DOI:
10.1039/D2SC06435A
(Edge Article)
Chem. Sci., 2023,
14, 1434-1442
BOINPYs: facile synthesis and photothermal properties triggered by photoinduced nonadiabatic decay†
Received
22nd November 2022
, Accepted 7th January 2023
First published on 9th January 2023
Abstract
Photothermal agents (PTAs) represent a core component of photothermal therapy (PTT). However, the current photothermal dyes are almost derived from well-known chromophores such as porphyrins, cyanine, and BODIPYs, and the design of new chromophores as versatile building blocks for PTA is considerably challenging because of the complexity of the modulation of excited-states. Herein, we adopted the concept of photoinduced nonadiabatic decay (PIND) to develop a photothermal boron-containing indoline-3-one-pyridyl chromophore (viz. BOINPY) with a facile one-pot synthesis and high yields. BOINPY derivatives exhibited specific features that fully address the concerns related to the design of PTA. The behavior and mechanism of BOINPYs for generating heat through the conical intersection pathway, which is called PIND, have been well understood through theoretical calculations. After encapsulation into the F127 copolymer, BOINPY@F127 nanoparticles displayed efficient photothermal conversion and performed well in the treatment of solid tumors upon light irradiation with good biocompatibility. This study provides useful theoretical guidance and concrete photothermal chromophores, which offer a versatile strategy embedding tunable properties for the development of diverse high-performance PTA.
Introduction
Cancer is one of the most deadly diseases known to humans.1 Conventional cancer therapies including surgery, chemotherapy, and radiotherapy suffer from several limitations, such as non-specificity and various side effects.2 Recently, photothermal therapy (PTT) has received significant attention for cancer treatment owing to its low systemic toxicity, good specificity, and minimal invasiveness.3 Various photothermal agents (PTAs) based on inorganic materials and organic polymers have been prepared; although they exhibit excellent photostability and photothermal conversion, their potential for long-term toxicity and lack of biodegradability provide barriers to effective clinical efforts.4 Small organic molecules are ideally potential alternatives to nanomaterials because they can be conceived with considerations of function, safety, and formulation, and their features can be further fine-tuned through specialized synthesis.5 Currently, most of the organic photothermal compounds are derived from well-known chromophores such as porphyrins,6 phthalocyanines,7 diketopyrrolopyrroles,8 cyanines,9 and BODIPY,10 whose syntheses are usually time-consuming, involving multiple steps and therefore low yields. Therefore, a rational design of molecular platforms with scale-up synthesis and high yields that exhibit high photothermal therapeutic efficacy is essential.
Photothermal effects originate from nonradiative relaxation processes.5a Because photothermal agents convert the absorbed photons into heat, they generally have low fluorescence quantum yields and low yields of singlet oxygen generation. In addition, photothermal agents should ideally absorb in the red/NIR region and be highly photostable in this region.11 Theoretically, the excited molecular motions can release the excitation energy as heat through the conical intersection (CI) nonradiative pathway, which is called photoinduced nonadiabatic decay (PIND). Such excited molecules can easily go by the nonradiative CI pathway and return to the ground state, thus PIND-guided chromophores have great potential to serve as photothermal agents.12 Our inspiration for building the novel core skeleton of photothermal agents came from isoindoline-1-one-pyridyl difluoroboron dyes (BOISPY), reported by Xiao et al. as shown in Fig. 1a (left), which possess strong blue fluorescence.13 The low molecular orbital (MO) distribution of the phenyl ring at the isoindoline unit in the highest occupied MO (HOMO) results in an absorption wavelength in the UV region. We have previously explored the spectroscopic properties of the aza-isoindoline-1-one-pyridyl difluoroboron dye.14 Based on the previous study, herein we describe the development of a new chromophore (BOINPY) in which the isoindolinyl unit is replaced with an indolin-3-one unit through a one-step continuous reaction (Fig. 1a, right). Analysis of the results calculated indicate that this new core has a narrow bandgap relative to the reported BOISPY, which is beneficial for the red/NIR light absorbing capability (Fig. 1b, S1 and Table S1, ESI†). In addition, nucleus-independent chemical shift (NICS) calculations show that the five-membered ring of the new chromophore exhibits weak antiaromaticity, which is conducive to the delocalization of electrons over the entire core skeleton,18 as observed in the ACID plot (Fig. 1c and d).19 Moreover, the S1 → S0 transition corresponds to a large charge separation with a small oscillator strength (f = 0.08), also observed from the hole and electron distributions for the S1 state of BOINPY (Fig. 1e and S2, ESI†); the S1 state is spectroscopically “dark”, which inhibits the radiative processes and quenches the fluorescence. Structural CI was computed to better explain the different spectroscopic properties of the two isomers and to explore a theoretical strategy for the construction of photothermal reagents in depth.20 After photoexcitation, the excited state of BOISPY relaxes to the excited state minimum (S1min), and then releases the energy through radiative decay because the configurations of S1min and CI are quite different and the energy gap from the S1min (3.47 eV) to CI (5.22 eV) is relatively large (ca. 1.75 eV). In addition, there is a small barrier (0.30 eV higher than CI) along the pathway connecting S1min and CI, which was approximately obtained by the linearly interpolated internal coordinates (LIICs). The radiative decay thus plays a major role, and hence BOISPY exhibits strong fluorescence (Fig. 1f). However, for BOINPY, the gap between S1min (2.56 eV) and CI (3.43 eV) is only 0.87 eV, which is energetically accessible. Further LIIC calculations show that there is no barrier. The small energy difference is somewhat because the structures of the S1min and CI for BOINPY are almost identical (Fig. 1f and S2, ESI†). This indicates that the excited energy can easily cross through the nonradiative pathway and return to the ground state by heat. Such a chromophore core thus has significant potential to serve as the photothermal agent. This design idea based on PIND provides a significant strategy for the design of photothermal agents.
