystallography Facility in the Beckman Institute at Caltech. Dr Mona Shahgholi at the Multi-User Mass Spectrometry Laboratory of the Division of Chemistry and Chemical Engineering is acknowledged for their help in acquisition of HRMS data with instrumentation purchased by funds from DOW Next Generation Instrumentation and the NSF CRIF program (CCEC.DOWINSTR-1-GRANT.DOWINSTR). Dr M. Takase and L. Henling are acknowledged for acquiring the X-ray diffraction data. Funding: Fellowship support was provided by the Natural Sciences and Engineering Research Council (NSERC) of Canada (PGS-D fellowship to J. K. K., grant PGSD3-532535-2019), and the NSF (DGE-1144469 to A. R. W. and V. W. M). S. E. R. acknowledges financial support from the NIH (R35GM118191).
Notes and references
-
(a) L. Velluz, J. Valls and G. Nomine, Angew. Chem., Int. Ed., 1965, 4, 181–200 CrossRef;
(b) J. B. Hendrickson, J. Am. Chem. Soc., 1977, 99, 5439–5450 CrossRef CAS;
(c) S. H. Bertz, J. Am. Chem. Soc., 1982, 104, 5801–5803 CrossRef CAS.
- For reviews:
(a) D. Urabe, T. Asaba and M. Inoue, Chem. Rev., 2015, 115, 9207–9231 CrossRef CAS PubMed;
(b) N. Hill, K. Paruch and J. Švenda, Tetrahedron, 2016, 72, 3345–3368 CrossRef CAS;
(c) M. Tomanik, I. T. Hsu and S. B. Herzon, Angew. Chem., Int. Ed., 2021, 60, 1116–1150 CrossRef CAS PubMed.
- E. A. Peterson and L. E. Overman, Proc. Natl. Acad. Sci. U. S. A., 2004, 101, 11943–11948 CrossRef CAS PubMed.
-
(a) B. J. Huffman, T. Chu, Y. Hanaki, J. J. Wong, S. Chen, K. N. Houk and R. A. Shenvi, Angew. Chem., Int. Ed., 2022, 61, e202114514 CrossRef CAS PubMed;
(b)
E. J. Corey and X.-M. Cheng, in The Logic of Chemical Synthesis, Wiley, New York, 1995, p. 39 Search PubMed.
- For select methods on the semi-pinacol rearrangement see:
(a) R. D. Li, W. J. Xia, Y. Q. Tu, F. M. Zhang and L. Shi, Chem. Commun., 2003, 798–799 RSC;
(b) L. Sejin, K. Keunho and C. Jin, Synlett, 2008, 19, 2155–2157 Search PubMed;
(c) X.-D. Hu, C.-A. Fan, F.-M. Zhang and Y. Q. Tu, Angew. Chem., Int. Ed., 2004, 43, 1702–1705 CrossRef CAS PubMed;
(d) Y. Liu, Y.-L. S. Tse, F. Y. Kwong and Y.-Y. Yeung, ACS Catal., 2017, 7, 4435–4440 CrossRef CAS;
(e) X. Li, B. Wu, X. Z. Zhao, Y. X. Jia, Y. Q. Tu and D. R. Li, Synlett, 2003, 14, 623–626 Search PubMed;
(f) T. J. Snape, Org. Biomol. Chem., 2006, 4, 4144–4148 RSC;
(g) H. Wu, Q. Wang and J. Zhu, J. Am. Chem. Soc., 2019, 141, 11372–11377 CrossRef CAS PubMed;
(h) K. Suzuki, M. Miyazawa and G.-I. Tsuchihashi, Tetrahedron Lett., 1987, 28, 3515–3518 CrossRef CAS;
(i) C. M. Marson, A. Khan, R. A. Porter and A. J. A. Cobb, Tetrahedron Lett., 2002, 43, 6637–6640 CrossRef CAS;
(j) M. Liu, H. Huang and Y. Chen, Chin. J. Chem., 2018, 36, 1209–1212 CrossRef CAS;
(k) C. Chen, J.-C. Kang, C. Mao, J.-W. Dong, Y.-Y. Xie, T.-M. Ding, Y.-Q. Tu, Z.-M. Chen and S.-Y. Zhang, Green Chem., 2019, 21, 4014–4019 RSC;
(l) D. H. Lukamto and M. J. Gaunt, J. Am. Chem. Soc., 2017, 139, 9160–9163 CrossRef CAS PubMed;
(m) F. Romanov-Michailidis, M. Pupier, L. Guénée and A. Alexakis, Chem. Commun., 2014, 50, 13461–13464 RSC;
