DOI:
10.1039/D2RP00153E
(Review Article)
Chem. Educ. Res. Pract., 2023,
24, 394-406
Teaching crystal structures in undergraduate courses: a systematic review from a disciplinary literacy perspective
Received
27th May 2022
, Accepted 27th December 2022
First published on 3rd January 2023
Abstract
Teaching engineering students how to effectively communicate using the language, representations, and tools specific to their discipline is important to their development as practitioners in their respective fields. This article describes a literature review of learning activities intended to teach crystal structure and crystallography and explores the extent to which educators implement elements of disciplinary literacy. We categorized the purpose of the paper, what the students are asked to do, the representations used in the activities, and the learner roles that the activities support. We observe that a majority of the papers engage students in disciplinary practices and support many, but not all aspects of disciplinary literacy. Instructors use a variety of representations in presenting concepts and ask students to interact with the learning activities to recount information or interpret results. However, we find that the activities rarely ask students to synthesize new information or evaluate their information in any wider context. We suggest that instructors should aim to implement the entire range of learning roles, and employ more critical socio-cultural approaches when designing learning activities to make the sciences accessible to a more diverse population of learners.
Introduction
Individual disciplines encompass vast amounts of knowledge and approaches towards acquiring and creating knowledge. The members of a discipline range from experts to novices, all joined by a common understanding of what the discipline is about and what it does. As such, disciplines can be recognized as individual cultures whose members uphold certain types of social and cognitive practices (Moje, 2015). These practices involve being literate in how and when to use the specialized vocabulary, representations, and tools specific to the discipline (Airey and Linder, 2009), and to understand why these particular resources are valued and important (Ahn, 2019). Teaching within any discipline should include consideration of what knowledge and practices are valued and the application of appropriate conventions to support learners' disciplinary literacy.
The term disciplinary literacy is used to describe how each discipline utilizes varying perspectives and approaches when generating, communicating, evaluating, and renovating knowledge (Fang, 2012). Airey and Linder (2009) discuss that this knowledge is not inherently known by novice learners when they enter a discipline, but that they develop their disciplinary literacy as they interact with tools, activities, and representations of the discipline. Because they are often working scientists themself, instructors at the undergraduate level may forget that knowledge is formed over time through immersion within the discipline and focus instead on imparting direct knowledge without the deeper context (Airey and Linder, 2009). Moje (2015) asserts that supporting learners' disciplinary literacy includes the affective, social, and cultural dimensions of learning, thus creating opportunities for learners to regard their backgrounds, cultures, abilities, and developing knowledge as essential elements of their knowledge creation. Applying a disciplinary literacy perspective to how teaching and learning happens within a discipline aids in our understanding of how we are developing learners' ability to effectively engage in disciplinary practices.
Instructors commonly design learning activities to introduce students to the ways of knowing, thinking, believing, acting, and communicating within a specific discipline (Moje and Lewis, 2007; Ahn, 2019). The activities they choose are intended to acquaint students with discipline-specific resources—that is, vocabulary, concepts, tools, practices—that are deemed relevant and important by those who are identified as experts. More specific to undergraduate science education, how instructors use these resources creates a shared way of knowing (Airey and Linder, 2009) that supports students’ ability to reason and behave as a scientist (Lemke, 1998). These abilities include a range of skills and competencies that Moje calls the disciplinary cycle, including working with information and data, being able to represent information in several ways (for example visually, symbolically, or as text), analyzing, summarizing, and synthesizing findings, examining, and evaluating claims, and communicating those claims (Moje, 2015). More generally, socio-cultural pedagogy is united by three broad tenets: learning is socially based, teaching consists of assisting the learner, and performance is situative (Teemant et al., 2005). Such a socio-cultural approach tends to be more meaningful and equitable allowing students to become literate in the social and cognitive practices of experts within the discipline. For example, Finkenstaedt-Quinn et al. (2017) takes a socio-cultural approach to materials science education. In that approach, students complete writing assignments that engage the disciplinary cycle as they communicate STEM topics to a hypothetical non-STEM audience. In addition, students critique their peers’ drafts. This method deliberately focuses on student-generated communication to enhance learning, and has been applied to chemistry and across STEM (Reynolds et al., 2012; Ortiz Cáceres and Candela Rodriguez, 2022) and discussed in Rivard (1994). Other socio-cultural strategies for disciplinary literacy education include the use of multiple representation types (Moore et al., 2018) and designing learning activities that engage various learner roles (Serafini, 2012). Observing how the literature implicitly employs these strategies helps us to see how the disciplinary practitioners intend to make students literate in the overall discipline.
In this article, we conduct a systematic review of the literature that describes learning activities pertaining to crystal structure and crystallography. We do this to explore the various ways of knowing that are valued by the community, as seen in the goals and content of learning activities, and the degree to which disciplinary literacy approaches are currently used in the teaching of that subject. In particular, we apply the theories of socio-cultural pedagogy as synthesized into the 4 E's Heuristic (Moje, 2015) to guide our understanding of the emergent themes we observe. Our comprehensive review of the literature seeks to answer these two research questions:
RQ1. What are the goals of the learning activities used in the publications describing teaching concepts of crystals structure and crystallography?
A. What are the thematic categories for the explicitly stated purpose of the publications?
B. What are the types of learning activities that the students were asked to do?
C. What are the observed relationships between the themes and learning activities?
RQ2. Which disciplinary literacy tactics that are known to enhance disciplinary literacy are explicitly used in the learning activities described in these publications?
A. What are the observed relationships between the utilized forms of representation and learning activities?
B. What are the observed relationships between the supported learner roles when transacting with a learning activity?
Answering these research questions allows us to understand what is valued in the teaching of crystal structures and crystallography, to determine whether instructors are already using the principles of disciplinary literacy in their teaching of these topics, and to begin to shed light on where any gaps exist in current teaching practices about promoting the socio-cultural development of learners within this discipline.
Methodology
Topic selection
We chose to focus on the topic of crystal structure and crystallography because it is a foundational topic within chemistry and physics and has contributed to a wide range of engineering advances in nanotechnology, electronics, biotechnology, among others. As evidence of its importance, approximately 40 Nobel prizes in Physics can be found that relate to crystal structure or crystallography.
Article selection
In our literature review, we used the method shown described by Extremera et al. (2020). (2020). Table 1 and Fig. 1 show detailed descriptions of our inclusion/exclusion criteria and a flowchart of the refinement process, respectively. We began by using the following string in the topic search of Web of Science: ((*crystal* OR nanostructure$) AND (teach* OR learn* OR train* OR educati*)). On March 24th, 2021, that query yielded 10928 entries in the database, so the query string was changed to the following in order to yield a manageable number of entries: ((*crystallogra*) AND (teach* OR learn* OR train* OR educati*)). This query yielded 1204 results. Articles with titles and/or keywords that did not pertain to the teaching of crystal structures and crystallography were eliminated, yielding 334 articles.
