DOI:
10.1039/D3RA05552C
(Paper)
RSC Adv., 2023,
13, 28299-28306
Au nanoparticle sensitized blue TiO2 nanorod arrays for efficient Gatifloxacin photodegradation†
Received
15th August 2023
, Accepted 20th September 2023
First published on 26th September 2023
Abstract
TiO2 nanorod arrays have been widely used in photocatalytic processes, but their poor visible light absorption and rapid carrier recombination limit their application. Both introducing oxygen vacancies and using precious metals as surface plasmon resonance (SPR) stimulators are effective strategies to enhance their photocatalytic performance. Herein, Au nanoparticle sensitized blue TiO2 nanorod arrays (Au/B-TiO2) were successfully fabricated for efficient Gatifloxacin photodegradation. The degradation efficiency of Gatifloxacin was up to 95.0%. Moreover, the corresponding reaction rate constant (Ka) was up to 0.02007 min−1. Additionally, it was suggested that Gatifloxacin could be subject to three different degradation pathways. The superior catalytic activity of Au/B-TiO2 is a result of the combined effect of the two components. Firstly, TiO2 nanorod arrays provide a larger surface area for Au deposition and act as efficient transfer channels. Secondly, the presence of oxygen vacancies in blue TiO2 nanorod arrays enhances the catalytic activity. Thirdly, Au acts as a SPR activator, providing a large number of high-energy electrons in the photocatalysis process. Lastly, the improved light capture capabilities are essential for efficient removal of Gatifloxacin. This work provides a new approach for the construction of a high-performance heterojunction photocatalyst in advanced oxidation processes.
1. Introduction
Antibiotics are commonly used to treat a variety of illnesses; Gatifloxacin, a fourth-generation fluoroquinolone, being one of the most popular.1,2 However, due to its toxicity, it is not easily removed from wastewater during the treatment process, leading to the accumulation of Gatifloxacin in natural water bodies and causing issues such as bacterial resistance and water pollution.3,4 Advanced oxidation processes (AOPs) are an effective way to remove antibiotic contaminants from wastewater by using highly active free radicals. Photocatalysis is a particularly popular AOP due to its efficient use of solar energy and its cycle stability, making it an economical and environmentally friendly option.5–7
Titanium dioxide (TiO2) is a popular semiconductor photocatalytic material because of its stability, non-toxicity, and low cost. But two major challenges remain: its low visible light absorption ability due to its wide band gap of 3.0–3.2 eV, and the rapid recombination of carrier pairs due to a large number of defects in the crystal centre.8,9 To address these issues, numerous approaches have been attempted to develop highly active photocatalysts that can absorb more visible light and have low carrier recombination rates.10,11 In recent years, researchers have identified and demonstrated that numerous defective metal-oxide semiconductors containing oxygen vacancies, such as TiO2,12,13 ZnO,14 CeO2,15 and WO3 (ref. 16) exhibit superior catalytic reactivity. These materials have been utilized in various photochemical energy conversions, including photocatalytic H2O2 production, pollutant removal, CO2 reduction, CO oxidation, and water splitting. The oxygen vacancies in these materials can adjust the band structure, lower the band gap, enhance carrier separation and facilitate charge transfer, leading to enhanced catalytic activity.17–19
By activating persulfate (PS) with a blue-TiO2 nanotube anode under electrochemical conditions, Zhou's team discovered that it is possible to decompose 2,4-dichlorophenoxyacetic acid efficiently while consuming minimal energy.20 Lee and colleagues discovered that the photooxidation of hazardous NO gas by blue TiO2 nanoparticles doped with Sn can be utilized as an effective atmospheric pollution remediation agent, achieving an efficiency of 72% in 60 min when exposed to sunlight.21 According to Kumar’ research, blue TiO2 nanorods modified with porous g-C3N4 had a remarkable capacity to convert CO2 to solar fuel, with an efficiency of up to 92%.22 Xu et al.'s research revealed that the blue-TiO2/PbO2-carbon nanotube electrode could completely oxidize 100 mg L−1 phenol in 210 min, offering a promising option for the purification of wastewater with phenol.23 Moreover, it has been demonstrated that a blue TiO2 nanotube array as an anode is successful in degrading 2,4-dichlorophenoxyacetic acid through a photocatalytic/photofenton process, thus providing a potential solution for the disposal of herbicide-containing wastewater.24 In addition, a blue TiO2 photocatalyst, which was supported by photonic crystals, was observed to have a higher CO2 photoreduction activity and improved CH4 selectivity.25
