DOI:
10.1039/D3RA06004G
(Paper)
RSC Adv., 2023,
13, 34371-34377
Tannic acid promotes the activation of persulfate with Fe(II) for highly efficient trichloroethylene removal†
Received
3rd September 2023
, Accepted 24th October 2023
First published on 23rd November 2023
Abstract
Trichloroethylene (TCE) is an Environmental Protection Agency (EPA) priority pollutant that is difficult to be removed by some remediation methods. For instance, TCE removal using persulfate (PS) activated by ferrous iron (Fe(II)) has been tested but is limited by the unstable Fe(II) concentration and the initial pH of contaminated water samples. Here we reported a new TCE removal system, in which tannic acid (TA) promoted the activation of PS with Fe(II) (TA-Fe(II)-PS system). The effect of initial pH, temperature, and concentrations of PS, Fe(II), TA, inorganic anions and humic acid on TCE removal was investigated. We found that the TA-Fe(II)-PS system with 80 mg L−1 of TA, 1.5 mM of Fe(II) and 15 mM of PS yielded about 96.2–99.1% TCE removal in the pH range of 1.5–11.0. Radical quenching experiments were performed to identify active species. Results showed that SO4˙− and ˙OH were primarily responsible for TCE removal in the TA-Fe(II)-PS system. In the presence of TA, the Fe-TA chelation and the reduction of TA could regulate Fe(II) concentration and activate persulfate for continuously releasing reactive species under alkaline conditions. Based on the excellent removal performance for TCE, the TA-Fe(II)-PS system becomes a promising candidate for controlling TCE in groundwater.
Introduction
In recent years, the cases of soil and groundwater polluted by chlorinated-volatile organic compounds (Cl-VOCs) have increased dramatically around the world, which has become an environmental problem that cannot be ignored.1–3 According to the statistics of the superfund remedy report 16th edition, 78% of the contaminated sites have excessive Cl-VOCs. Trichloroethylene (TCE) is a typical Cl-VOC, commonly used as an industrial solvent, household cleaner, and metal degreaser.4–7 TCE is classified by the Environmental Protection Agency (EPA) as a priority environmental pollutant because of its greater density than water, stable chemical properties, and toxicity.8,9 It has been reported that exposure to TCE may damage the human central nervous system with major symptoms such as nausea, facial numbness, and incoordination.10,11 Therefore, there is a great need to develop an efficient removal process for TCE.
In order to control the content of TCE in groundwater, several studies have been reported, such as bioremediation processes,12 membrane separation processes,13 physical adsorption processes,14,15 chemical reduction processes,16,17 advanced oxidation processes (AOPs),5,6 etc. Among these technologies, AOPs have received a lot of attention in the last decade.18 AOPs refer to the introduction of strong oxidants into groundwater, which can be activated by some methods, thereby oxidatively degrading the pollutants into low-toxic or non-toxic substances.19–21 Due to their green and environmentally friendly characteristics, AOPs have become a panacea for environmental remediation. Persulfate (PS) is a commonly used oxidant in AOPs. Compared with H2O2, PS has higher redox potential, easier storage way and lower price.22,23 Through activation methods such as heating,24,25 ultrasound,26,27 ultraviolet,28,29 and transition metals,30–32 etc., PS can be activated to generate reactive species, which are responsible for the oxidative degradation of pollutants. Due to the abundant content in nature, ferrous iron (Fe(II)) is considered as a promising homogeneous activator.33,34 Similar to the traditional Fenton reaction, the activation of PS by Fe(II) (Fe(II)-PS system) can generate reactive species such as sulfate radicals (SO4˙−) and hydroxyl radicals (˙OH).24,35 In the pursuit of higher reaction rate, excess Fe(II) is often added to Fe(II)-PS system, which results in the consumption of large amounts of generated SO4˙−.33,36 To solve this problem, some complexing agents, such as ethylenediaminetetraacetic acid (EDTA),33,37 citric acid (CA),38 are used to adjust the concentration of Fe(II) to maintain the reactivity of the Fe(II)-PS system. However, Fe(II) still gradually activate PS and convert to trivalent iron (Fe(III)). Meanwhile, these removal systems generally have better removal percentage under acidic conditions due to the generation of iron hydroxide under alkaline conditions.33
Tannic acid (TA), a biodegradable natural polyphenol, is a benign reducing agent.39 Our previous work showed that the Fe-TA chelation could keep Fe ions stable under alkaline conditions.36,40 Considering the reducing properties and transition metal complexation, we believe that TA facilitate the activation of PS by Fe(II). Therefore, in this study, a new TCE removal system was established in which TA was used to promote Fe(II) activated PS (TA-Fe(II)-PS system). The effects of some reaction parameters, such as TA, PS, and Fe(II) concentrations, temperature, and initial pH on TCE removal were evaluated. Moreover, the effects of inorganic anions and humic acids were used to investigate the potential of TA-Fe(II)-PS system in practical groundwater treatment. Through radical quenching experiments, the reactive species of TA-Fe(II)-PS system were identified. Based on the following results, a reaction mechanism of TA-Fe(II)-PS system for removing TCE was proposed.