Table 1 Inclusion and exclusion criteria
Criteria |
Inclusion |
Exclusion |
Time period |
Published from 2010 to March 2021 |
Studies outside this time period |
Literature type |
Peer-reviewed journal articles |
Literature that are not peer-reviewed journal articles (conference proceedings, popular press, book chapters) |
Language |
English |
Non-English |
Accessibility |
Accessible online through the University of Michigan library |
Not accessible |
Topic |
Concerned with teaching and learning of concepts related to crystal structure and crystallography in physical sciences (physics, chemistry, materials science, etc.) |
Not concerned with teaching and learning of concepts related to crystal structure and crystallography, dealing with crystallography in the life sciences, descriptions of historical crystal- and crystallography-based courses not containing description of specific learning activities |
Target educational level |
Undergraduate |
K-12, graduate-only, or professional-only |
Supporting information |
Has supporting information including detailed descriptions of the learning activity |
Does not have supporting information that includes detailed descriptions of the learning activity |
 |
| Fig. 1 Summary of the process used in this review to select relevant works on the subject of crystal structures and crystallography. | |
The list of 334 articles was refined by restricting the search to those articles published between 2010–2021, and removing conference papers or any articles that indicated they were “Work in Progress” or “Preliminary Results.” This refinement was done to restrict our review to contemporary and completed articles, as we are choosing to examine what ways of teaching and instilling disciplinary literacy are valued by practitioners. Conference proceedings in the fields of Chemistry, Physics, Materials Science, and Education generally do not undergo extensive peer review nor contain finished works. While they may provide more examples of activities in use, peer-reviewed articles were exclusively chosen for this study. The abstracts of the remaining 127 articles were read and coded according to three parameters: 1. The topic of the article, such as whether it was concerned largely with crystal structure, crystallography, or with a larger course that may contain those topics. 2. The level of the described intervention or activity, such as whether it was for the public, for high school students, or for university students. 3. The general purpose of the article, such as whether it was aimed strongly at a teaching method or introducing novel visualization tools. Next, articles that focused on biological crystallography were removed as the disciplinary approach used in such articles was seen to be disparate from the approach used by other chemistry, materials science, or physics. Those that focused on undergraduate education were retained, leaving 45 articles. Finally, only articles that included detailed descriptions of the learning activities as Supporting Information were retained, leaving 26 articles to examine. The inclusion of detailed descriptions was determined to be critical to our analysis, as otherwise we would not be able to gather sufficient data to address RQ2, in particular.
General description of the articles
The 26 articles in this review span a range of contexts and origins. All articles describe at least one learning activity aimed at undergraduate students. Most of them (20 articles) were explicitly implemented in a chemistry course, while others were implemented in physics or interdisciplinary crystallography courses (for example, (Stojilovic and Isaacs, 2019)). While most of the courses tended to be upper level or specialized undergraduate courses (17 articles), there were some that were aimed at introductory courses such as general chemistry (for example (Collins, 2011)). The activities were implemented in classrooms in a variety of countries, including in the USA (Collins, 2011), UK (Wilson et al., 2012), Russia (Savchenkov, 2020), China (Ma et al., 2020), Japan (Ohashi, 2015), Germany (Lenzer et al., 2019), and Poland (Duda et al., 2020). Though the articles describe activities that have different academic and national contexts, we include them in our review because they are united by an overarching common factor. Each of the articles was seen as valuable by their respective authors and editors to publish to an international audience of similar disciplinary practitioners.
Coding categories
A content analysis was conducted on the final 26 articles. The analysis consisted of a coding process that identified elements within each article based upon our research questions (Krippendorff, 2004). The elements were coded to describe the phenomena identified, and a codebook was created to keep track of the codes. There were two categories for which each article was examined: themes – the general purpose of the article and learning activities – what the students were asked to do.
The classification of the theme of each article was done according to the author(s)’ specific statements within the articles. In all cases, only one theme was assigned for each article. Themes were then grouped according to their commonalities, leaving six categories: General-Principle Experiment, Specific-Concept Activity, Implementation Guidelines, Technique/Equipment, Research Practice, and Virtual/Physical Models. The General-Principle Experiment theme applied to articles where an experiment activity was chosen to convey a broader concept. The Specific-Concept Activity theme was used to indicate articles in which a specific concept was conveyed to students within an activity designed for that purpose. The Implementation Guidelines theme applied when the aim of the author(s) was to provide advice for how to implement and adapt a certain type of learning activity or teaching tool for a variety of different contents and contexts. The Technique/Equipment theme indicated articles where the goal was to have students learn to perform a certain technique or use a piece of equipment. The Research Practice theme pertained to articles where the primary intention was for students to take part in some authentic research experience. Finally, the Virtual/Physical Model theme described articles that centred around the introduction of a new virtual tool or physical model of a crystal structure or crystallography concept.
There were instances where the learning activities in two articles were largely the same, but the articles were classified differently because the stated purpose of the articles were different. For example, both (Wriedt et al. (2016) and Ismail (2020)) described a learning experience in which students learned to synthesize and characterize a target material. However, whereas Wriedt et al. (2016) presented the purpose of this activity to “teach students basic research tools and procedures” (categorized as Technique/Experiment), Ismail (2020) presented the purpose as “to prepare students for a research-focused independent term project” (categorized as Research Practice). In some cases, there were secondary themes that could be implied based on the text, but in such cases, we attempted to classify the articles based only on what was explicitly stated. For example, many of the articles with the Specific-Concept Activity theme (Collins, 2011; Sunderland, 2014; Ma et al., 2020) introduced a new way of visualizing or modelling crystal structures, but they were not classified as having the Virtual/Physical Model theme because the authors noted that the purpose of the article was the activity that uses the novel visualizations, not the visualizations themselves.
Articles were also coded according to what types of learning activity were used in the overall activity described. Four activity types were identified: Experiment, Computer Visualization, Physical Visualization, and Writing/Speaking. Learning activities classified as Experiment included students conducting some type of experiment such as chemical synthesis or X-ray diffraction. The category Computer Visualization was composed of articles where students use computers to visualize and possibly interact with crystalline models. Activities labelled Physical Visualization were those where students interacted with a physical model of any sort. Finally, the category Writing/Speaking included activities where students write or orally give a response to a prompt of some kind, be it participating in a class discussion, filling out a lab notebook, or describing a phenomenon or finding.
Articles describing specific learning activities were coded according to the types of representations used, as defined by Lesh (Moore et al., 2018), and the role of the learner, as defined by Serafini (2012). Lesh (Moore et al., 2018) described five categories of representations: pictorial (e.g.: schematics, charts, and figures), symbolic (e.g.: mathematical equations), language (e.g.: descriptions using words), concrete (physical models), and Realistic. Lesh defines Realistic representations as those with “Real-World or Experienced Contexts” (Moore et al., 2018). For this review, we categorized representations as Realistic when students either performed experiments or worked with real data.
The role of the learner was coded according to the Expanded Four Resource Model (Serafini, 2012), which consist of four categories–Navigator, Interpreter, Designer, and Interrogator– identifying what role learners take on when interacting with the learning activity. According to Firkins (Firkins, 2021), the four-resource model is a schema and tool that instructors can use to plan and design learning activities. While a student could take on any role while interacting with the learning activity, the learning activities could be intentionally designed to support and activate certain learning roles. In the context of our study, we identified what learner roles were supported in the activities and coded for the four learner roles as follows. The Navigator role was supported whenever students were expected to know or carry out a procedure. For example, one of the articles under study supported the Navigator role by having students conduct a detailed procedure to synthesize and then characterize a covalent organic framework (Lyle et al., 2018). Students took on the Interpreter role whenever they were tasked with analyzing data, interpreting results, or drawing conclusions. The role was illustrated in (Campbell et al., 2016), where students analyzed crystal structure data taken from databases to find specific parameters and judge the quality of the proposed structure. The Designer role was emphasized when students were called to actively engage with the activity and to make choices that affect the activity's trajectory. In (Ismail, 2020), this role was supported by having students design, propose, and conduct individual term projects. Finally, the Interrogator role was seen whenever students were asked to put their current actions and activity in context of the topic at hand, the wider scientific field, or their education. This role was seen in (Wilson et al., 2012), where students collaborated with researchers on authentic crystallography experiments, with a heavy emphasis on the larger context. A learning activity could have been designed to support more than one role. For example, in (Stojilovic and Isaacs, 2019), students familiar with powder diffraction were tasked with doing a diffraction experiment on a large single crystal, where they had to explore ways to mount the crystal (Designer), carry out the diffraction procedure (Navigator), and analyze data that include unexpected results (Interpreter).