Defect engineering can improve the catalytic efficiency of TiO2, but it is still not able to effectively absorb and utilize the visible light which comprises the majority of the solar energy.26 To further amplify the visible light absorption of TiO2, surface plasmon resonance (SPR) metals have been incorporated, which widens the range of light absorption and provides more active sites to boost the photocatalytic process, thereby supplying hot electrons to the semiconductor, diminishing carrier recombination, and ultimately improving carrier separation.27–29 Au, as a typical SPR metal, has been shown to be an important factor in the photocatalytic performance of TiO2.30 Researchers have demonstrated that Au/TiO2 nanoparticles have good catalytic activity in both thermal and photocatalytic CO2 conversion.31 Furthermore, due to the SPR excited state charges, Au/TiO2 nanostructures have been proven to be beneficial for photochemical (PEC) water splitting and organic pollutant photodegradation.32 It has been observed that the TiO2/Au/TiO2 sandwich-type plasma photocatalysts display improved photocatalytic activity and durability when subjected to visible light.33 Additionally, Rebrov's team discovered that Au/TiO2 can efficiently photo-catalyze the reduction of 4-nitrophenol to 4-aminophenol under green light.34 Au nanorods were also used to modify TiO2 nanobelts in order to improve the light absorption of the full solar spectrum for efficient photocatalytic antibacterial activity applications.35 In conclusion, combining blue TiO2 and plasma Au is an effective method to produce high-performance photocatalysts.
In this work, a novel heterostructure catalyst (Au/B-TiO2) was developed for efficient removal of Gatifloxacin by means of Au sensitized blue TiO2 nanorod arrays. The photocatalytic properties of the catalyst were studied and the degradation pathway and catalytic mechanism of Gatifloxacin were investigated. This research provides potential for the creation of novel heterojunction catalysts and a better comprehension of the pathways and mechanisms of Gatifloxacin decomposition.
2. Experimental
2.1 Materials
Gatifloxacin, chloroauric acid (HAuCl4), sodium borohydride (NaBH4), sulfate (Na2SO4), and p-benzoquinone (BQ) were procured from Aladdin Biochemical Technology Co., Ltd. FTO glasses were purchased from Wu Han Jinge Solar Energy Technology Co., Ltd. Ethylenediaminetetraacetic acid disodium (EDTA–2Na), sodium sulfide (Na2S), and sodium sulfite (Na2SO3) were sourced from Beijing Innochem Science & Technology Co., Ltd. Hydrochloric acid (HCl) and isopropyl alcohol (IPA) were obtained from Sinopharm Chemical Reagent Co., Ltd.
2.2 Production of Au/B-TiO2
The TiO2 nanorod arrays were prepared by traditional hydrothermal process.36 The precursor solution was prepared by combining deionized water, hydrochloric acid and tetrabutyl titanate in a ratio of 15
:
15
:
0.5. Subsequently, the solution was put into a Teflon reactor containing FTO glass and heated at 150 °C for 720 min. The nanorod arrays were then cleaned and dried for future use. To obtain blue TiO2 (B-TiO2) with oxygen vacancies defect, NaBH4 treatment was performed.12 A piece of TiO2 nanorod arrays (3 × 2 cm2) placed in a ceramic kettle, then it was completely covered with ground NaBH4 powder. This was then transferred to a tube furnace for thermal reduction treatment in a N2 atmosphere. The temperature was set to 340 °C and the process was completed in 120 min. Au nanoparticles were then modified on B-TiO2 by photoreduction of HAuCl4.37 B-TiO2 was immersed in HAuCl4 solution with a concentration of 0.6 mg mL−1, and then irradiated under a 39 W mercury lamp for 10, 30, and 60 min to produce Au/B-TiO2-1, Au/B-TiO2-2, and Au/B-TiO2-3, respectively. Finally, they were given a thorough cleaning and dried, in preparation for further use. Fig. 1 illustrates the steps involved in the preparation process.