Experimental
Materials
Sodium persulfate (Na2S2O8, >99%), ferrous sulfate heptahydrate (FeSO4·7H2O, 99%), sodium chloride (NaCl, 99.8%), sodium bicarbonate (NaHCO3, 99.7%), methanol (CH3OH, GR), tannic acid (C76H52O46, AR), and catechol (C6H6O2, AR) were supplied by Aladdin Reagent Company (Shanghai China). Sodium hydroxide (NaOH, 99%), sulfuric acid (H2SO4, 98%), tert-butanol (C4H10O, 99.5%) and trichloroethylene (C2HCl3, 99%) were purchased from Yuanli Technology (Tianjin, China). p-benzoquinone (C6H4O2, 99%), sodium nitrate (NaNO3, 99.7%) and humic acid were obtained from commercially available chemical reagent companies. Thus, all the commercially chemicals could be used without further purification. Pure water (resistivity 18.2 MΩ) was prepared by a water purification system (Sartorius Arium Pro VF).
TA-Fe(II)-PS system for TCE removal
Batch experiments of TCE removal by TA-Fe(II)-PS system were set up in 100 mL brown anaerobic bottles. Briefly, a certain volume of TCE mother liquor was added to ultrapure water to obtain 100 mL of TCE solution with an initial concentration of 55 mg L−1. Subsequently, 50 mg L−1 of TA, 3 mM of Fe(II), and 15 mM of PS solutions were added to the TCE solution, which was defined as the TA-Fe(II)-PS system. PS, TA-PS, Fe-PS, and catechol-Fe(II)-PS system were established in similar methods and were defined as control groups. Brown anaerobic bottles were placed in a water bath shaker (Julabo SW22, Germany, 120 rpm, 298 K) to start the removal experiment. H2SO4 (0.1 M) or NaOH (0.1 M) solution was used to adjust the initial pH of the rection solutions. The detection method for TCE is similar to our previous work.21,36 Specifically, the concentrations of TCE from 1 mL of water samples were monitored using a gas chromatograph-mass spectrometer (GC-MS, Agilent Technologies, 7890A GC, 5975C MSD) equipped with a headspace sampler (Agilent Technologies G1888). An HP-5 chromatographic column (Agilent Technologies, 19091S-413, 30 m, 0.32 mm, 0.25 μm) was used for the separation of the samples. The mass spectrometer was operated using MS full scan mode at 1.5 kV with a detection range of 35–300 m/z. The effect of the different parameters, including persulfate concentrations (1–20 mM), Fe(II) concentrations (0–15 mM), tannic acid concentrations (0–200 mg L−1), and reaction temperatures (288–308 K) was investigated.
Analytic methods
Removal efficiency. The residue percentage of TCE (Rst, %), and the removal percentage of TCE (Rmt, %) were calculated using the following equations: |
Rmt = (C0 − C)/C0 × 100
| (2) |
where C0 was the initial concentration of TCE, C was the concentration of TCE at the preset time intervals. All experiments were repeated at least three times in parallel and reported as mean values.
Free radicals quenching experiment. In quenching experiments, 1 M of tert-butyl alcohol, 1 M of methanol or 0.1 M of p-benzoquinone was added into the reaction solutions to quench sulfate radicals (SO4˙−), hydroxyl radicals (˙OH) or superoxide radicals (O2˙−) before the start of the experiments. The experimental parameters were similar to those described in Section 2.2 except for the addition of scavengers.
Simulated groundwater analysis. A certain concentration of Cl− (1, 5, 10 mM), HCO3− (1, 5, 10 mM), NO3− (1, 5, 10 mM) or humic acid (10, 50, 100 mg L−1) was introduced into the reaction solution to simulate inorganic anions or natural organic matter present in groundwater. The experimental parameters were set similar to those described in Section 2.2 except for the addition of inorganic anions or natural organic matter.
Detection of Cl− concentrations. The concentrations of Cl− were determined through ion chromatography (Thermo ICS-1100).