(n) K. Namba, M. Kanaki, H. Suto, M. Nishizawa and K. Tanino, Org. Lett., 2012, 14, 1222–1225 CrossRef CAS PubMed;
(o) B. M. Wang, Z. L. Song, C. A. Fan, Y. Q. Tu and Y. Shi, Org. Lett., 2002, 4, 363–366 CrossRef CAS PubMed;
(p) M. Shimazaki, H. Hara, K. Suzuki and G.-I. Tsuchihashi, Tetrahedron Lett., 1987, 28, 5891–5894 CrossRef CAS;
(q) S. Yao, K. Zhang, Q.-Q. Zhou, Y. Zhao, D.-Q. Shi and W.-J. Xiao, Chem. Commun., 2018, 54, 8096–8099 RSC;
(r) C.-A. Fan, X.-D. Hu, Y.-Q. Tu, B.-M. Wang and Z.-L. Song, Chem.–Eur. J., 2003, 9, 4301–4310 CrossRef CAS PubMed;
(s) C. J. Cheer and C. R. Johnson, J. Am. Chem. Soc., 1968, 90, 178–183 CrossRef CAS;
(t) Y. Q. Tu, L. D. Sun and P. Z. Wang, J. Org. Chem., 1999, 64, 629–633 CrossRef CAS;
(u) N. Hirama, R. Sakamoto and K. Maruoka, Asian. J. Org. Chem., 2019, 8, 1390–1393 CrossRef CAS;
(v) P. Gu, Y.-M. Zhao, Y. Q. Tu, Y. Ma and F. Zhang, Org. Lett., 2006, 8, 5271–5273 CrossRef CAS PubMed;
(w) Y. Q. Tu, C. A. Fan, S. K. Ren and A. S. C. Chan, J. Chem. Soc., Perkin Trans. 1, 2000, 3791–3794 RSC;
(x) M. Y. Yang, L. Wang, S.-Y. Zhang, Y.-Q. Tu and F.-M. Zhang, Org. Lett., 2012, 14, 5114–5117 CrossRef CAS PubMed.
- K. Tanino, K. Onuki, K. Asano, M. Miyashita, T. Nakamura, Y. Takahashi and I. Kuwajima, J. Am. Chem. Soc., 2003, 125, 1498–1500 CrossRef CAS PubMed.
- For reviews on the use of the semi-pinacol rearrangement in total syntheses see:
(a) T. J. Snape, Chem. Soc. Rev., 2007, 36, 1823–1842 RSC;
(b) B. Wang and Y.-Q. Tu, Acc. Chem. Res., 2011, 44, 1207–1222 CrossRef CAS PubMed;
(c) Z.-L. Song, C.-A. Fan and Y.-Q. Tu, Chem. Rev., 2011, 111, 7523–7556 CrossRef CAS PubMed;
(d) X.-M. Zhang, Y.-Q. Tu, F.-M. Zhang, Z.-H. Chen and S.-H. Wang, Chem. Soc. Rev., 2017, 46, 2272–2305 RSC;
(e) X.-M. Zhang, B.-S. Li, S.-H. Wang, K. Zhang, F.-M. Zhang and Y.-Q. Tu, Chem. Sci., 2021, 12, 9262–9274 RSC.
- Select examples of a convergent fragment coupling semi-pinacol based strategy in total synthesis.
(a) A. Cernijenko, R. Risgaard and P. S. Baran, J. Am. Chem. Soc., 2016, 138, 9425–9428 CrossRef CAS PubMed;
(b) A. R. Wong, N. J. Fastuca, V. W. Mak, J. K. Kerkovius, S. M. Stevenson and S. E. Reisman, ACS Cent. Sci., 2021, 7, 1311–1316 CrossRef CAS PubMed.
- B. Mathieu and L. Ghosez, Tetrahedron, 2002, 58, 8219–8226 CrossRef CAS.
- Evaluation of a limited number alkyl substituents as migrating groups failed to give the desired semi-products..
- M. J. Fox, X. Huang, A. Chieffi and S. L. Buchwald, J. Am. Chem. Soc., 2000, 122, 1360–1370 CrossRef.
-
(a) C. Dank, R. Sanichar, K.-L. Choo, M. Olsen and M. Lautens, Synthesis, 2019, 51, 3915–3946 CrossRef CAS;
(b) K. G. M. Kou, B. X. Li, J. C. Lee, G. M. Gallego, T. P. Lebold, A. G. DiPasquale and R. Sarpong, J. Am. Chem. Soc., 2016, 138, 10830–10833 CrossRef CAS PubMed.
Footnote |
† Electronic supplementary information (ESI) available: Experimental procedures, characterization data (1H and 13C NMR, HRMS, FTIR) for all new compounds (PDF). See DOI: https://doi.org/10.1039/d2sc07023e |
|
This journal is © The Royal Society of Chemistry 2023 |
Click here to see how this site uses Cookies. View our privacy policy here.