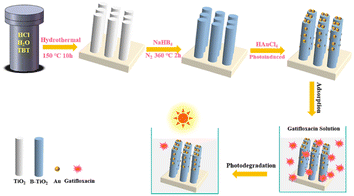 |
| Fig. 1 Diagram of preparation of Au/B-TiO2 system and its photocatalytic process. | |
2.3 Characterizations
An X-ray diffractometer (XRD) from Empyrean S, which utilized Cu Kα radiation, was utilized to obtain XRD data, and a Hitachi-S4800 instrument was used to obtain scanning electron microscopy (SEM) images. For Transmission electron microscopy (TEM) and high-resolution TEM (HRTEM), a Fei-2000 was employed. Additionally, X-ray photoelectron spectroscopy (XPS; ESCALAB-250) was utilized to investigate chemical states, and Electron spin resonance (ESR) measurements were executed utilizing an EMX+ (Bruker).
2.4 Optical and photoelectrochemical investigations
A Shimadzu UV-2550 spectrophotometer was employed to acquire the absorption spectra. To measure photoluminescence (PL) spectra, a Hitachi FL-4500 fluorescence spectrophotometer was utilized. The CHI660D electrochemical workstation, comprising a three-electrode system, was used to record the photocurrent response, electrochemical impedance spectroscopy (EIS) and Mott–Schottky curves. The photocurrent response test was conducted using the Zolix LSH-X500 irradiation source that had a power of 500 mW cm−2. Furthermore, a combination of 0.25 M Na2S and 0.35 M Na2SO3 was used as the electrolyte of EIS, and a 0.1 M Na2SO4 solution was utilized for the photoelectric response and Mott–Schottky test.
2.5 Photodegradation analysis
To evaluate the photodegradation of various samples (2 × 2 cm2), 10 mL of Gatifloxacin (10 mg L−1) was exposed to a 300 W xenon lamp. A dark adsorption period of 30 min was conducted before the light was switched on, to reach absorption equilibrium. LCMS (LTQ Orbitrap XL) was utilized to analyse the decomposition intermediates. An LC-MS was employed with a C18 analytical column, and the liquid flow phases were composed of water and methane. Data acquisition was done in the m/z range of 50–500. The Shimadzu TOC-L CPH was utilized to carry out a Total Organic Carbon (TOC) test.
3. Results and discussion
3.1 Microstructure investigations
The XRD spectra of all samples are displayed in Fig. 2a, showing seven clear characteristic peaks. The peaks at 36.1°, 41.3°, 54.2°, 62.8°, and 69.8° are linked to the (101), (111), (211), (002), and (112) crystal planes of rutile TiO2 respectively, as per JCPDS No. 21-1276. Referring to JCPDS No. 65-2870, the remaining two peaks at 38.2° and 44.4° belong to the (111) and (200) crystal planes of Au, respectively.38 The corresponding optical images of all samples are shown in Fig. 2b.
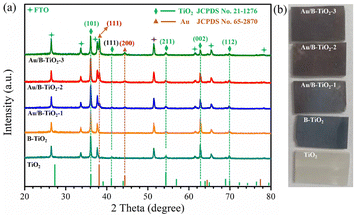 |
| Fig. 2 (a) XRD patterns of each sample, and (b) corresponding optical images. | |
The surface SEM images of TiO2 and B-TiO2, presented in Fig. S1,† demonstrate the dimensions of the TiO2 nanorod arrays to be between 140–200 nm. In addition, Fig. 3a represents the SEM image of Au/B-TiO2-2, and it is evident that a large amount of Au nanoparticles has been successfully modified on the TiO2 nanorod arrays, and the sizes of the Au nanoparticles are between 40–80 nm. HRTEM images in Fig. 3b and c show that the crystal face spacing of 0.25 nm is associated with the (101) plane of rutile TiO2, while the 0.24 nm crystal face spacing corresponds to Au(111).39 These findings are corroborated by the XRD results (Fig. 2a). The element mapping diagram (Fig. 3d) confirms the successful synthesis of Au/B-TiO2.