Results and discussion
Removal of TCE in different systems
Fig. 1a showed the time profiles of Rst in five removal systems: Blank, PS, TA-PS, Fe(II)-PS and TA-Fe(II)-PS system. It was obvious that the Rst had little change in Blank, PS, and TA-PS system, all less than 10%, which showed that TCE was mainly consumed by volatilization in the above three systems. At the same time, single TA did not have the ability to activate persulfate. As reported in previous studies, Fe(II) could activate persulfate to remove organic pollutants, and 33% of TCE was removed in this work.24 Interestingly, with the addition of TA, the Rmt of TCE in the TA-Fe(II)-PS system was significantly improved, achieving 96.4% in 60 min. This result indicated that TA could promote the removal of TCE through the interaction with Fe ions. The gas chromatograms of the reaction solutions at preset time intervals in the TA-Fe(II)-PS system were shown in Fig. 1b. As the reaction proceeded, the concentration of TCE in the reaction solution dropped rapidly within 5 min, while only a small amount of TCE remained after 60 min. In addition, no other harmful substances were detected during the reaction. Compared with the reported work on TCE removal (Table S1†), the TA-Fe(II)-PS system achieved efficient removal of high concentration of TCE (55 mg L−1), effectively improving the limitations of single Fe(II) activated persulfate to remove pollutants. Nevertheless, some key parameters and mechanisms of the TA-Fe(II)-PS system still need further optimization and exploration.
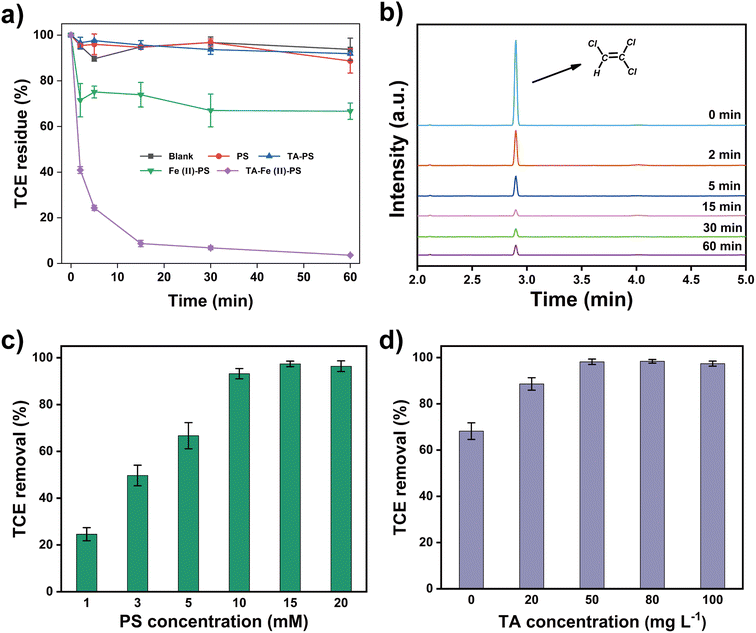 |
| Fig. 1 (a) The comparison of the Rst of TCE in PS, TA-PS, Fe(II)-PS, and TA-Fe(II)-PS system. (b) The gas chromatogram of the aliquots taken out from TA-Fe(II)-PS system at different times. Effect of (c) PS and (d) TA concentrations on TCE removal. Conditions: [TCE] = 55 mg L−1 (a and b) [PS] = 15 mM, [TA] = 50 mg L−1, [Fe(II)] = 3 mM; (c) PS to Fe(II) molar ratio = 1 : 1, [TA] = 100 mg L−1; (d) [PS] = 15 mM, [Fe(II)] = 15 mM. T = 298 K, pH 4.1 ± 0.2. | |
Optimization of different reaction parameters in TA-Fe(II)-PS system
Effect of PS concentration. The effect of PS concentration on TCE removal was shown in Fig. 1c. When the PS concentration was increased from 1 mM to 15 mM, the Rmt increased from 24.6% to 97.4%. Theoretically, adding more PS could generate more reactive species and thus remove more TCE.41 However, when the PS concentration was further increased to 20 mM, the Rmt decreased from 97.6% to 96.4%, suggesting that excess PS would deplete the generated reactive species.36,42 Moreover, excessive PS could pose certain environmental risks. To reduce the amount of persulfate introduced, the PS concentration was set to 15 mM for further optimization.