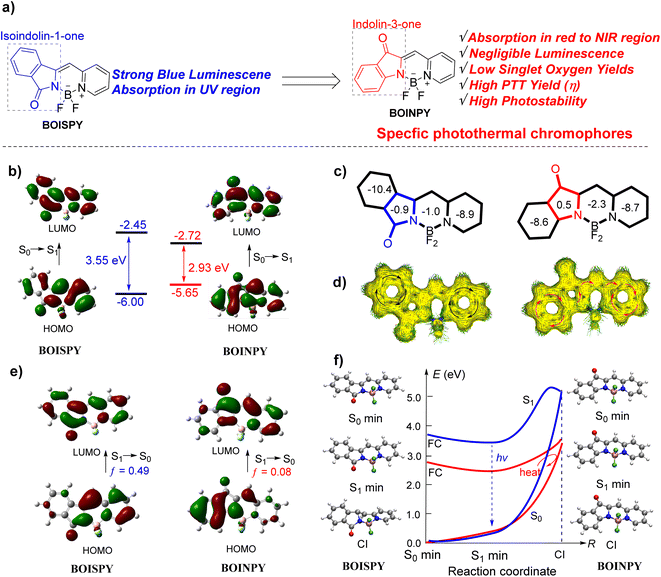 |
| Fig. 1 (a) Structures of boron-containing isoindoline-1-one-pyridyl dyes (BOISPY) and our designed boron-containing indolin-3-one-pyridyl (BOINPY) chromophore. (b) Frontier molecular orbital (MO) energetics of the ground-state. (c) NICS(1) value. (d) ACID plot. (e) Frontier MOs of the excited S1 state. The vertical excitation related calculations are based on the optimized ground-state geometry (S0 state), while the emission related calculations were based on the optimized excited state (S1 state) geometry at the B3LYP/6-31G(d,p) level.15 (f) The structures of the conical intersection (CI) were determined at the spin flip SF-BHandHLYP/6-31G(d,p) level using the GAMESS 2021 software (blue line for BOISPY and red line for BOINPY).16 All excitation energies were further calculated using the SF-BHandHLYP functional with the basis set 6-311G(d,p).17 | |
As theoretically predicted, the BOINPY core and its derivatives exhibited gratifying properties such as negligible emission, extremely low singlet oxygen production capability, and superior photostability; the above-mentioned features fully address the concerns related to the use of PTA in PTT. Compounds 2b and 2b encapsulated in F127 copolymer (2b@F127) exhibited high photothermal conversion efficiencies of 57% and 44%, respectively. In addition, 2b@F127 exhibited NIR PTT capability, robust photostability and excellent biocompatibility in vivo.
Results and discussion
Synthesis
BOINPY was synthesized from commercially available 1-acetyl-3-indolinone via a one-step continuous reaction (Scheme 1a). Treatment of 1-acetyl-3-indolinone with an aromatic aldehyde in the presence of triethylamine afforded the key precursor by a simple filtration, which was trapped with BF3·OEt2 in the presence of triethylamine or with BPh3 in refluxing toluene to obtain the target molecules 1a–3a in yields of 64–71% and 1b–3b in yields of 78–95%. This simple potent skeleton might prove useful to enrich the structural diversity of chromophores. With the above promising synthesizing results, dye 1c, carrying a bromide atom, was prepared according to the synthetic method of 1a. And then it was tolerated under the conditions of Suzuki–Miyaura coupling, Heck coupling, and Sonogashira coupling and afforded phenylated (4a), styrylated-(4b), and phenylethynylated (4c) BOINPYs (Scheme 1b). All these compounds were stable in air and moisture. The structures of all the BOINPY dyes were characterized by 1H NMR and 13C NMR spectroscopy and high-resolution MS.