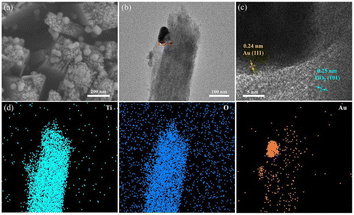 |
| Fig. 3 (a) SEM image of Au/B-TiO2-2, and its (b) TEM image, (c) HRTEM image, and (d) elemental mappings, respectively. | |
3.2 XPS investigations
Verification of the chemical states of Au/B-TiO2-2 was conducted using XPS. The survey is displayed in Fig. 4a, displaying the presence of Au 4f, Ti 2p and O 1s, demonstrating the coexistence of Au, Ti, and O. As demonstrated by Fig. 4b, two distinct peaks at 83.0 eV and 86.7 eV, belonging to Au 4f7/2 and Au 4f5/2, respectively, showing the presence of Au nanoparticles.26 As shown in Fig. 4c, the Ti 2p spectrum is composed of four distinct peaks at 457.4, 458.4, 463.4, and 464.2 eV, which correspond to Ti3+ 2p3/2, Ti4+ 2p3/2, Ti3+ 2p1/2; and Ti4+ 2p1/2, respectively.40 The findings indicate the presences of Ti3+ and oxygen vacancies in the composite.12 Fig. 4d presents the O 1s spectra that is composed of three distinct peaks at 529.6, 531.5 and 533.4 eV, respectively. Ti2O is represented by the 529.6 eV peak, with the 531.5 eV peak being caused by the surface –OH. The third peak at 533.4 eV is caused by the adsorption of H2O on the surface.12
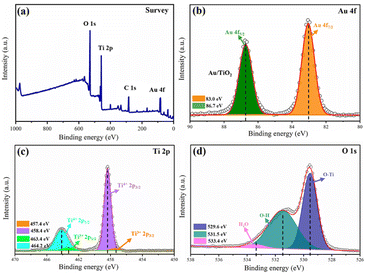 |
| Fig. 4 (a) XPS survey of Au/B-TiO2-2, and its spectra of (b) Au 4f, (c) Ti 2p, and (d) O 1s. | |
3.3 Optical and photoelectrochemical characteristics
The UV-vis absorption spectra were unitized examining the light capture efficiency of each synthesized sample, as depicted in Fig. 5a. Observation revealed that the bandgap of TiO2 (3.03 eV) resulted in a diminished absorption strength for wavelengths less than 400 nm. However, the presence of O vacancies in B-TiO2 increased the absorption strength. Additionally, the sensitization of Au nanoparticles caused the absorption edge to be redshifted and a peak to be observed near 535 nm due to the SPR effect of Au. According to the Tauc equation,41 the bandgaps (Eg) of TiO2, B-TiO2, Au/B-TiO2-1, Au/B-TiO2-2 and Au/B-TiO2-3 are 3.03, 3.01, 3.00, 2.99 and 2.98 eV, respectively (Fig. 5b). The Au/B-TiO2 system's impressive light absorption capacity implies its effectiveness in photocatalysis.
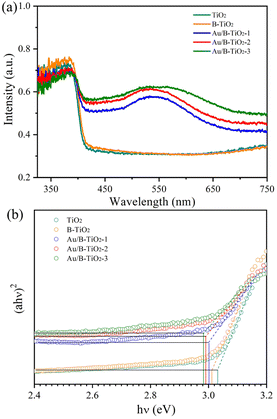 |
| Fig. 5 (a) UV-vis spectra of each sample, and (b) band gaps. | |
The efficiency of carrier separation and transport at the interface of various samples were investigated using photoluminescence (PL). To enhance the catalytic performance of the catalyst, it is necessary to reduce the recombination of photogenerated carriers. A weak PL spectrum indicates fewer carrier pairs recombining, which suggests that more carriers are taking part in the catalytic reaction. Fig. 6 demonstrated that TiO2 had the most intense PL peak, which implied the highest rate of carrier recombination. Additionally, the presence of oxygen vacancies leaded to a lower peak intensity of B-TiO2 in comparison to TiO2. Further, when Au was added to form Au/B-TiO2, the intensity was further reduced. The PL strength of Au/B-TiO2-2 is the weakest, showing that the recombination of photogenerated carrier pairs is minimized and thus, effective charge separation is achieved.