Effect of TA concentration. In order to confirm the role of TA in the TA-Fe(II)-PS system, the Rmt in the TA-Fe(II)-PS system under different concentrations of TA (0, 20, 50, 80, 100 mg L−1) was investigated. As depicted in Fig. 1d, the Rmt (68.2–98.4%) was positively correlated with the TA concentration when the TA concentration ranged from 0–80 mg L−1. However, higher concentrations of TA (100 mg L−1) did not result in more TCE being removed. Therefore, as a typical complexing agent and reducing agent, TA may have the following two roles in the TA-Fe(II)-PS system. First, through the complexation between TA and Fe ions, the concentration of Fe(II) in the TA-Fe(II)-PS system tended to be stable, so more reactive species were released, which allowed more TCE to be removed.36,40 Second, the reducibility of TA could reduce Fe(III) to Fe(II) (eqn (4)),43,44 so the concentration of Fe(II) in the TA-Fe(II)-PS system could be effectively regulated. Nevertheless, the excess TA might deplete the oxidant in the TA-Fe(II)-PS system, which was consistent with the trend of Rmt in Fig. 1d. Thus, 80 mg L−1 of TA was selected for the next optimization. |
Fe(II) + S2O2−8 → Fe(III) + SO˙−4 + SO2−4
| (3) |
|
Fe(III) + R–Ph–OH → Fe(II) + R–Ph = O
| (4) |
Effect of Fe(II) concentration. The effect of Fe(II) concentration on TCE removal was shown in Fig. 2a. The Rmt increased from 10.0% to 90.7% when the Fe(II) concentration increased from 0 to 0.15 mM. However, when the Fe(II) concentration was further increased threefold, the Rmt increased by only 8.4%, probably because excess Fe(II) would consume the generated reactive species (eqn (5)).45,46 This inference was validated with the addition of higher concentrations of Fe(II) (1.5, 3, and 15 mM). Interestingly, the Rmt decreased slightly when the Fe(II) concentration further increased from 0.6 mM to 15 mM. Based on the above results, we found that with the addition of TA, the required concentration of Fe(II) could be greatly reduced. Through the TA-Fe chelation and the reduction of TA, Fe(II) could be effectively regulated and consistently released in stable concentrations,40,44 thus increasing the number of available reactive species to improve the Rmt. Therefore, 1.5 mM of Fe(II) was chosen in the next experiments. |
Fe(II) + SO˙−4 → Fe(III) + SO2−4
| (5) |
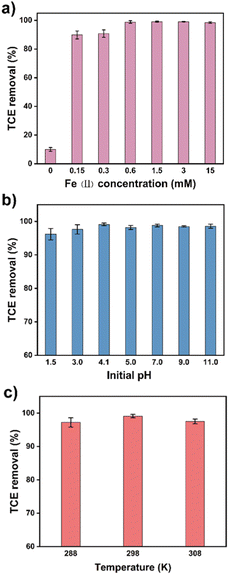 |
| Fig. 2 Effect of (a) Fe(II) concentration, (b) initial pH values, and (c) reaction temperature on TCE removal. Conditions: [TCE] = 55 mg L−1 (a) [PS] = 15 mM, [TA] = 80 mg L−1, T = 298 K, pH 4.1 ± 0.2; (b) [PS] = 15 mM, [TA] = 80 mg L−1, [Fe(II)] = 1.5 mM, T = 298 K; (c) [PS] = 15 mM, [Fe(II)] = 1.5 mM, [TA] = 80 mg L−1, pH 4.1 ± 0.2. | |
Effect of initial pH. The initial pH of the contaminated water sample is one of the critical factors affecting the removal of pollutants by the Fe(II)-PS system. On the one hand, the initial pH determines the form of Fe ions present. Therefore, most of the modified systems perform well under acidic conditions due to the production of iron hydroxides under alkaline conditions.33,38 On the other hand, the initial pH affects the type of reactive species generated in the removal system.36 Fig. 2b illustrated the effect of different initial pH (the initial pH range is from 1.5 to 11, where pH = 4.1 is the pH of contaminated water sample when unadjusted) on TCE removal by TA-Fe(II)-PS system. Surprisingly, the TA-Fe(II)-PS system achieved superior Rmt (96.2–99.1%) over a wide pH range (1.5–11), suggesting that the Fe-TA chelation inhibited the formation of precipitates under alkaline conditions, which facilitated the efficient removal of TCE by the TA-Fe(II)-PS system even under strong alkaline conditions (pH 11). Therefore, the TA-Fe(II)-PS system has good pH adaptability, which is beneficial for practical engineering applications.