Coding validation
In establishing the initial coding procedure for the papers and inter-rater reliability, two model articles were chosen for all four researchers to read and code. Each researcher individually identified the themes, learning activities, and other features of one article, then discussed their findings to ensure everyone had the same working definitions of the terms in use. The process was repeated with the second model article. The researchers then split into pairs to review five articles and confirm that the coding had converged, before each researcher went on to review eight to nine articles independently. After all the articles were coded, the researchers did a final review of all articles to make sure all the salient points to our research questions were noted and included in the coding. At this point, there were articles that made it through the selection process but were excluded as they included crystal structures or crystallography but those topics were incidental, not integral, to the articles' purposes. Lastly, the themes were reviewed and revised and themes that were not initially agreed upon were discussed until a consensus was reached among the team (Lincoln and Guba, 1985). Coding for the four learner roles was conducted in a similar process to that described above for the themes, activities, and representations, the main difference being that it was done by two of the researchers instead of all four.
Results
RQ1: goals and content of the articles
The first research question is concerned with the goals and content of the learning activities described in the articles of our review. Our first line of inquiry is to understand the articles’ explicitly stated purpose (RQ1A). The purpose of each article is categorized into only one of 6 overall themes: General-Principle Experiments, Specific-Concept Activities, Implementation Guidelines, Virtual/Physical Models, Technique/Equipment, and Research Practice (Table 2). The articles are roughly evenly distributed among the six themes.
Table 2 List of the themes of the research articles, the hit counts, and the articles that fall under each analysis category. All counts are out of the total of 26 articles
Classification |
Count |
Ref. |
General-principle experiments |
5 |
(Varberg and Skakuj, 2015; Martín-Ramos et al., 2018; Stojilovic, 2018; Colacino et al., 2019; Stojilovic and Isaacs, 2019) |
Specific-concept activities |
5 |
(Collins, 2011; Reisner et al., 2012; Sunderland, 2014; Duda et al., 2020; Ma et al., 2020) |
Implementation guidelines |
3 |
(Campbell et al., 2016; Malbrecht et al., 2016; Zheng and Campbell, 2018) |
Technique/equipment |
4 |
(Wilson et al., 2012; Thananatthanachon, 2016; Wriedt et al., 2016; Evans and Evans, 2021) |
Research practice |
4 |
(Small et al., 2014; Bazley et al., 2018; Lyle et al., 2018; Ismail, 2020) |
Virtual/physical model |
5 |
(Cushman and Linford, 2015; Ohashi, 2015; Lenzer et al., 2019; Gruber et al., 2020; Savchenkov, 2020) |
RQ1A.
General-principle experiment.
This includes articles that describe a particular experiment or set of experiments to teach a broader principle such as structure–property relationships. The specific experiment is chosen by the authors because it illustrates a general principle that could not be conveyed directly. This theme is exemplified in (Varberg and Skakuj, 2015), where the authors describe how diffraction experiments are used to determine the structural characteristics of various intermetallic compounds including the type of lattice and unit cell dimensions. However, the emphasis is on how to convey the general principle of structural characterization with X-ray diffraction and the intermetallic compounds are chosen to aid that goal. A similar example is seen in (Colacino et al., 2019), where this time the choice of chemical synthesis is made to introduce students to the general principle of mechanochemistry and green chemical synthesis. This theme is also seen in (Martín-Ramos et al., 2018), where the general principle of characterization of pigments found in historical paintings is taught through experiments around the synthesis and powder x-ray diffraction of cobalt-based pigments.
Specific-concept activities.
These articles describe activities that illustrate a particular concept. For example, in (Duda et al., 2020), students are taught how to identify symmetry operations through the analysis of friezes, decorative architectural flourishes at a local historical building. More than half of the articles with the Specific-Concept Activity theme (Collins, 2011; Sunderland, 2014; Ma et al., 2020) describe activities that involve building crystal models and learning their atomic arrangements and spatial properties. For example, in (Collins, 2011), students build specific crystal structures and stacking arrangements of atoms using spherical magnets and marbles and are then guided through calculations of unit cell volumes and packing efficiency.
Implementation guidelines.
These articles describe Implementation Guidelines for various teaching methods in chemical or crystallographic education contexts. In (Malbrecht et al., 2016), the authors describe how extended field trips to experimental facilities can be designed, organized, and implemented to add richness and deeply engage students in the topic at hand, in this case specialized applications of X-ray diffraction. The emphasis of this article is not on the activities and experiments done on the field trip per se, rather it provides advice on how to best plan these field trips. This theme is also seen in (Campbell et al., 2016), which explains how to best implement case studies in crystallography courses, and in (Zheng and Campbell, 2018), which focuses on how to implement a crystallography module into a chemistry course at an institution where X-ray diffraction equipment is unavailable.
Technique/equipment.
The fourth theme, Technique/Equipment, also emerges from the authors’ overall purpose. For these articles, such as (Wilson et al., 2012; Thananatthanachon, 2016), the general aim is to help students learn a particular experimental (Wriedt et al., 2016) or analytical (Evans and Evans, 2021) method. While similar to the General-Principle Experiment category, this category is distinct in that the authors explicitly state that the purpose is to learn the technique itself rather than the principle it embodies. For example, in (Thananatthanachon, 2016) the author's stated purpose was that students learn how to use common synthesis and characterization techniques for crystalline solid state materials, while in (Evans and Evans, 2021), the students are to learn how to do a Rietveld refinement, which is a data analysis technique. The activity or experiments described in the articles are meant to impart specific, transferrable procedural knowledge to students.
Research practice.
In these articles, the author's intended purpose is to give students opportunities for authentic Research Practice. In this case, seen in (Small et al., 2014; Ismail, 2020), the purpose is not to teach principles or concepts, or to train students in the use of particular techniques. Instead, the purpose is to help students learn about and gain appreciation for the process of research, including experimental design, data collection and analysis, and the communication of findings. For example, (Ismail, 2020) describes a semester-long course focused on independent research-like projects concerning inorganic chemical synthesis and characterization of metal-oxide zeolites. Similarly, (Lyle et al., 2018) describes an experimental series that focuses on metal-organic and covalent-organic frameworks and has students go through a mock research article submission process.
Virtual/physical models
These articles, such as (Ohashi, 2015; Gruber et al., 2020), focus less on any particular educational activity and more on the model being introduced for use in educational settings. For instance, (Gruber et al., 2020) describes a new web application that enables the visualization of crystal structures and reports on the application's features. While it includes examples of using the application in a classroom setting and a sample learning activity, the stated purpose of the article is to describe the application itself. Likewise, (Ohashi, 2015) discusses a new method of modelling crystal structures using latex balls cut to fit into unit cell shapes, with detailed instructions on how to properly construct the models. The article also describes a learning activity that has been implemented in classrooms, but the stated purpose and the bulk of the article discusses the principles behind the design of the model, leaving the design of learning activities using the model up to the reader.
RQ1B: learning activity type
We also examined the types of learning activities, or what the students were asked to do (RQ1b). These categories consist of Experiments, Computer Visualization, Physical Visualization, and Writing/Speaking (Table 3). While each article is categorized under one theme based on the author(s) stated purpose, several learning activities could be described in each article.
Table 3 List of the teaching activities of the research articles, the hit counts, and the articles that fall under each analysis category. All counts are out of the total of 26 articles
Classification |
Count |
Ref. |
Experiment |
14 |
(Small et al., 2014; Varberg and Skakuj, 2015; Malbrecht et al., 2016; Thananatthanachon, 2016; Wriedt et al., 2016; Bazley et al., 2018; Lyle et al., 2018; Martín-Ramos et al., 2018; Stojilovic, 2018; Zheng and Campbell, 2018; Colacino et al., 2019; Stojilovic and Isaacs, 2019; Ismail, 2020; Evans and Evans, 2021) |
Computer viz. |
6 |
(Wilson et al., 2012; Campbell et al., 2016; Wriedt et al., 2016; Lyle et al., 2018; Zheng and Campbell, 2018; Gruber et al., 2020) |
Physical viz. |
6 |
(Collins, 2011; Sunderland, 2014; Ohashi, 2015; Lenzer et al., 2019; Ma et al., 2020; Savchenkov, 2020) |
Writing/speaking |
23 |
(Collins, 2011; Reisner et al., 2012; Small et al., 2014; Sunderland, 2014; Cushman and Linford, 2015; Ohashi, 2015; Varberg and Skakuj, 2015; Campbell et al., 2016; Thananatthanachon, 2016; Wriedt et al., 2016; Bazley et al., 2018; Lyle et al., 2018; Martín-Ramos et al., 2018; Stojilovic, 2018; Zheng and Campbell, 2018; Colacino et al., 2019; Lenzer et al., 2019; Stojilovic and Isaacs, 2019; Duda et al., 2020; Gruber et al., 2020; Ismail, 2020; Ma et al., 2020; Evans and Evans, 2021) |
Experiments.