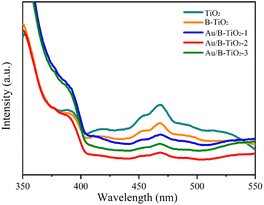 |
| Fig. 6 PL spectra of various samples. | |
Moreover, the photochemical properties of the samples were studied using the photoelectric method which revealed that Au/B-TiO2-2 had the highest current density of 25.4 μA cm−2, 3 times more than B-TiO2 and 6.2 times more than TiO2 (Fig. 7a). This suggests that Au/B-TiO2 has a higher carrier separation efficiency, leading to better photodegradation performance.
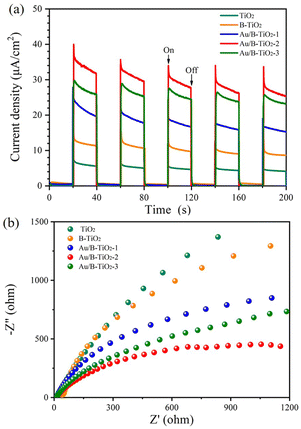 |
| Fig. 7 (a) I–T curves, and (b) EIS curves of each sample. | |
In addition, the electrochemical impedance spectroscopy (EIS) spectra are presented in Fig. 7b, and it is evident that the Au/B-TiO2-2 sample has the smallest radius, indicating the lowest interfacial charge transport resistance and the highest charge transfer ability.
3.4 Optical and photocatalytic analysis
The photocatalytic performance of all samples was assessed by Gatifloxacin decomposition. The UV–vis spectra of Gatifloxacin solution are represented by Fig. S2,† which vary according to the irradiation time, indicating that Gatifloxacin is gradually removed with increasing light exposure. Fig. 8a illustrates the photodegradation efficiency of Gatifloxacin for various samples. After 150 min, TiO2 had the lowest removal rate of 50.4%. For B-TiO2, the photodegradation efficiency increased to 58.6%. The incorporation of Au further improved the photocatalytic efficiency, with Au/B-TiO2-2 achieving the highest rate of 95.0%. This improvement is a result of the combined effect of the components, which reduces the interface charge transfer resistance and enhances catalytic efficiency.17 Secondly, precious metal Au nanoparticles can significantly boost their capacity to absorb visible light through surface plasmon resonance (SPR) effect. Furthermore, as an exceptional provider of plasma electrons, Au can supply an abundance of high-energy electrons into the semiconductor TiO2 in the photocatalytic process.27 Additionally, TiO2 nanorod arrays facilitate electron transport, thereby contributing to the increased photocatalytic efficiency.
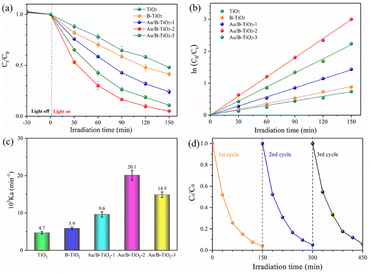 |
| Fig. 8 (a) Photodegradation performance of each sample, (b) kinetics fitting, (c) reaction rate constant, and (d) three-cycle test of Au/B-TiO2-2. | |
The degradation kinetics curve of Gatifloxacin, shown in Fig. 8b, is linear, indicating that it follows the first-order kinetic law. Fig. 8c displays the reaction rate constants (Kα) of each sample, with TiO2 and B-TiO2 being 0.00740 and 0.00588 min−1, respectively. The highest Kα value is found in Au/B-TiO2-2, which is 4.29 and 3.41 times greater than TiO2 and B-TiO2 respectively, at 0.02007 min−1. In addition, recycling performance is an important index to evaluate catalyst. The corresponding cyclic stability investigation of Au/B-TiO2-2 is shown in Fig. 8d. After three cycles, there is only a slight decline, no significant decline, showing good stability. To assess the consequence of the effect of Gatifloxacin concentrations on the photocatalytic performance, experiments were conducted and the results are presented in Fig. S3.† The degradation performance was observed to be 73.8% when the Gatifloxacin concentration increased to 15 mg L−1, which is likely due to the Gatifloxacin molecules covering the active sites, thus hindering the catalytic reaction. All the above-mentioned results show that Au/B-TiO2 system is a kind of photocatalytic nanomaterial with high activity and stability.