Effect of reaction temperature. As seen in Fig. 2c, different reaction temperature (288, 298, or 308 K) was used to examine the effect of temperature on the removal of TCE by the TA-Fe(II)-PS system. As the reaction temperature increased from 288 K to 308 K, there was no obvious difference in Rmt, which indicated that the TA-Fe(II)-PS system had a favorable temperature adaptability.25,47
Effect of simulated groundwater on the TA-Fe(II)-PS system. In actual groundwater treatment, there are some impurities in the water sample, such as inorganic anions, and natural organic matter, etc., which may affect the removal of pollutants. Accordingly, in this work, different concentrations of Cl− (0–10 mM), HCO3− (0–10 mM), NO3− (0–10 mM), and humic acid (0–100 mg L−1) were introduced into the TA-Fe(II)-PS system to simulate the possible impurities in actual groundwater. As shown in Fig. 3a, with the increase of Cl− concentration, the Rmt did not change significantly, but the removal rate of TCE became slower. This result indicated that the reactivity of the TA-Fe(II)-PS system was reduced, probably due to the reaction between Cl− and reactive species to form less reactive chloride radical (Cl˙). The effect of HCO3− on the TA-Fe(II)-PS system was evaluated and presented in Fig. 3b. Obviously, HCO3− inhibited the reactivity of the TA-Fe(II)-PS system, and only 42.9% of TCE was removed with the addition of 10 mM of HCO3−. Due to the hydrolysis of Fe(II) and HCO3−, a large dose of HCO3− consumed part of Fe(II) to form FeCO3, which reduced the dissolved Fe(II) in the reaction solution, thereby inhibiting the reactivity of the TA-Fe(II)-PS system. The inference was consistent with the phenomenon of large amounts of precipitate generated during the removal process. Unlike the two anions mentioned above, the effect of NO3− (Fig. 3c) and humic acid (Fig. 3d) on the TA-Fe system can be neglected. Overall, the order of the effects of the four impurities on the TA-Fe(II)-PS is HCO3− > Cl− > NO3− ≈ humic acid. Based on these results, we believe that the TA-Fe(II)-PS system has the prospect of treating actual groundwater.
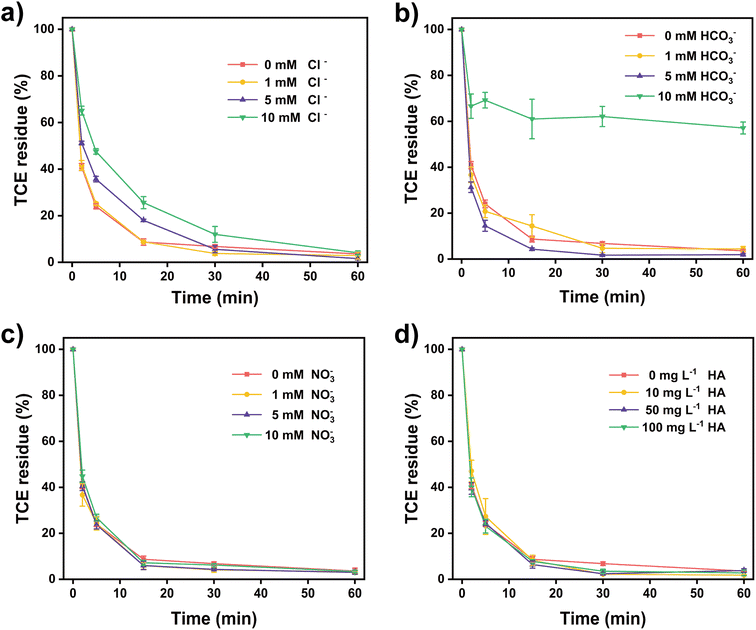 |
| Fig. 3 Effect of (a) Cl−, (b) HCO3−, (c) NO3− and (d) humic acid on TCE removal. Conditions: [TCE] = 55 mg L−1, [PS] = 15 mM, [TA] = 50 mg L−1, [Fe(II)] = 3 mM, T = 298 K, pH 4.1 ± 0.2. | |
Mechanism analysis
To further analyze the activation mechanism of the TA-Fe(II)-PS system, quenching experiments were conducted using several different quenching agents to identify the possible reactive species and their contribution to the removal of TCE. Both methanol (MeOH: k˙OH+MeOH = 9.7 × 108 M−1 S−1 and kSO4˙−+MeOH = 1.1 × 107 M−1 S−1) and tert-butanol (TBA: k˙OH+TBA = 6.8 × 108 M−1 S−1 and kSO4˙−+TBA = 4 × 105 M−1 S−1) are usually used simultaneously to determine the contributions of SO4˙− and ˙OH.48 In addition, p-benzoquinone (BQ) was employed as a quencher of superoxide radicals (O2˙−) with a reaction rate constant of 1 × 109 M−1 S−1.49 As shown in Fig. 4a, the Rmt in the presence of sufficient amount of TBA decreased from 99.1% to 65.2% compared with the no scavenger group, indicating that 33.9% of Rmt was dominated by ˙OH. With the addition of sufficient amount of MeOH, the Rmt decreased to 39.7%, which suggested that 59.4% of TCE was removed by both ˙OH and SO4˙−. Meanwhile, when BQ was added to the TA-Fe(II)-PS system, the Rmt decreased to 95.2% slightly, meaning that less O2˙− was produced during the removal of TCE. Therefore, SO4˙− and ˙OH were considered to be the dominant reactive species in the TA-Fe(II)-PS system, and contribute equally to the removal of TCE.