Of the reviewed articles, about half implement Experiments as a learning activity, where students engage in laboratory procedures and research techniques to teach the concepts of crystal structure and its relation to material properties. This category encompasses students engaging in a wide variety of activities. Some articles with this learning activity type describe diffraction experiments on various materials (for example (Stojilovic, 2018)), while others describe material and chemical synthesis (for example (Ismail, 2020) and (Colacino et al., 2019)). This category also includes non-structural characterization experiments (for example (Small et al., 2014)). Often, more than one of these individual activities is done in the experiment series. In one example, students synthesize various intermetallic alloys, then analyze their alloys as well as the elemental constituents using X-ray diffraction to determine the details of their crystal structures (Varberg and Skakuj, 2015). In another case, students are given large crystal specimens of pyrite for X-ray diffraction analysis and are asked to compare and contrast the obtained results with those found in the literature for powdered samples (Stojilovic and Isaacs, 2019).
Computer visualization.
Another subset of articles uses learning activities centred around Computer Visualization, in which students interact with content using a computer interface to visualize crystals. These learning activities can be implemented using structural databases such as the Cambridge Structural Database and integrated into longer courses focused on teaching crystallography (Wilson et al., 2012; Campbell et al., 2016). In these activities, students are provided with visualizations of chemical and crystal structures and are asked to interact with them and identify things like bond lengths and angles. Another approach to learning activities utilizing computer visualization is to provide students with software in which they could view and manipulate 3D models of crystals. In (Gruber et al., 2020), a web-based interactive tool with representations of crystals in unit-cell and extended configurations is developed to supplement instruction.
Physical visualization.
The next type of learning activity requires students to build, manipulate, and interpret physical models of crystal structures to learn such crystallography concepts as including packing efficiency, coordination, and atom counting. The Physical Visualization learning activity gives students tactile and visual feedback as they use physical models to understand the visuo-spatial relationships of atoms in crystal structures. In many of these articles, students assemble balls into different packing/crystal structures unit cells, with different types of materials in use for the atoms (silicone (Ma et al., 2020), magnets, marbles (Collins, 2011), latex (Ohashi, 2015)) and for the unit cells (acrylic boxes (Ohashi, 2015), metal rods (Sunderland, 2014)). Also falling under this activity type is the use of 3D printed model sets of crystals and molecules (Savchenkov, 2020).
Writing/speaking.
The final type of learning activity is called Writing/Speaking learning activities. This type of activity includes anything where students are instructed to write or orally relay ideas in their own words. The learning activities can include writing short responses to an assigned reading, describing their understanding of details of what they have learned regarding crystallography and material properties, or reporting findings of an experiment (Reisner et al., 2012; Campbell et al., 2016). For instance, in (Campbell et al., 2016), students are given case studies based on research to read and use in a guided discussion during class. Other examples include learning activities wherein the student is asked a series of open-ended questions designed to guide their thoughts and learning (Stojilovic, 2018; Stojilovic and Isaacs, 2019). For example, in (Stojilovic and Isaacs, 2019), students perform a diffraction experiment that leads to a deliberately puzzling result, and then are asked to answer a series of leading questionns until they can explain the puzzling result. The most common example of this type of learning activity is writing a lab report (Lyle et al., 2018; Colacino et al., 2019; Ma et al., 2020).
RQ1C: article purpose vs. learning activity type
We also observed the relationships between the themes and learning activities (RQ1C). Fig. 2 shows the normalized frequencies of the four types of learning activities based on their occurrence in articles within each of the six themes, allowing us to see which themes and types of learning activities co-occur and with what frequencies they do so. Each type of learning activity has a unique frequency distribution across the six themes. Writing/Speaking is the most common learning activity type, occurring in the majority of articles and broadly distributed across each of the six article themes. The Experiment learning type is common in General-Principle Experiment, Research Practice, Technique/Equipment, and Implementation Guidelines themes, but is not found at all in the Virtual/Physical Model and Specific-Concept Activity themes. The Physical Visualization learning activity type only occurs in articles with the Virtual/Physical Model theme and, less often, in articles having the Specific-Concept Activity theme. The final activity type, Computer Visualization, occurs in Implementation Guideline-themed articles, and sometimes Research Practice, Technique/Equipment, and Virtual/Physical Model-themed articles, but has no occurrences in articles of the General-Principle Experiment and Specific-Concept Activity themes.
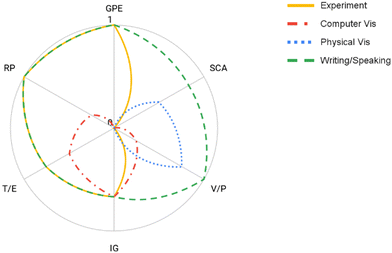 |
| Fig. 2 Radial plot of the frequency of the four learning activity types in articles with the six article themes-General-Principle Experiment (GPE), Specific-Concept Activity (SCA), Virtual/Physical Model (V/P), Implementation Guidelines (IG), Technique/Equipment (T/E), and Research Practice (RP). The frequencies are normalized, representing the fraction of articles with an article theme that reported a particular activity type. A frequency of one means that all articles within a specific article theme include that activity type. The normalized frequencies do not sum to one, because some articles reported as many as four activity types, though each article can have only one article theme. | |
RQ2
Disciplinary literacy tactics.
For research question 2, we examined both what the articles described having the students do (the learning activities) and how those activities employed various types of representations and supported different learner roles.
RQ2A: learning activity type vs. representations used.
Fig. 3 displays the proportion of the articles with a particular learning activity that also have a particular Lesh model representation type, allowing us to see which representation types are the most frequent and in which contexts they are used. The summary of what papers employed which representation types is seen in Table 4. For instance, Fig. 3 shows that of the articles that have Experiment learning activities, almost all of them have Pictorial representations. Two trends regarding the types of representations used are apparent. In all cases, the high frequency of Pictorial, Language, and Symbolic representations is nominally the same across all learning activity types, but the frequency of Realistic versus Concrete representations varies. The Experiment, Computer Visualization, and Writing/Speaking learning activities all exhibit a high frequency of Realistic representations and a low frequency of Concrete representations. In contrast, every article with Physical Visualization learning activities uses some form of Concrete representations (e.g., crystal structure models made from latex balls and acrylic plates in (Ohashi, 2015)), and has no usage of Realistic representations. There are also notable differences in the frequency of representation type between the Experiment, Computer Visualization, and Writing/Speaking learning activities. Articles with the Experiment activity type have the highest occurrences of Realistic representations and a slightly lower frequency of Pictorial representations compared to Computer Visualization and Writing/Speaking learning activities. Computer Visualization learning activities have the lowest frequency of Language representations of all four activity types, with Language representations only being seen in half of the articles. Articles with Writing/Speaking learning activities have the second-lowest occurrence of Realistic representations (after Physical Visualization learning activities), with Realistic representations being moderately frequent.