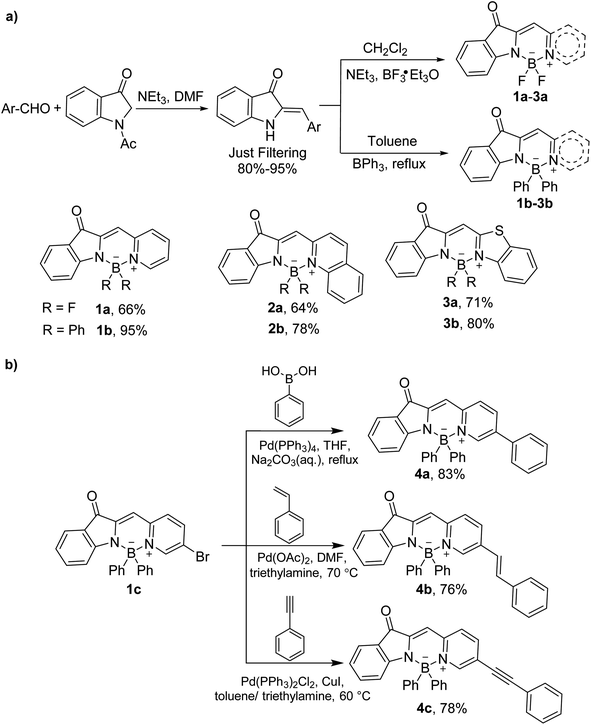 |
| Scheme 1 (a) Synthetic route to BOINPYs 1a–3a and 1b–3b. (b) Transition metal catalyzed coupling reaction of BOINPYs 4a–4c. | |
X-ray single-crystal structure analysis
Single crystals of 1a, 3a, and 2b suitable for X-ray diffraction were obtained by the slow diffusion of hexane into dichloromethane at room temperature. The C1–C2 and C3–C4 bond lengths in BOISPY are 1.469 and 1.479 Å, respectively, indicating a single-bond character. The single bond character suggests that the phenyl ring at the indolinyl unit is rarely involved in the π-conjugation system. This is consistent with theoretical calculations and spectral properties. In contrast, the C2–N1 bond length in BOINPY is 1.410–1.420 Å, which is more conducive to the delocalization of electrons in the indole and pyridine units, the red-shifted absorption maximum can be attributed to this delocalization. The boron atom of BOINPY is coordinated in a tetrahedral geometry by two nitrogen atoms and two fluorine atoms or carbon atoms of the phenyl group (Fig. 2). The B–N (pyridyl or thiazolyl nitrogen) bond lengths are larger than the B–N bond lengths (indoline nitrogen) because of the asymmetric structure.14a,21 The core was nearly planar, and partially overlapping head-to-head or head-to-tail π–π stacking interactions were observed (Fig. S3, ESI†).
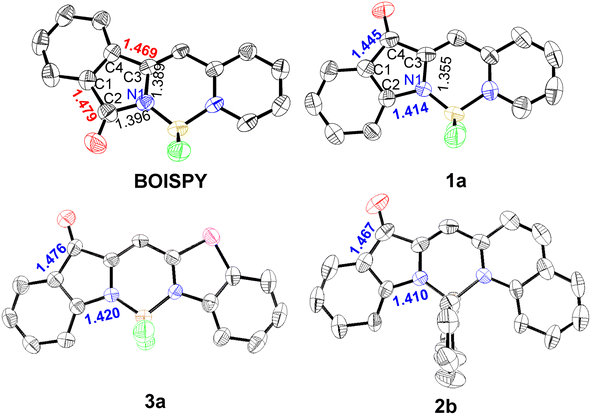 |
| Fig. 2 ORTEP views of the molecular structures of BOISPY, 1a, 3a, and 2b with thermal ellipsoids set at 50% probability. | |
Photophysical properties
The photophysical properties of the prepared dyes were investigated in different solvents and films, and the results are summarized in Tables 1 and S2.† The absorbance maxima of BOINPYs (1b–3b and 4a–4c) were observed in the wavelength ranges of 518–644 nm and 527–720 nm in dichloromethane and film, respectively (Fig. 3aand b). Usually, replacing F with an aromatic group at the boron of BODIPY did not affect the absorption wavelength; however, a large redshift (75–94 nm) of the absorption maximum was observed for BOINPY, because the electron-donating phenyl group participated in the frontier MO and decreased the band gap. The absorption bands on the film were broader, with a redshift of the absorbance maxima to 720 nm (for 2b) owing to π–π stacking. The absorption maxima did not show any clear trend as a function of the solvent polarity (Fig. S4, ESI†). Notably, unlike BOISPY, these molecules have high molar extinction coefficients of absorption (εmax = 1.69–7.22 × 104 M−1 cm−1) and negligible fluorescence quantum yields (<1%) (Tables 1, S2 and Fig. S4, S5, ESI†), indicating highly efficient nonradiative processes in the excited states originating from the nonradiative CI pathway. The photostability was assessed, and the absorption did not decrease beyond 3% in any case after 30 min of irradiation (Fig. 3cand d). However, the reference dyes rhodamine 6G and MB were quickly decomposed. The yields of singlet oxygen generation were significantly low (<8%) (Fig. S6 and S7, ESI†). Therefore, the BOINPY system is sufficiently stable and suitable for PTT.
Table 1 Photophysical and photosensitising properties of compounds 1a–4c
Compounds |
λ
abs/nm |
λ
em/nm |
Φ
F
|
Φ
Δ
|
DCM |
Film |
2-MeTHF |
2-MeTHF |
Absolute fluorescence quantum yield (ΦF) upon irradiation at the corresponding absorption maxima.
Singlet oxygen quantum yield (ΦΔ) is determined using Rose Bengal (RB) for 1a–3a (ΦΔ(RB) = 0.75 in methanol)22 and MB for 1b–3b and 4a–4c (ΦΔ(MB) = 0.57 in DCM).23
n.d. = not detected, DCM = dichloromethane.