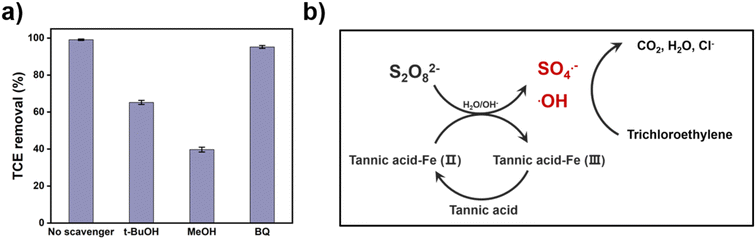 |
| Fig. 4 (a) Effect of radical scavenger (t-BuOH: tert-butyl alcohol; MeOH: methanol; BQ: p-benzoquinone) on the removal of TCE. (b) Schematic illustration of a possible activation mechanism of TA-Fe(II)-PS system on TCE removal. | |
To verify the chelation mechanism of TA and Fe(II), the Rst of TCE was compared between the TA-Fe(II)-PS system and the catechol-Fe(II)-PS system. As shown in Fig. S1,† 99.5% of TCE was removed in the catechol-Fe(II)-PS system within 60 min, indicating that the chelation of Fe(II) and the catechol is crucial for persulfate activation to remove TCE. Compared to TA, the pure catechol exhibited better reaction performance.
Based on the above analysis, a possible activation mechanism of the TA-Fe(II)-PS system was proposed and shown in Fig. 4b. On the one hand, TA complexed the Fe(II) and Fe(III) in the TA-Fe(II)-PS system and inhibit the formation of iron hydroxide precipitation; on the other hand, TA reduced Fe(III) to Fe(II). The above two mechanisms could effectively regulate the concentration of Fe(II), and slow the release of Fe(II) to continuously release reactive species. SO4˙− and ˙OH were the dominant reactive species in the TA-Fe(II)-PS system, attacking TCE to generate non-toxic products such as CO2, Cl−, and H2O.50,51 As shown in Fig. S2,† the Cl− concentrations gradually increased, indicating the organochlorine was converted into Cl−. Therefore, TCE can be efficiently removed in TA-Fe(II)-PS system.
Conclusions
In conclusion, TA promoted Fe(II) activated PS (TA-Fe(II)-PS) system, was constructed in this work for efficient TCE removal (96.2–99.1%) in a wide pH range (1.5–11). Due to the Fe-TA chelation and the reducibility of tannic acid, the concentration of Fe(II) was effectively regulated and slowly released during the removal process to activate persulfate for continuously releasing reactive species. Therefore, the addition of Fe(II) in the TA-Fe(II)-PS system was much lower than that in the Fe(II)-PS system. Moreover, the introduction of TA inhibited the formation of iron hydroxide precipitation, which allowed TCE to be efficiently removed under alkaline conditions. SO4˙− and ˙OH were identified as the dominant reactive species in the TA-Fe(II)-PS system. It was worth mentioning that no other harmful products were detected during the TCE removal, which indicated that the reactivity of the TA-Fe(II)-PS system was strong. Among the common impurities in groundwater, HCO3− and Cl− had obvious inhibitory effects on the TA-Fe(II)-PS system, while NO3− and humic acid had little effect. Based on the above results, we believe that the TA-Fe(II)-PS system is a promising process for TCE treatment.
Author contributions
M. Che: methodology, visualization, writing – original draft. H. Su: investigation, funding acquisition. X. Zhao: investigation, visualization. D. Fu: investigation. R. Huang: conceptualization, project administration, supervision, writing – review & editing. X. Guo: investigation. R. Su: conceptualization, funding acquisition, supervision, writing – review & editing.
Conflicts of interest
There are no conflicts to declare.
Acknowledgements
This work was supported by the Tianjin Municipal Science and Technology Bureau, China (20YFZCSN00650), the National Natural Science Foundation of China (no. 21976132) and Wuqing S&T Commission (WQKJ201806).
References
- B. Huang, C. Lei, C. Wei and G. Zeng, Environ. Int., 2014, 71, 118–138 CrossRef CAS PubMed.
- F. W. Lin, L. Xiang, Z. M. Zhang, N. Li, B. B. Yan, C. He, Z. P. Hao and G. Y. Chen, Crit. Rev. Environ. Sci. Technol., 2022, 52, 311–355 CrossRef CAS.
- H. Si, M. Che, Z. Chen, S. Qiu, M. Cui, R. Huang, W. Qi, Z. He and R. Su, Environ. Chem. Lett., 2021, 19, 3511–3515 CrossRef CAS.