 |
| Fig. 3 Radial plot of the proportion of the number of papers having a Lesh model representation type and one of the four learning activities divided by the number of papers having that learning activity. A frequency value of one means that the type of representation occurred in all articles within that activity type. Values do not sum to one, because articles may have as many as five representation types. | |
Table 4 List of the Lesh Model representations in the research articles, the hit counts, and the articles that fall under each analysis category. All counts are out of the total of 26 articles
Classification |
Count |
Ref. |
Pictorial |
24 |
(Collins, 2011; Reisner et al., 2012; Wilson et al., 2012; Small et al., 2014; Sunderland, 2014; Cushman and Linford, 2015; Ohashi, 2015; Varberg and Skakuj, 2015; Campbell et al., 2016; Thananatthanachon, 2016; Wriedt et al., 2016; Bazley et al., 2018; Lyle et al., 2018; Martín-Ramos et al., 2018; Stojilovic, 2018; Zheng and Campbell, 2018; Colacino et al., 2019; Lenzer et al., 2019; Stojilovic and Isaacs, 2019; Duda et al., 2020; Gruber et al., 2020; Ismail, 2020; Ma et al., 2020; Evans and Evans, 2021) |
Language |
15 |
(Reisner et al., 2012; Small et al., 2014; Sunderland, 2014; Ohashi, 2015; Varberg and Skakuj, 2015; Campbell et al., 2016; Wriedt et al., 2016; Bazley et al., 2018; Lyle et al., 2018; Martín-Ramos et al., 2018; Colacino et al., 2019; Lenzer et al., 2019; Ismail, 2020; Ma et al., 2020; Evans and Evans, 2021) |
Realistic |
16 |
(Reisner et al., 2012; Wilson et al., 2012; Small et al., 2014; Varberg and Skakuj, 2015; Campbell et al., 2016; Malbrecht et al., 2016; Thananatthanachon, 2016; Wriedt et al., 2016; Bazley et al., 2018; Lyle et al., 2018; Stojilovic, 2018; Zheng and Campbell, 2018; Colacino et al., 2019; Stojilovic and Isaacs, 2019; Ismail, 2020; Evans and Evans, 2021) |
Symbolic |
18 |
(Collins, 2011; Reisner et al., 2012; Small et al., 2014; Sunderland, 2014; Cushman and Linford, 2015; Ohashi, 2015; Varberg and Skakuj, 2015; Campbell et al., 2016; Thananatthanachon, 2016; Wriedt et al., 2016; Bazley et al., 2018; Lyle et al., 2018; Stojilovic, 2018; Colacino et al., 2019; Stojilovic and Isaacs, 2019; Gruber et al., 2020; Savchenkov, 2020; Evans and Evans, 2021) |
Concrete |
8 |
(Collins, 2011; Sunderland, 2014; Ohashi, 2015; Martín-Ramos et al., 2018; Lenzer et al., 2019; Gruber et al., 2020; Ma et al., 2020; Savchenkov, 2020) |
The fact that Language representations are not universal in articles with Writing/Speaking learning activities could seem like a discrepancy, but the two terms are not synonymous. In the Lesh model, Language representations refer to descriptions using words, while the Writing/Speaking learning activity type refers to any case where students are asked to write down or talk about something during the student activity. This definition includes producing descriptions that describe concepts but can also mean performing calculations or answering questions.
RQ2B: Learning activity vs. learner roles.
Fig. 4 shows the proportion of the articles with a particular learning activity that also have a particular Extended Four Resources model learner role, allowing us to see which learner roles are the most frequent and in what contexts they occur. The summary of what papers included which learner roles is seen in Table 5. Interpreter and Navigator occur frequently, and Interrogator and Designer occur much less frequently. Only one activity type, Physical Visualization, has frequent occurrences of the Designer role, but it has no occurrences of Interrogator. In general, the frequent occurrence of the Navigator and Interpreter roles relates to students performing a procedure (Navigator) and collecting and using data (Interpreter). For example, in (Lyle et al., 2018), the students are tasked with performing a computational activity and a lengthy synthesis procedure to create covalent organic frameworks, then collecting structural and functional data from the resulting covalent organic frameworks and comparing it to calculated data. The Designer role is most frequent in articles with the Physical Visualization learning activity, as those activities often give students more freedom to make choices that materially affect the system being modelled. For example, (Ma et al., 2020) has students construct models of various compounds, both small molecules and crystalline unit cells, but it also asks students to construct models of chemicals that interest them and use the models to help explain relationships between bonding, structure, and chemical properties. Least common is the Interrogator role, as the learning activities reviewed do not often discuss how students could put their current doings in the context of the topic, field, or wider education. However, it does occur in some articles, such as (Malbrecht et al., 2016), which describes how a multi-day field trip to a synchrotron site could be arranged and includes both an actual experiment and multiple seminars on crystallography topics that students cite as being illuminating on the breadth of crystallography and its place in scientific research.
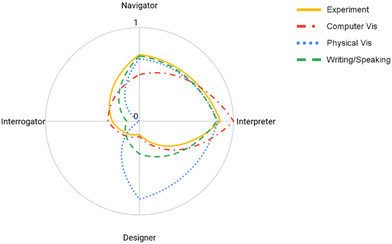 |
| Fig. 4 Radial plot of the proportion of the number of papers having a Four Resources model learner role and one of the four learning activities divided by the number of papers having that learning activity. A value of one means that the learner role occurred in all articles with that learning activity type. Values do not sum to one, as any one article may have as few as one or as many as four learner roles. | |
Table 5 List of the Extended Four Resources Model learner roles in the research articles, the hit counts, and the articles that fall under each analysis category. All counts are out of the total of 26 articles
Classification |
Count |
Ref. |
Navigator |
17 |
(Reisner et al., 2012; Small et al., 2014; Sunderland, 2014; Cushman and Linford, 2015; Ohashi, 2015; Varberg and Skakuj, 2015; Wriedt et al., 2016; Bazley et al., 2018; Lyle et al., 2018; Martín-Ramos et al., 2018; Colacino et al., 2019; Lenzer et al., 2019; Stojilovic and Isaacs, 2019; Gruber et al., 2020; Ismail, 2020; Savchenkov, 2020; Evans and Evans, 2021) |
Interpreter |
21 |
(Collins, 2011; Wilson et al., 2012; Small et al., 2014; Sunderland, 2014; Ohashi, 2015; Varberg and Skakuj, 2015; Campbell et al., 2016; Malbrecht et al., 2016; Thananatthanachon, 2016; Wriedt et al., 2016; Bazley et al., 2018; Lyle et al., 2018; Martín-Ramos et al., 2018; Stojilovic, 2018; Zheng and Campbell, 2018; Lenzer et al., 2019; Stojilovic and Isaacs, 2019; Duda et al., 2020; Gruber et al., 2020; Ismail, 2020; Ma et al., 2020) |
Designer |
9 |
(Collins, 2011; Reisner et al., 2012; Wilson et al., 2012; Sunderland, 2014; Ohashi, 2015; Lenzer et al., 2019; Stojilovic and Isaacs, 2019; Ismail, 2020; Ma et al., 2020) |
Interrogator |
5 |
(Wilson et al., 2012; Small et al., 2014; Malbrecht et al., 2016; Wriedt et al., 2016; Colacino et al., 2019) |
Discussion
In this work, we analyze what is valued by the community of crystal structure and crystallography instructors and the extent to which these instructors use concepts from disciplinary literacy in describing learning activities. After examining 26 articles, we find that the articles tend to fall into six themes in terms of purpose or intent, split evenly between General-Principle Experiment, Specific-Concept Activity, Virtual/Physical Model, Implementation Guidelines, Technique/Equipment, and Research Practice. We also find that the activities themselves tend to be composed of several individual types, of which the most common is Writing/Speaking. Though the activities tend to support learner roles focused on procedural knowledge and analysis, the activities employ a variety of representational types in conjunction with each other. These trends demonstrate that hands-on experience with laboratory settings and discipline-related communication, as well as fluency in different types of representation, are valued by the instructor-practitioners of the discipline and are deemed important to teach to students. This emphasis on experimentation and visualization tools does more than familiarize students with them or train students to carry out specific procedures, it also serves in the enculturation of students into the ways of knowing that are important to the discipline. For example, students' interactions with a physical tool (such as a microscope or a crystal model) can lead both to a situated understanding of how to conduct aspects of scientific work and to access to some of the ways of knowing implicit in the tool's development and application (Airey and Linder, 2009, p. 30).