|
1a
|
518 |
527 |
591 |
0.006 |
n.d.c |
2a
|
552 |
567 |
621 |
0.005 |
n.d. |
3a
|
534 |
576 |
606 |
0.003 |
n.d. |
1b
|
593 |
644 |
660 |
0.004 |
1.5% |
2b
|
644 |
720 |
711 |
0.002 |
2.2% |
3b
|
618 |
672 |
690 |
0.003 |
n.d. |
4a
|
608 |
645 |
671 |
0.005 |
8% |
4b
|
625 |
660 |
680 |
0.006 |
n.d. |
4c
|
621 |
639 |
685 |
0.004 |
n.d. |
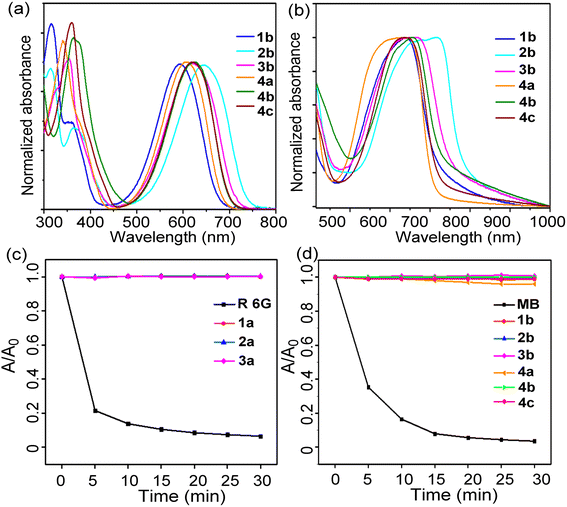 |
| Fig. 3 Normalized absorption spectra of 1b–3b and 4a–4c in dichloromethane (a) and in drop-cast film on a quartz plate (b) at 293 K. (c) and (d) Photostability in dichloromethane upon irradiation with a laser beam (560 nm for 1a–3a, rhodamine 6G as the reference compound; 635 nm for 1b–3b and 4a–4c, methylene blue as the reference compound) for 30 min (dye concentration = 5.0 μM, 298 K). | |
Photothermal studies in solution and in vitro
2b and 4a–4c were selected for the investigation of photothermal performance. The temperature of 2b in DMF increased from 25 to 45 °C within 15 min upon irradiation with a 650 nm laser of intensity 0.8 W cm−2 (Fig. S8a and b, ESI†). No significant change in temperature (<3 °C) was detected in the control of DMF. The temperature increase is more prominent at higher laser powers (Fig. S8c, ESI†). The concentration and laser power dependence of 2b indicated that the heat generation could be controlled as desired. Furthermore, the heating and cooling curves were examined to determine the photothermal conversion efficiency (Fig. S8d, ESI†). Based on the corresponding thermal time constant, the photothermal conversion efficiency (η) of 2b was determined to be 56.9% (Fig. S8e, ESI†). Notably, even after four on-and-off cycles with 650 nm laser irradiation, the maximum temperature of 2b did not decrease significantly (Fig. S8f, ESI†), demonstrating the outstanding photothermal stability of 2b. Thus, 2b could efficiently convert light into heat and serve as a good contrast agent for PTT. The photothermal conversion efficiencies of 4a–4c were calculated to be 55.3%, 50.1%, and 52.7%, respectively (Fig. S9, ESI†), indicating that structural modification based on this chromophore core is an intriguing strategy to design high-performance photothermal agents.
Encouraged by the good NIR absorption capacity and theoretical computation, 2b was selected for the in vitro and in vivo photo-treatment investigation. To evaluate the therapeutic efficacy of the 2b-based photothermal agent in living systems, we fabricated the nanomedicine via simple encapsulation with Pluronic F127 as an encapsulation matrix to improve the biocompatibility of 2b. As shown in Fig. 4a, the spherical morphology of 2b@F127 could be observed in the transmission electron microscopy (TEM) image while a hydrodynamic size of about 81.8 nm was tested by dynamic light scattering (DLS), which contributed to their accumulation at the tumour parts. Meanwhile, the UV-vis absorbance spectra also confirmed the successful encapsulation of 2b in Pluronic F127. The absorption maximum of 2b@F127 was slightly redshifted relative to that of 2b in DMF due to aggregation (Fig. 4b). The absorption maximum at 650 nm is in good agreement with that of commercial lasers. 2b@F127 has excellent water solubility and structural stability, with almost no change observed for hydrodynamic diameter while stored in the refrigerator at 4 °C for one week in the normal physiological environment (Fig. 4c). We then explored whether 2b@F127 was an effective photothermal agent in aqueous media. The temperature changes at different concentrations of 2b@F127 upon laser irradiation were recorded (Fig. 4d). The temperature reaches up to 35 °C at concentrations of 100 μmol L−1 upon irradiation for 900 s at an intensity of 0.5 W cm−2. As shown in Fig. 4e, the photothermal conversion efficiency (η) of 2b@F127 was 44.2%, based on the photothermal effects and the time constant. This η value is higher than those of most photothermal agents24 like Cu9S5 nanoparticles (ca. 25.7%) and indocyanine green (ICG) (ca. 12.1%). After irradiation for 5 min with a 650 nm laser (0.5 W cm−2), the temperature of the 2b@F127 solution increased from 23 to 40 °C and a small change was observed for the PBS-only sample (Fig. 4f). In addition, the biocompatibilities of 2b@F127 were investigated by the hemocompatibility assays using a healthy mouse's red blood cells (RBCs). There was no observable hemolysis occurrence in either PBS or 2b@F127 solution under the studied concentration range and time (Fig. 4g).