- Z. Li, Y. Sun, Y. Yang, Y. Han, T. Wang, J. Chen and D. C. W. Tsang, J. Hazard. Mater., 2020, 383, 121240 CrossRef CAS PubMed.
- P. Wang, Z. Xu, Y. Liu, X. Sheng, J. Dong, Z. Lu, A. Shan and S. Lyu, Environ. Sci. Pollut. Res., 2022, 29, 53176–53190 CrossRef CAS PubMed.
- Y.-C. Hsu, S.-H. Chang, W.-C. Chung and M.-B. Chang, Environ. Sci. Pollut. Res., 2019, 26, 26276–26285 CrossRef CAS PubMed.
- Y. Su, K. Fu, C. Pang, Y. Zheng, C. Song, N. Ji, D. Ma, X. Lu, C. Liu, R. Han and Q. Liu, Environ. Sci. Technol., 2022, 56, 9854–9871 CrossRef CAS PubMed.
- P. Liu, C. Long, H. M. Qian, Y. Li, A. M. Li and Q. X. Zhang, Chin. Chem. Lett., 2009, 20, 492–495 CrossRef CAS.
- Y.-P. Peng, K.-F. Chen, W.-H. Lin, Y.-C. Chang and F. Wu, RSC Adv., 2016, 6, 41247–41260 RSC.
- F. Lin, Z. Zhang, N. Li, B. Yan, C. He, Z. Hao and G. Chen, Chem. Eng. J., 2021, 404, 126534 CrossRef CAS.
- S. Zhang, T. Wang, X. Guo, S. Chen and L. Wang, Environ. Sci. Pollut. Res., 2023, 30, 14240–14252 CrossRef CAS PubMed.
- G. Pant, D. Garlapati, U. Agrawal, R. G. Prasuna, T. Mathimani and A. Pugazhendhi, J. Hazard. Mater., 2021, 405, 124631 CrossRef CAS PubMed.
- T. J. Ainscough, D. L. Oatley-Radcliffe and A. R. Barron, Membranes, 2021, 11, 61 CrossRef CAS PubMed.
- E. David and V. C. Niculescu, Int. J. Environ. Res. Public Health, 2021, 18, 13147 CrossRef CAS PubMed.
- M. Ahmad, S. S. Lee, S.-E. Oh, D. Mohan, D. H. Moon, Y. H. Lee and Y. S. Ok, Environ. Sci. Pollut. Res., 2013, 20, 8364–8373 CrossRef CAS PubMed.
- F. He, Z. Li, S. Shi, W. Xu, H. Sheng, Y. Gu, Y. Jiang and B. Xi, Environ. Sci. Technol., 2018, 52, 8627–8637 CrossRef CAS PubMed.
- J. Han, J. Xin, X. Zheng, O. Kolditz and H. Shao, Environ. Sci. Pollut. Res., 2016, 23, 14442–14450 CrossRef CAS PubMed.
- J. Zhang, X. Jin, H. Zhao and C. Yang, RSC Adv., 2022, 12, 1904–1913 RSC.
- H. Zheng, Y. Hou, S. Li, J. Ma, J. Nan and T. Li, Chin. Chem. Lett., 2022, 33, 5013–5022 CrossRef CAS.
- Y. Xiang, J. Fang and C. Shang, Water Res., 2016, 90, 301–308 CrossRef CAS PubMed.
- M. Che, J. Xiao, C. Shan, S. Chen, R. Huang, Y. Zhou, M. Cui, W. Qi and R. Su, Water Res., 2023, 243, 120420 CrossRef CAS PubMed.
- K. Tian, L. Hu, L. Li, Q. Zheng, Y. Xin and G. Zhang, Chin. Chem. Lett., 2022, 33, 4461–4477 CrossRef CAS.
- X. Du, Y. Zhang, I. Hussain, S. Huang and W. Huang, Chem. Eng. J., 2017, 313, 1023–1032 CrossRef CAS.
- Y. Liu, Z. Liu, Y. Wang, Y. Yin, J. Pan, J. Zhang and Q. Wang, J. Hazard. Mater., 2018, 342, 326–334 CrossRef CAS PubMed.
- Y. Ji, C. Dong, D. Kong, J. Lu and Q. Zhou, Chem. Eng. J., 2015, 263, 45–54 CrossRef CAS.
- Z.-H. Diao, F.-X. Dong, L. Yan, Z.-L. Chen, W. Qian, L.-J. Kong, Z.-W. Zhang, T. Zhang, X.-Q. Tao, J.-J. Du, D. Jiang and W. Chu, J. Hazard. Mater., 2020, 384, 121385 CrossRef CAS PubMed.