3.5 Investigations of decomposition pathways
Furthermore, in order to understand the possible decomposition pathways of Gatifloxacin during the degradation process, the LC-MS technique was used to help us identifying the intermediate products. The MS spectra of intermediates are revealed in Fig. S4–S7.† It can be found that the main m/z corresponding values from large to small are 376, 301, 282, 279, 266, 261, 244, 228, 205, 203, 101, 87, and 74. By analysing the intermediates, Fig. 9 displays three possible photodecomposition pathways of Gatifloxacin; these include demethylation, decarboxylation, deoxygenation, dehydroxylation, hydroxylation, benzene ring breakage, piperazine ring breakage and defluorination.12
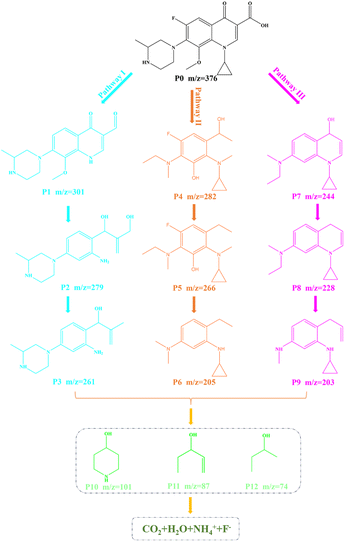 |
| Fig. 9 Possible decomposition pathways of Gatifloxacin. | |
As shown in Fig. 9, at the start of pathway I, P0 (m/z = 376) underwent a series of reactions such as demethylation, dehydroxylation, and defluorination to form P1 (m/z = 301). Then, P2 (m/z = 279) was created by demethylation, hydroxylation, benzene ring cleavage, dehydroxylation, and deoxygenation of P1. Subsequently, P3 (m/z = 261) was produced by dehydroxylation of P2. For pathway II, the reaction began with P0 forming P4 (m/z = 282) through a series of reactions including piperazine ring breakage, demethylation, hydroxylation, dehydroxylation, and benzene ring breakage. P5 (m/z = 266) was then obtained through dehydroxyl and demethyl hydroxylation. Following this, P5 was subjected to demethylation, defluorination, and dehydroxylation, resulting htr in the production of P6 (m/z = 205). On pathway III, P1 experienced piperazine ring cleavage, dehydroxylation, deoxygenation, defluorination, and hydroxylation to generate P7 (m/z = 244). After demethylation of P7, P8 with an m/z of 228 was formed. P9 (m/z = 203) was then obtained by demethylation and breaking of the benzene ring of P8. Further reactions yielded a variety of small molecule structure intermediates, such as m/z = 101, 87, and 74. These results show that macromolecules are gradually broken down, and some of them eventually mineralize to produce H2O, CO2, F−, and NH4+.
In addition, the mineralization performance of Au/B-TiO2-2 was evaluated by TOC removal rate, which was calculated as 66.7% (Fig. S8†) by comparing the TOC[final] and TOC[initial].