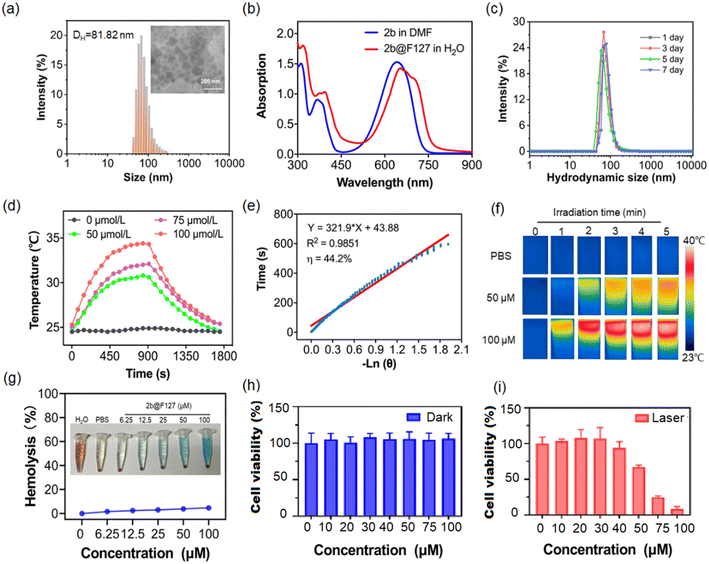 |
| Fig. 4 (a) Hydrodynamic diameter distribution and TEM images (inset) obtained for 2b@F127. (b) The absorption spectra of 2b in DMF and 2b@F127 in water. (c) Dynamic light scattering (DLS) curves of PBS of 2b@F127 on different days of a week. (d) The photothermal effect of 2b@F127 with different concentrations after irradiation by 650 nm laser (0.5 W cm−2) for 30 min. (e) Cooling time of 2b@F127 vs. negative log of temperatures and the linear fitting curve. (f) The photothermal photographs of 2b@F127 (λex = 650 nm, 0.5 W cm−2) using an FLIR E53 thermal imaging camera. (g) Hemolysis assay of 2b@F127 against mice red blood cells (RBCs). (h) and (i) Cell viability of HeLa cells incubated with different concentrations of 2b@F127 for 24 h without and with 650 nm (0.5 W cm−2) laser irradiation. | |
Having verified the photochemical and photophysical properties of 2b@F127 in solution, we next investigated the photothermal potential at cellular levels with HeLa cancer cells. As displayed in Fig. 4h, the low dark cytotoxicity of 2b@F127, even at a high concentration up to 100 μM, was found by co-incubation with HeLa cells using the standard Cell Counting Kit 8 (CCK-8). 2b@F127 exerted significant concentration-dependent cytotoxicity towards cells, and the cell viability was inhibited down to 8% at a dose of 100 μM when exposed to a 650 nm laser (Fig. 4i). Then, intracellular generation of reactive oxygen species (ROS) by 2b@F127 was investigated using 2′,7′-dichlorofluorescein diacetate (DCFH-DA) as a probe, Fig. S10† shows that 2b@F127 can generate intracellular ROS upon NIR light irradiation. We next investigated the 2b@F127-mediated therapeutic efficacy in cells. This was also intuitively demonstrated by live-dead cell staining using confocal laser scanning microscopy (CLSM), by co-staining with calcein AM (live, green fluorescence), and PI (dead, red fluorescence). As depicted in Fig. S10e,† the 2b@F127 + laser group treated cells showed the highest cell-killing effect. Additionally, we determined the role of 2b@F127 for phototherapy to induce DNA damage on clone formation (Fig. S10f†). A significantly decreased number of colonies was found in 2b@F127, compared with the control groups. These results confirmed that the photothermal effect of 2b@F127 could effectively kill tumor cells.
In vivo animal experiments
Encouraged by the excellent photothermal properties of 2b@F127 in vitro, photothermal therapy was performed in vivo. Tumour-bearing nude mice were randomly divided into two groups, named “PBS” and “2b@F127”. The HeLa-tumour-bearing mice were treated with intra-tumour injections of PBS or 2b@F127 (200 μM), following which both the groups were exposed to a 650 nm laser for 0–10 min. The thermal images were continuously obtained with an IR thermal camera to monitor the temperature change at the tumour site upon laser irradiation. The thermal signals reached their maximum after 6 min. As shown in Fig. 5aand b, upon laser irradiation, the mice treated with 2b@F127 showed an approximate increase of 26 °C within 10 min, which was reasonably higher than that observed for the mice treated with PBS only. Thus, 2b@F127 underwent effective photothermal conversion in vivo.