- K. Fedorov, M. Plata-Gryl, J. A. Khan and G. Boczkaj, J. Hazard. Mater., 2020, 397, 122804 CrossRef CAS PubMed.
- J. Yang, M. Zhu and D. D. Dionysiou, Water Res., 2021, 189, 116627 CrossRef CAS PubMed.
- M. Y. Kilic, W. H. Abdelraheem, X. He, K. Kestioglu and D. D. Dionysiou, J. Hazard. Mater., 2019, 367, 734–742 CrossRef CAS PubMed.
- Z. Wang, W. Qiu, S. Pang, Y. Gao, Y. Zhou, Y. Cao and J. Jiang, Water Res., 2020, 172, 115504 CrossRef CAS PubMed.
- X. Li, Y. Jia, M. Zhou, X. Su and J. Sun, J. Hazard. Mater., 2020, 397, 122764 CrossRef CAS PubMed.
- H. Zhen, Y. Hou, S. Li, J. Ma, J. Nan and N. Wang, Chin. Chem. Lett., 2023, 34, 107253 CrossRef.
- Y. Ji, C. Ferronato, A. Salvador, X. Yang and J.-M. Chovelon, Sci. Total Environ., 2014, 472, 800–808 CrossRef CAS PubMed.
- S. Wang and J. Wang, RSC Adv., 2017, 7, 48670–48677 RSC.
- K. Hou, Z. Pi, F. Yao, B. Wu, L. He, X. Li, D. Wang, H. Dong and Q. Yang, Chem. Eng. J., 2021, 407, 127078 CrossRef CAS.
- M. Che, Z. Chen, S. Qiu, M. Cui, R. Huang, W. Qi, Z. He and R. Su, Environ. Chem. Lett., 2021, 19, 4015–4020 CrossRef CAS.
- Y. Liu, G. Qu, Q. Sun, H. Jia, T. Wang and L. Zhu, Chem. Eng. J., 2021, 406, 126774 CrossRef CAS.
- Z.-H. Diao and W. Chu, Sci. Total Environ., 2021, 754, 142155 CrossRef CAS PubMed.
- A. D. Van Diepeningen, A. J. M. Debets, J. Varga, M. Van Der Gaag, K. Swart and R. F. Hoekstra, Mycol. Res., 2004, 108, 919–925 CrossRef CAS PubMed.
- M. Y. Liu, R. L. Huang, M. D. Che, R. X. Su, W. Qi and Z. M. He, Chem. Eng. J., 2018, 352, 716–721 CrossRef CAS.
- D. Guo, S. You, F. Li and Y. Liu, Chin. Chem. Lett., 2022, 33, 1–10 CrossRef CAS.
- G. D. Fang, J. Gao, D. D. Dionysiou, C. Liu and D. M. Zhou, Environ. Sci. Technol., 2013, 47, 4605–4611 CrossRef CAS PubMed.
- N. G. Bastus, F. Merkoci, J. Piella and V. Puntes, Chem. Mater., 2014, 26, 2836–2846 CrossRef CAS.
- L. Zhang, S. S. Wan, C. X. Li, L. Xu, H. Cheng and X. Z. Zhang, Nano Lett., 2018, 18, 7609–7618 CrossRef CAS PubMed.
- G. A. Kifle, Y. Huang, M. Xiang, W. Wang, C. Wang, C. Li and H. Li, Chem. Eng. J., 2022, 442, 136187 CrossRef CAS.
- J. Liu, C. Peng and X. Shi, Environ. Pollut., 2022, 293, 118565 CrossRef CAS PubMed.
- K.-Y. Andrew Lin, H.-A. Chang and C.-J. Hsu, RSC Adv., 2015, 5, 32520–32530 RSC.
- J. Yu, H. Feng, L. Tang, Y. Pang, G. Zeng, Y. Lu, H. Dong, J. Wang, Y. Liu, C. Feng, J. Wang, B. Peng and S. Ye, Prog. Mater. Sci., 2020, 111, 100654 CrossRef CAS.
- S. Waclawek, H. V. Lutze, K. Grubel, V. V. T. Padil, M. Cernik and D. D. Dionysiou, Chem. Eng. J., 2017, 330, 44–62 CrossRef CAS.
- Y.-C. Chang, K.-F. Chen, T.-Y. Chen, H.-H. Chen, W.-Y. Chen and Y.-C. Mao, Chemosphere, 2022, 295, 133906 CrossRef CAS PubMed.
- T.-M. Nguyen, H.-H. Chen, Y.-C. Chang, T.-C. Ning and K.-F. Chen, Chemosphere, 2023, 333, 138954 CrossRef CAS PubMed.
|
This journal is © The Royal Society of Chemistry 2023 |
Click here to see how this site uses Cookies. View our privacy policy here.