The 4 E's heuristic
Moje (2015) describes a heuristic for teaching using the socio-cultural perspective of disciplinary literacy. This heuristic is composed of the “four E's:” Engage, Engineer, Examine, and Evaluate. Most of the articles in this literature review, especially those that involve experiments as learning activities, are consistent with the first of the four E's in that they seek to “engage students in the everyday practices of the discipline” (Moje, 2015 p. 261). The General-Principle Experiment, Technique/Equipment, and Research Practice themes are clearly related to scientific practice overall, and even the Implementation Guidelines theme attempts to link learning activities to specific scientific practice. For example, (Ismail, 2020) (classified as Research Practice) describes a seven-week project intended to give students the background and experience necessary in synthesis, characterization, and the research process to conduct their own independent project later in the course. As another example, (Malbrecht et al., 2016) (classified as Implementation Guidelines) describes a substantive field trip to a national lab intended both to give students experience with a high-level research project and allow them to put their crystallography work in the context of the scientific endeavour.
Engage.
The relationship between the themes and the learning activities reveals the ways of knowing that are valued by this community. Moje defines a “disciplinary cycle” within the Engage dimension that is common to virtually any discipline, consisting of problem framing, working with data, using varied media, analyzing and synthesizing findings, examining and evaluating claims, and communicating claims. Many of the themes and learning activities identified in this review are either concerned with the entire disciplinary cycle (Research Practice and Experiments, for example) or portions of the cycle (Virtual/Physical Model and Writing/Speaking, for example). Indeed, the Experiment learning activity appears across most of the themes. Having students do experiments places them in the role of scientists and teaches them how to reason and behave as one. Writing/Speaking is also extremely important, as it appears across all the themes. This prevalence suggests that learning how to communicate technical information and engage in disciplinary discourse is an extremely important goal in science education (Mouton and Grange, 2020), an understandable priority given that instructors of undergraduate-level STEM courses are usually scientists in addition to instructors. The other two activities, namely Computer Visualization and Physical Visualization, are highly localized and confined to a limited subset of themes. Perhaps this localization is partly because said themes (Virtual/Physical Model and Specific-Concept Activity especially) are focused on specific aspects of the disciplinary cycle, namely using varied media, and analyzing and synthesizing findings. The specificity of the relationship between Physical (or Computer) Visualization learning activities and the Virtual/Physical Model (or Implementation Guidelines) theme begs the question of whether these skills are important specifically to teaching crystal structures and crystallography. An analysis of other subtopics within science could reveal additional or different themes and learning activities.
Engineer.
Because undergraduate students are not yet full disciplinary practitioners, Moje contends that instructors need to Engineer (the second of the four E's) the learning activities, so they are a “developmentally appropriate facsimile” of the disciplinary work. Moje posits that it is possible to teach skills (such as conducting a diffraction experiment), but if those skill are not targeted at an appropriate level they are not deeply learned or are learned as abstractions. The articles in this review do not discuss how instructors decided that the learning activities are developmentally appropriate outside of mentioning the students’/courses’ level (e.g.: senior undergraduates, introductory course). The Implementation Guidelines theme attempts to offer some advice on how to design specific types of learning activities, but there is not a strong description of how instructors recognize what is developmentally appropriate, nor what constitutes an appropriate facsimile. Indeed, recognition of students’ prior knowledge, understanding, or skills, or how to teach across their inevitable variability is not discussed.
Moje also asserts that the Engineering level is where content literacy, the disciplinary concepts and vocabulary, resides within the heuristic. The Specific-Concept Activity theme is purely didactic in that it is concerned with imparting content knowledge. For example, (Sunderland, 2014) describes an activity where students build 3D models of crystal structures to learn the atomic placements and observe other features of the structure. The Virtual/Physical Model theme is similarly didactic in nature, introducing and discussing representations of concepts. In Gruber et al. (2020) for example, a new crystal structure visualization application is introduced and described, focusing on the different representations possible in the application and their use for illustrating particular concepts. Both themes deal primarily with introducing students to novel concepts and helping them create and reinforce mental models, an important aspect of content literacy (Moje, 2015).
Examine and evaluate.
The last two E's of Moje's heuristic are related to the use of language and discourse within the discipline. The Examine level exhorts instructors to help their students understand how and why specific technical language is used, and to use technical language appropriately. The predominance of Writing/Speaking learning activities across all themes demonstrates the importance of learning to use technical language correctly. The last E is Evaluate, where instructors provide students with opportunities to determine the appropriateness of technical language in various contexts. In science education, examples of Evaluation may include understanding in what circumstances to use the appropriate number of significant figures for example or explaining scientific findings to various audiences. While we do not explicitly analyze the learning activities with respect to the Evaluate heuristic, authors rarely ask students to discuss scientific concepts in more than one context.
Prevalence of literacy-enhancing tactics
Next, we examine which tactics that are known to enhance disciplinary literacy are explicitly used in the learning activities (RQ2). We find that many authors of the reviewed articles appear to recognize that an important aspect of disciplinary literacy is being able to use multiple representations of any given concept. Our findings demonstrate that instructors teaching crystal structures routinely use 4 out of 5 types of representations over the course of the learning activities, consistent with best practices in the STEM fields (Ainsworth, 1999; Glancy and Moore, 2013). It appears that instructors seem to know, either through intuition, experience, or reading the literature, that presenting scientific concepts using varied representational forms—via illustrations, equations, or written explanation—deepens student learning and enables representational fluency.
Analysis of which learner roles are explicitly supported as part of the learning activities shows that the learning activities emphasize Interpreting and Navigating learner roles, but not Interrogating or Designing roles. Given the fact that Writing/Speaking learning activities are present in many of the learning activities, this finding suggests that the learning activities in question are predominately about having students extract and restate knowledge correctly rather than critically assessing the knowledge. The scientific disciplines clearly value both Interrogating and Designing (as seen in scientific method) in their practice, but this review illustrates that these ideals are not conveyed in the way crystals and crystallography topics are taught, at least not in the articles reviewed here. It could be that this is appropriate for introductory courses, but many of these articles describe activities aimed at upper-level students, suggesting that these aspects of disciplinary literacy are not integrated throughout degree programs.
This review strongly suggests that learning activities, courses, and programs must be explicitly designed to support the roles of Interrogator and Designer in learning experiences. In the case of crystal structures and crystallography education, support of the Designer role most often takes the form of having students freely build and then analyze different crystal structures (Lenzer et al., 2019; Ismail, 2020), but could also extend to having students propose experimental procedures to use in a project. Similarly, the Interrogator role can be supported through explicitly having students consider the relationship between crystallography and the wider discipline of chemistry (Malbrecht et al., 2016), but could also be supported by having students consider what materials in their daily lives could be characterized through crystallography.
Socio-cultural approaches for disciplinary literacy
Another aspect of re-examining the way scientific topics are taught is that it partially determines who studies science. Students pick what to study based on how well their own self-image matches the “prototype” of the field (Perez et al., 2014). Students are more likely to pick fields where they feel comfortable within the culture of that field. The process of becoming literate in a discipline involves the induction of students into the discipline's culture. However, there is a large cultural gap between science and other aspects of students' lives, as science is often perceived as disconnected and irrelevant to day-to-day reality. Using more critical socio-cultural approaches when designing learning activities (such as using familiar aspects of students’ cultural environment to convey a point (Duda et al., 2020)) may be more inclusive. While most of the articles reviewed here do not include such approaches, there is one example seen in (Duda et al., 2020), where students were taught about crystal symmetry using patterns seen in friezes found at a local cultural landmark. Changing pedagogy to bridge the gap between disciplinary culture and students' outside experiences could assist in making the sciences accessible to diverse learners.