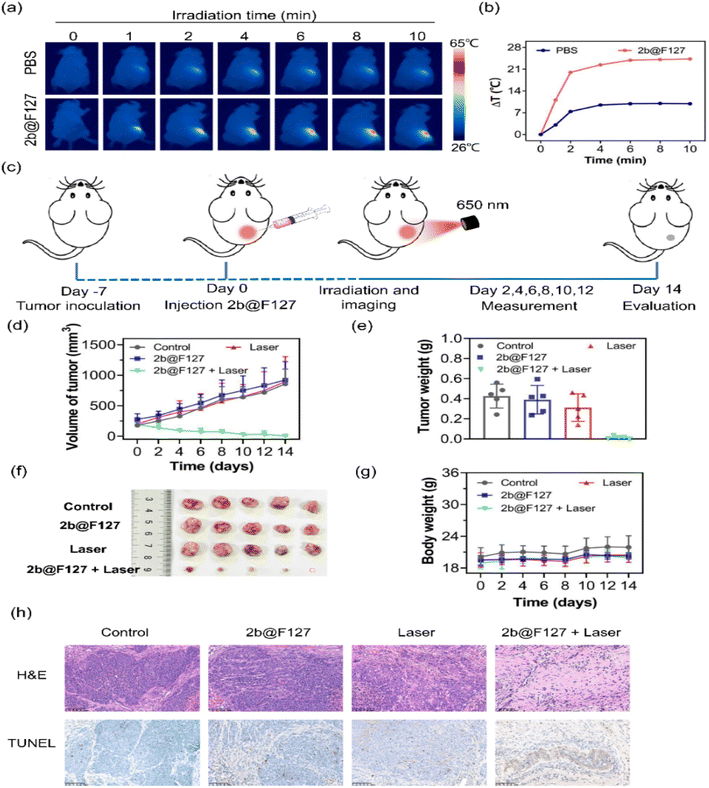 |
| Fig. 5 (a) Thermal imaging of HeLa-tumour-bearing mice subjected to laser irradiation for 10 min after intra-tumour injection of PBS or 2b@F127 (200 μM, λex = 650 nm, 0.5 W cm−2). (b) Temperature variation in the tumour region within 10 min. (c) Schematic diagram of tumor-bearing mice model establishment and treatment operation. The mice in the treated groups were injected with 2b@F127 and the tumor sites were irradiated with a 650 nm laser (0.5 W cm−2, 10 min) after the injection. (d) Tumor volumes of the mice in the different groups during the observation period (n = 5). (e) Average weights of tumors collected from mice 14 days after initiation of various treatments. (f) Digital photos of excised tumors from the mice after 14 days under different treatment conditions as indicated. (g) Body weights of the mice in different groups during the observation period. (h) Tumor tissues of each group after photothermal therapy were stained by hematoxylin and eosin (H&E), and TUNEL (scale bar: 100 μm). | |
Subsequently, in order to investigate the therapeutic efficacy of 2b@F127 towards solid tumours, we further implemented an antitumor study on nude mice bearing HeLa tumours (Fig. 5c). These tumour-bearing mice were randomly divided into four groups (PBS, 2b@F127, laser, and 2b@F127 + laser). All mice were intratumor injected with 2b@F127 or control samples (PBS), and then subjected to laser exposure (650 nm, 0.5 W cm−2 for 10 min). During the 14 days treatment period, the tumor volumes and body weights were measured every 2 days. As shown in Fig. 5d–f, it was found that the control, 2b@F127, and laser groups exhibited negligible therapeutic effects on tumor growth. In the 2b@F127 + laser group, the tumor growth was obviously inhibited and the size was smaller and even disappeared compared to the other groups, showing that 2b@F127 exhibited exceptional anti-tumor efficacy under laser irradiation. Additionally, the body weight of mice in all four groups showed a slightly increasing trend throughout the 14 days tumor treatment period (Fig. 5g), demonstrating that 2b@F127 had low biotoxicity and high biocompatibility. These results clearly demonstrated that high performance in the cure of solid tumors could be achieved by the 2b@F127-enabled phototherapy under laser irradiation. To further investigate the PTT effect and biosecurity of 2b@F127, hematoxylin & eosin (H&E) staining and a terminal deoxynucleotidyl transferase-mediated dUTP nick end labeling (TUNEL) assay of tumor sections were performed. H&E-staining of the tumor slices indicated that the 2b@F127 + laser-treated group achieved the best treatment efficacy, while no obvious apoptotic/necrotic tissues were observed in the other groups. Moreover, the TUNEL assay results displayed a significant increase in the amount of apoptotic chromatin in the tumors treated with 2b@F127 under laser irradiation. In addition, the in vivo systemic toxicity to main organs was also evaluated by monitoring H&E staining. 2b@F127 showed no significant damage to normal tissues, including the heart, liver, spleen, lung, and kidney of the mice (Fig. S11, ESI†). Overall, these results validated that 2b@F127 possesses a potential application for PTT.
Conclusions
In summary, we have developed a class of BOINPY derivatives with modular synthesis and high yields. They exhibited negligible emission, extremely low singlet oxygen production capability, superior photostability, and high photothermal conversion efficiencies. These results demonstrated that the BOINPY core provides a versatile construction kit for the design of PTA. As illustrated here for a series of BOINPY derivatives, the molecular platform enables structural modification with diversity and meanwhile retains the photothermal properties. Moreover, we have shown that a high-efficiency photothermal agent can be rationally designed through a PIND process under NIR-laser irradiation. In vitro and in vivo investigations revealed that chromophore@F127 can serve as an efficient photothermal agent for tumour theranostics with minimized side effects for other tissues. Theoretically, PIND-guided photothermal molecules should have quantitative photothermal efficiency. For BOINPY, the energy of the CI state is higher than that of the S0min and S1min states, it needs to consume a certain amount of energy to cross the energy barrier. The design of photothermal molecules, in which the energy of the CI state is lower than those of the S0min and S1min states, is now ongoing. This work provides a novel photothermal molecular platform and a deeper understanding for exploring organic NIR-absorbing PTA for practical applications.
Ethical statement
All the animal experiments were performed in strict accordance with the National Institutes of Health Guide for the Care and Use of Laboratory Animals and were approved by the Institutional Animal Care and Use Committee of the Peking University First Hospital (Beijing, China).
Data availability
The ESI† includes all experimental details, including TD-DFT calculations, spectroscopic measurements, X-ray analysis, synthesis and characterization of all products reported in this study. NMR spectra of all products are included as well.
Author contributions
H. L., J. Z. and Z. G., supervised the work. L. G., X. S., S. W. and R. Z. conducted the experiments. H. L. and Z. N. performed the theoretical calculations. H. L., L. G. and J. Z. wrote the manuscript. All authors have been involved in checking the data, and have approved the final version of the manuscript.
Conflicts of interest
There are no conflicts to declare.
Acknowledgements
Financial support was provided by the National Natural Science Foundation of China (Grants No. 21871072, 21801057, 21571007, 21621061, 21778002, and 21861162008), the Chemistry and Chemical Engineering Guangdong Laboratory (1932002) and theoretical calculations were carried out at the Computational Centre for Molecular Design of Organosilicon Compounds, Hangzhou Normal University.
Notes and references
- R. L. Siegel, K. D. Miller and A. Jemal, Ca-Cancer J. Clin., 2020, 70, 7–30 CrossRef PubMed.
- K. Mortezaee, A. Narmani, M. Salehi, H. Bagheri, B. Farhood, H. Haghi-Aminjan and M. Najafi, Life Sci., 2021, 269, 119020 CrossRef CAS PubMed.
-
(a) Y. Liu, P. Bhattarai, Z. Dai and X. Chen, Chem. Soc. Rev., 2019, 48, 2053–2108 RSC;
(b) W. Fan, B. Yung, P. Huang and X. Chen, Chem. Rev., 2017, 117, 13566–13638 CrossRef CAS PubMed;
(c) L. Cheng, C. Wang, L. Feng, K. Yang and Z. Liu, Chem. Rev., 2014, 114, 10869–10939 CrossRef CAS PubMed;
(d) J. Huo, Q. Jia, H. Huang, J. Zhang, P. Li, X. Dong and W. Huang, Chem. Soc. Rev., 2021, 50, 8762–8789 RSC;
(e) Q. Zheng, X. Liu, Y. Zheng, K. W. K. Yeung, Z. Cui, Y. Liang, Z. Li, S. Zhu, X. Wang and S. Wu, Chem. Soc. Rev., 2021, 50, 5086–5125 RSC.
- N. Ni, X. Zhang, Y. Ma, J. Yuan, D. Wang, G. Ma, J. Dong and X. Sun, Coord. Chem. Rev., 2022, 458, 214415 CrossRef CAS.
-
(a) G. Feng, G.-Q. Zhang and D. Ding, Chem. Soc. Rev., 2020, 49, 8179–8234 RSC;
(b) H. S. Jung, P. Verwilst, A. Sharma, J. Shin, J. L. Sessler and J. S. Kim, Chem. Soc. Rev., 2018, 47, 2280–2297 RSC;
(c) G. Xu, W. Guo, X. Gu, Z. Wang, R. Wang, T. Zhu, H. Tian and C. Zhao, CCS Chem., 2020, 2, 527–538 CrossRef CAS.
-
(a)
T. W. Liu, E. Huynh, T. D. MacDonald and G. Zheng, in Cancer Theranostics, Elsevier, 2014, pp. 229–254 Search PubMed;
(b) Z.-S. Yang, Y. Yao, A. C. Sedgwick, C. Li, Y. Xia, Y. Wang, L. Kang, H. Su, B.-W. Wang and S. Gao, Chem. Sci., 2020, 11, 8204–8213 RSC.
- B.-D. Zheng, Q.-X. He, X. Li, J. Yoon and J.-D. Huang, Coord. Chem. Rev., 2021, 426, 213548 CrossRef CAS.
-
(a) Y. Cai, W. Si, Q. Tang, P. Liang, C. Zhang, P. Chen, Q. Zhang, W. Huang and X. Dong, Nano Res., 2017, 10, 794–801 CrossRef CAS;
(b) X. Jiang, L. Wang, H. Tang, D. Cao and W. Chen, Dyes Pigm., 2020, 181, 108599 CrossRef CAS.
- K. Bilici, S. Cetin, E. Aydındogan, H. Yagci Acar and S. Kolemen, Front. Chem., 2021 DOI:10.3389/fchem.2021.707876.
-
(a) M. Zhao, Q. Zeng, X. Li, D. Xing and T. Zhang, Nano Res., 2022, 15, 716–727 CrossRef CAS;
(b) C. Ma, T. Zhang and Z. Xie, J. Mater. Chem. B, 2021, 9, 7318–7327 RSC;
(c) D. Chen, Z. Zhong, Q. Ma, J. Shao, W. Huang and X. Dong, ACS Appl. Mater. Interfaces, 2020, 12, 26914–26925 CrossRef CAS PubMed.
- C. Xu and K. Pu, Chem. Soc. Rev., 2021, 50, 1111–1137 RSC.
- J. S. Ni, X. Zhang, G. Yang, T. Kang, X. Lin, M. Zha, Y. Li, L. Wang and K. Li, Angew. Chem., 2020, 132, 11394–11398 CrossRef.
- Y. Zhou, Y. Xiao, D. Li, M. Fu and X. Qian, J. Org. Chem., 2008, 73, 1571–1574 CrossRef CAS PubMed.