Limitations
This review has several limitations that may prevent wide applicability to other contexts. First, the search process was limited by our choice of search engines and by our ability to identify and use search terms that were consistent with how we understand crystal structure and crystallography. As such, it is conceivable that our search process did not capture all the published studies on the teaching of crystal structure and crystallography during the specified time period. Second, our choice of scope for the literature review, namely to focus on peer-reviewed articles, limits the conclusions that can be drawn from it. Perhaps a more in-depth survey of learning activities could be achieved using discourse or curriculum analysis on course teaching materials. Comparing such results to those in this review would provide a glimpse into the differences between what instructors consider necessary to convey to students and what they consider worthy of publication. Third, crystal structure and crystallography may not be a representative subtopic for STEM in general, and our methodology specifically excluded articles concerning its applications in biology, biochemistry, and medicine. However, we tried to keep the research questions specific enough to address variation in this topic and illuminate larger trends while keeping the scope of the review to a manageable level. Some of the themes and learning activities may be specific to teaching crystal structures, such as the prevalence of Specific-Concept Activity-themed articles or physical visualization learning activities. However, the ubiquity of the Writing/Speaking learning activities and focus on experimentation in themes and activity choice suggests that some themes and learning activities may be more generalizable. Even with this understanding in mind, this review is still a plausible representative sample of the published activities about teaching crystal structures and crystallography.
Conclusions
In this review, we examined published learning activities that teach crystal structures and crystallography in undergraduate courses to determine what is valued by the community using disciplinary literacy as the guiding perspective of our analysis. The results revealed that most of the examined articles describe activities directly related to the disciplinary work—conducting experiments, doing analysis, and writing up findings. Additionally, students’ learning was supported utilizing multiple types of representations, including text, symbols, figures, etc. Both practises are well aligned with pedagogies that support disciplinary literacy.
However, this review also revealed that other practices that support disciplinary literacy were not abundant. For example, the analyzed publications lacked adequate description of how learning activities were deemed developmentally appropriate for students. There are articles at other levels of education that describe how developmental appropriateness may be determined and adapted, such as Scott et al. (2019), which discusses how learning activities should be designed to support basic science concepts that are interconnected to one another, and Stevens et al. (2009), which is a book describing the types of prerequisite knowledge learners should have prior to completing an activity, as well as potential areas that students will struggle with and common misconceptions. No discussions of this type were seen the articles analyzed here. Likewise, most often, the analyzed publications supported students in extracting and restating knowledge correctly, but supported students in synthesizing or critically assessing their knowledge or putting their disciplinary knowledge in the context of other fields far less frequently. This review illustrates that the central ideal of scientific method are not conveyed in the way crystals and crystallography topics are taught, at least not in the articles reviewed here. The articles in this review frequently support students in learning the “how” of disciplinary practices (the Navigator and Interpreter learner roles which focus on producing and working with data), but infrequently supported students in learning the “why” of disciplinary practices (the Interrogator role which focuses on contextualizing what students are learning) or the “who/which” (the Designer role which focuses on having students take agency in what they do and make choices). The central idea of scientific method consists of more than just technical knowledge of how to conduct particular experiments, but also why experiments should be done and which experiments should be done. As such, while some few learning activities may support students learning these topics, in general the way central ideals such as this concept are taught in scientific disciplines may not actually be helping students to learn and internalize it.
Author contributions
VC had the roles of Conceptualization, data curation, investigation, visualization, writing – original draft, and writing – review and editing. BAC had the roles of data curation, visualization and writing – review and editing. JM had the roles of project administration, resources, supervision, conceptualization, and writing – review and editing. SR had the roles of conceptualization, methodology, and writing – review and editing.
Conflicts of interest
There are no conflicts to declare.
Acknowledgements
BAC acknowledges support from the Rackham Graduate School at the University of Michigan. VC, BAC and JM acknowledge support from the College of Engineering at the University of Michigan. SR acknowledges support from the University of Tennessee.
References
- Ahn J., (2019), Drawing Inspiration for Learning Experience Design (LX) from Diverse Perspectives Diverse Perspectives, Emerg. Learn. Des. J., 6(1), 1–6.
- Ainsworth S., (1999), The Functions of Multiple Representations, Comput. Educ., 33(2–3), 131–52 DOI:10.1016/S0360-1315(99)00029-9.
- Airey J. and Linder C., (2009), A disciplinary discourse perspective on university science learning: achieving fluency in a critical constellation of modes, J. Res. Sci. Teach., 46(1), 27–49 DOI:10.1002/tea.20265.
- Bazley I. J., Erie E., Feiereisel G., Lewarne C., Peterson J., Sandquist K., Oshin K. and Zeller M., (2018), X-ray Crystallography Analysis of Complexes Synthesized with Tris(2-pyridylmethyl)amine: A Laboratory Experiment for Undergraduate Students Integrating Interdisciplinary Concepts and Techniques, J. Chem. Educ., 95(5), 876–881 DOI:10.1021/acs.jchemed.7b00685.
- Campbell M. G., Powers T. M. and Zheng S. L., (2016), Teaching with the Case Study Method to Promote Active Learning in a Small Molecule Crystallography Course for Chemistry Students, J. Chem. Educ., 93(2), 270–274 DOI:10.1021/acs.jchemed.5b00629.
- Colacino E., Dayaker G., Morère A. and Friščić T., (2019), Introducing Students to Mechanochemistry via Environmentally Friendly Organic Synthesis Using a Solvent-Free Mechanochemical Preparation of the Antidiabetic Drug Tolbutamide, J. Chem. Educ., 96(4), 766–771 DOI:10.1021/acs.jchemed.8b00459.
- Collins D. C., (2011), A unit cell laboratory experiment: Marbles, magnets, and stacking arrangements, J. Chem. Educ., 88(9), 1318–1322 DOI:10.1021/ed200019r.
- Cushman C. V. and Linford M. R., (2015), Using the Plan View To Teach Basic Crystallography in General Chemistry, J. Chem. Educ., 92(8), 1415–1418 DOI:10.1021/acs.jchemed.5b00011.
- Duda M., Rafalska-Łasocha A. and łasocha W., (2020), Plane and Frieze Symmetry Group Determination for Educational Purposes, J. Chem. Educ., 97(8), 2169–2174 DOI:10.1021/acs.jchemed.0c00093.
- Evans J. S. O. and Evans I. R., (2021), Structure Analysis from Powder Diffraction Data: Rietveld Refinement in Excel, J. Chem. Educ., 98(2), 495–505 DOI:10.1021/acs.jchemed.0c01016.
- Extremera J., Vergara D., Dávila L. P. and Rubio M. P., (2020), Virtual and augmented reality environments to learn the fundamentals of crystallography, Crystals, 10(6), 456 DOI:10.3390/cryst10060456.
- Fang Z., (2012), Language correlates of disciplinary literacy, Top. Language Disorders, 32(1), 19–34 DOI:10.1097/TLD.0b013e31824501de.
- Finkenstaedt-Quinn S. A., Halim A. S., Chambers T. G., Moon A., Goldman R. S., Gere A. R. and Shultz G. V.,(2017), Investigation of the Influence of a Writing-To-Learn Assignment on Student Understanding of Polymer Properties, J. Chem. Ed., 94(11), 1610–1617 DOI:10.1021/acs.jchemed.7b00363.
- Firkins A. S., (2021), May 03, The four resource model: a useful framework for planning programs both inside and outside the classroom in development education [Online]. Available at: https://palms.org.au/2021/05/03/the-four-resource-model-a-useful-framework-for-planning-programs-both-inside-and-outside-the-classroom-in-development-education/. (Accessed: 17 May 2022).
- Glancy A. W. and Moore T. J., (2013), Theoretical Foundations for Effective STEM Learning Environments, School of Engineering Education Working Papers, Paper 1.
- Gruber C., James A., Berchtold J., Wood Z., Scott G. and Alghoul Z., (2020) Interactive Unit Cell Visualization Tool for Crystal Lattice Structures, J. Chem. Educ., 97(7), 2020–2024 DOI:10.1021/acs.jchemed.9b01207.
- Ismail M. N., (2020), Hydrothermal Synthesis and Characterization of Titanosilicate ETS-10: Preparation for Research Integrated Inorganic Chemistry Laboratory Course, J. Chem. Educ., 97(6), 1588–1594 DOI:10.1021/acs.jchemed.0c00165.
- Krippendorff K. H., (2004), Content Analysis: An Introduction to Its Methodology, 2nd edn, Sage.