-
(a) H. Liu, H. Lu, J. Xu, Z. Liu, Z. Li, J. Mack and Z. Shen, Chem. Commun., 2014, 50, 1074–1076 RSC;
(b) H. Liu, H. Lu, Z. Zhou, S. Shimizu, Z. Li, N. Kobayashi and Z. Shen, Chem. Commun., 2015, 51, 1713–1716 RSC;
(c) Y. Wu, H. Lu, S. Wang, Z. Li and Z. Shen, J. Mater. Chem. C, 2015, 3, 12281–12289 RSC;
(d) H. Liu, Y. Wu, Z. Li and H. Lu, J. Porphyrins Phthalocyanines, 2014, 18, 679–685 CrossRef CAS;
(e) H. Zhang, Y. Wu, M. Fan, X. Xiao, J. Mack, G. Kubheka, T. Nyokong and H. Lu, New J. Chem., 2017, 41, 5802–5807 RSC;
(f) H. Liu, H. Lu, F. Wu, Z. Li, N. Kobayashi and Z. Shen, Org. Biomol. Chem., 2014, 12, 8223–8229 RSC.
-
M. J. Frisch, G. W. Trucks, H. B. Schlegel, G. E. Scuseria, M. A. Robb, J. R. Cheeseman, G. Scalmani, V. Barone, G. A. Petersson, H. Nakatsuji, X. Li, M. Caricato, A. V. Marenich, J. Bloino, B. G. Janesko, R. Gomperts, B. Mennucci, H. P. Hratchian, J. V. Ortiz, A. F. Izmaylov, J. L. Sonnenberg, D. Williams-Young, F. Ding, F. Lipparini, F. Egidi, J. Goings, B. Peng, A. Petrone, T. Henderson, D. Ranasinghe, V. G. Zakrzewski, J. Gao, N. Rega, G. Zheng, W. Liang, M. Hada, M. Ehara, K. Toyota, R. Fukuda, J. Hasegawa, M. Ishida, T. Nakajima, Y. Honda, O. Kitao, H. Nakai, T. Vreven, K. Throssell, J. A. Montgomery Jr, J. E. Peralta, F. Ogliaro, M. J. Bearpark, J. J. Heyd, E. N. Brothers, K. N. Kudin, V. N. Staroverov, T. A. Keith, R. Kobayashi, J. Normand, K. Raghavachari, A. P. Rendell, J. C. Burant, S. S. Iyengar, J. Tomasi, M. Cossi, J. M. Millam, M. Klene, C. Adamo, R. Cammi, J. W. Ochterski, R. L. Martin, K. Morokuma, O. Farkas, J. B. Foresman and D. J. G. R. C. Fox, Gaussian 16 (Revision A.03), Gaussian Inc., Wallingford, CT, 2016 Search PubMed.
- M. W. Schmidt, K. K. Baldridge, J. A. Boatz, S. T. Elbert, M. S. Gordon, J. H. Jensen, S. Koseki, N. Matsunaga, K. A. Nguyen and S. Su, J. Comput. Chem., 1993, 14, 1347–1363 CrossRef CAS.
- S. R. Billeter and A. Curioni, J. Chem. Phys., 2005, 122, 034105 CrossRef PubMed.
- Z. Chen, C. S. Wannere, C. Corminboeuf, R. Puchta and P. v. R. Schleyer, Chem. Rev., 2005, 105, 3842–3888 CrossRef CAS PubMed.
- D. Geuenich, K. Hess, F. Köhler and R. Herges, Chem. Rev., 2005, 105, 3758–3772 CrossRef CAS PubMed.
-
(a) J.-S. Ni, X. Zhang, G. Yang, T. Kang, X. Lin, M. Zha, Y. Li, L. Wang and K. Li, Angew. Chem., Int. Ed., 2020, 59, 11298–11302 CrossRef CAS PubMed;
(b) N. Inai, D. Yokogawa and T. Yanai, J. Phys. Chem. A, 2021, 125, 559–569 CrossRef CAS PubMed.
- Y. P. Wu, S. S. Wang, Z. F. Li, Z. Shen and H. Lu, J. Mater. Chem. C, 2016, 4, 4668–4674 RSC.
- J. R. Wagner, H. Ali, R. Langlois, N. Brasseur and J. E. van Ller, Photochem. Photobiol., 1987, 45, 587–594 CrossRef CAS PubMed.
- F. Wilkinson, W. P. Helman and A. B. Ross, J. Phys. Chem. Ref. Data, 1993, 22, 113–262 CrossRef CAS.
-
(a) Y. Zou, M. Li, T. Xiong, X. Zhao, J. Du, J. Fan and X. Peng, Small, 2020, 16, 1907677 CrossRef CAS PubMed;
(b) W. Zhang, W. Lin, C. Li, S. Liu, X. Hu and Z. Xie, ACS Appl. Mater. Interfaces, 2019, 11, 32720–32728 CrossRef CAS PubMed;
(c) W. Sang, Z. Zhang, Y. Dai and X. Chen, Chem. Soc. Rev., 2019, 48, 3771–3810 RSC.
Footnotes |
† Electronic supplementary information (ESI) available: Experimental and additional spectral data. CCDC 2142887, 2142888 and 2142890. For ESI and crystallographic data in CIF or other electronic format see DOI: https://doi.org/10.1039/d2sc06435a |
‡ These authors contributed equally. |
|
This journal is © The Royal Society of Chemistry 2023 |
Click here to see how this site uses Cookies. View our privacy policy here.