- Lemke J. L., (1998), Teaching All the Languages of Science: Words, Symbols, Images, and Actions, Barcelona: La Caixa Conference on Science Education DOI:10.13140/2.1.4022.5608.
- Lenzer S., Smarsly B. and Graulich N., (2019), Making It Clear: Exploring Crystal Structures by Constructing and Comparing See-Through Models, J. Chem. Educ., 96(8), 1630–1639. DOI:10.1021/acs.jchemed.9b00119.
- Lincoln Y. S. and Guba E. G., (1985), Naturalistic inquiry, Los Angeles: SAGE Publications, Inc.
- Lyle S. J., Flaig R. W., Cordova K. E. and Yaghi O. M., (2018), Facilitating Laboratory Research Experience Using Reticular Chemistry, J. Chem. Educ., 95(9), 1512–1519 DOI:10.1021/acs.jchemed.8b00265.
- Ma Y. Z., Yang Z. L., Wang Y., Wang H. H. and Tian S. J., (2020), Using Magnet-Embedded Silicone Balls to Construct Stable Models for Close-Packed Crystal Structures, J. Chem. Educ., 97(11), 4063–4068 DOI:10.1021/acs.jchemed.0c00515.
- Malbrecht B. J., Campbell M. G., Chen Y. S. and Zheng S. L., (2016), Teaching Outside the Classroom: Field Trips in Crystallography Education for Chemistry Students, J. Chem. Educ., 93(9), 1671–1675 DOI:10.1021/acs.jchemed.6b00073.
- Martín-Ramos P., Susano M., Gil F., Pereira Da Silva P., Martín-Gil J. and Silva M., (2018) Facile Synthesis of Three Kobolds: Introducing Students to the Structure of Pigments and Their Characterization, J. Chem. Educ., 95(8), 1340–1344 DOI:10.1021/acs.jchemed.7b00402.
- Moje E. B., (2015), Doing and Teaching Disciplinary Literacy with Adolescent Learners: A Social and Cultural Enterprise, Harvard Educ. Rev., 85(2), 254–278 DOI:10.17763/0017-8055.85.2.254.
- Moje E. B. and Lewis C., (2007), Examining opportunities to learn literacy: the role of critical sociocultural literacy research, in Lewis C., Enciso P. E. and Moje E. B. (ed.), Reframing sociocultural research on literacy: identity, agency, and power, Routledge, pp. 15–48 DOI:10.4324/9781003064428-3.
- Moore T. J., Guzey S. S., Roehrig G. H. and Lesh R., (2018), Representational Fluency: A Means for Students to Develop STEM Literacy, in Daniel K. L. (ed.) Towards a Framework for Representational Competence in Science Education, Models and Modeling in Science Education. Springer, Vol. 11, pp. 13–30.
- Mouton M., Grange I., (2020), Scientific discourse: Can our first-year students express themselves in science? Int. Conf. Higher Educ. Adv., 579–586.
- Ohashi A., (2015), Using latex balls and acrylic resin plates to investigate the stacking arrangement and packing efficiency of metal crystals, J. Chem. Educ., 92(3), 512–516 DOI:10.1021/ed5006954.
- Ortiz Cáceres L. M. and Candela Rodriguez B. F., (2022), La Estrategia De Escribir Para Aprender: El Caso Del Equilibrio Químico. Góndola, Enseñanza y Aprendizaje de Las Ciencias, 17(1), 168–183 DOI:10.14483/23464712.16531.
- Perez T., Cromley J. G. and Kaplan A., (2014), The role of identity development, values, and costs in college STEM retention, J. Educ. Psychol., 106(1), 315–329 DOI:10.1037/a0034027.
- Reisner B. A., Stewart J., Williams B., Goj L., Holl P., Eppley H. and Johnson A., (2012), Virtual inorganic pedagogical electronic resource learning objects in organometallic chemistry, J. Chem. Educ., 89(2), 185–187 DOI:10.1021/ed200200.
- Reynolds J. A., Thaiss C., Katkin W. and Thompson R. J., (2012), Writing-to-learn in undergraduate science education: a community-based, conceptually driven approach, CBE Life Sci. Educ., 11(1), 17–25 DOI:10.1187/cbe.11-08-0064.
- Rivard, L. P., (1994), A Review of Writing to Learn in Science: Implications for Practice and Research, J. Res. Sci. Teach., 31(9), 969–983.
- Savchenkov A. V., (2020), Designing Three-Dimensional Models That Can Be Printed on Demand and Used with Students to Facilitate Teaching Molecular Structure, Symmetry, and Related Topics, J. Chem. Educ., 97(6), 1682–1687 DOI:10.1021/acs.jchemed.0c00192.
- Serafini F., (2012), Expanding the four resources model: reading visual and multi-modal texts, Pedagogies, 7(2), 150–164 DOI:10.1080/1554480X.2012.656347.
- Scott, E. E., Wenderoth, M. P. and Doherty, J. H., (2019), Learning progressions: An empirically grounded, learner-centered Framework to guide biology instruction. CBE Life Sci. Educ., 18(4), es5 DOI:10.1187/cbe.19-03-0059.
- Small L. J., Wolf S. and Spoerke E. D., (2014), Exploring electrochromics: a series of eye-catching experiments to introduce students to multidisciplinary research, J. Chem. Educ., 91(12), 2099–2104 DOI:10.1021/ed500238j.
- Stevens, S. Y., Sutherland, L. M. and Krajcik, J. S. (2009), The big ideas of nanoscale science & engineering: a guidebook for secondary teachers, National Science Teachers Association Press.
- Stojilovic N., (2018), Using Cu Kα1/Kα2 Splitting and a Powder XRD System to Discuss X-ray Generation, J. Chem. Educ., 95(4), 598–600 DOI:10.1021/acs.jchemed.7b00546.
- Stojilovic N. and Isaacs D. E., (2019), Inquiry-Based Experiment with Powder XRD and FeS2 Crystal: ‘discovering’ the (400) Peak, J. Chem. Educ., 96, 1449–1452 DOI:10.1021/acs.jchemed.9b00099.
- Sunderland D. P., (2014), Studying crystal structures through the use of solid-state model kits, J. Chem. Educ., 91(3), 432–436 DOI:10.1021/ed400367x.
- Teemant A., Smith M. E., Pinnegar S. and Winston Egan M., (2005), Modeling Sociocultural Pedagogy in Distance Education. Teach. Coll. Rec., 107(8), 1675–1698.
- Thananatthanachon T., (2016), Synthesis and Characterization of a Perovskite Barium Zirconate (BaZrO3): An Experiment for an Advanced Inorganic Chemistry Laboratory, J. Chem. Educ., 93(6), 1120–1123 DOI:10.1021/acs.jchemed.5b00924.
- Varberg T. D. and Skakuj K., (2015), X-ray Diffraction of Intermetallic Compounds: A Physical Chemistry Laboratory Experiment, J. Chem. Educ., 92(6), 1095–1097 DOI:10.1021/ed500804b.
- Wilson C. C., Parkin A. and Thomas L. H., (2012), Frontiers of crystallography: A project-based research-led learning exercise, J. Chem. Educ., 89(1), 34–37 DOI:10.1021/ed100953n.
- Wriedt M., Sculley J. P., Aulakh D. and Zhou H. C., (2016), Using Modern Solid-State Analytical Tools for Investigations of an Advanced Carbon Capture Material: Experiments for the Inorganic Chemistry Laboratory, J. Chem. Educ., 93(12), 2068–2073 DOI:10.1021/acs.jchemed.6b00258.
- Zheng S. L. and Campbell M. G., (2018), Connecting Key Concepts with Student Experience: Introducing Small-Molecule Crystallography to Chemistry Undergraduates Using a Flexible Laboratory Module, J. Chem. Educ., 95(12), 2279–2283 DOI:10.1021/acs.jchemed.7b00985.
|
This journal is © The Royal Society of Chemistry 2023 |
Click here to see how this site uses Cookies. View our privacy policy here.