3.6 Photocatalytic mechanism analysis
Capturing free radicals was the method used in experiments to investigate the different active groups in the photodegradation process. It is well known that ˙OH, ˙O2− and h+ are the main active groups in photocatalytic reactions.42–44 In this study, EDTA–2Na, BQ and IPA were chosen as trapping agents for ˙OH, ˙O2− and h+, respectively.45 Adding EDTA–2Na, BQ and IPA resulted in the degradation efficiency of Gatifloxacin dropping from 95.0% to 78.6%, 59.5% and 54.8%, respectively, as seen in Fig. 10a. It is evident from these results that these three free radicals influence the photodegradation of Gatifloxacin. Furthermore, ˙OH and ˙O2− have a greater effect than h+. Notably, ˙OH has the most powerful influence as it has multiple sources and can be involved in photocatalytic reactions to remove Gatifloxacin. This is because a portion of ˙OH is created from the interaction of h+ with adsorbed H2O or OH−.46 In addition, ˙OH may be generated from the reaction between H2O2 and ˙O2−.47
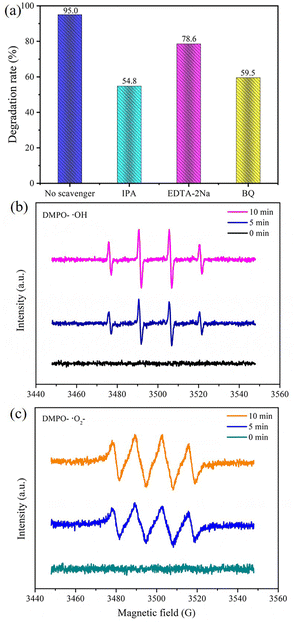 |
| Fig. 10 (a) Free radical capture in Au/B-TiO2 system. (b) EPR of DMPO–˙OH. (c) And DMPO−˙O2−. | |
The ESR technique was used to analyse the presence of ˙OH and ˙O2− during photodegradation. As seen in Fig. 10b and c, no signal was detected in the dark state (0 min). After 5 min of light exposure, a quadruple peak of ˙OH was observed (1
:
2
:
2
:
1), confirming its presence (Fig. 10b). At the same time, four ESR peaks with a 1
:
1
:
1
:
1 area ratio was observed, implying the presence of ˙O2− (Fig. 10c). With the extension of the irradiation time to 10 min, the ESR signals grew stronger, indicating an augmentation in the formation of free radicals.
Generally speaking, when the light used to stimulate a semiconductor catalyst has an energy that is higher than the Eg of the semiconductor, photogenerated holes and electrons are formed. These holes and electrons create active groups that are involved in the breakdown of pollutants. To determine the energy level of the valence band and conductive band for each component, the Mott–Schottky technique was used. As is demonstrated in Fig. S9,† which shows a flat band potential (Efb) of B-TiO2 is −0.30 V vs. Ag/AgCl. According to eqn (1), Efb of B-TiO2 was estimated to be −0.10 V vs. NHE.48
|
ENHE = EAg/AgCl + 0.197
| (1) |
Generally, TiO2 is an n-type semiconductor and its conduction band potential (ECB) is 0.20 eV positive than its Efb.49 Hence, the ECB of B-TiO2 was calculated to −0.30 V vs. NHE. Base on eqn (2) and Eg value (Fig. 5b), the valence band potential (EVB) of B-TiO2 was determined to be 2.68 V vs. NHE,
In addition, on the basis of the outcomes of the prior investigation, the Fermi level (Ef) of Au was 0.81 V vs. NHE.39
According to the above results, the mechanism insight and the photodegradation process of Gatifloxacin by Au/B-TiO2 system are illustrated in Fig. 11. When exposed to light radiation, high energetic electrons created by the SPR of Au will be moved to the conduction band of B-TiO2.50 Meanwhile, electrons and holes were produced at the conduction band and valence band of B-TiO2 under ultraviolet excitation, respectively. Since O2/˙O2− (−0.046 V vs. NHE) has a higher potential than B-TiO2's ECB (−0.30 V vs. NHE), electrons at conduction band of B-TiO2 are able to react with O2 to form ˙O2− (eqn (4)).51 Additionally, since the EVB of B-TiO2 (2.68 V vs. NHE) is more positive than that of H2O/˙OH (2.37 V vs. NHE) and OH−/˙OH (1.99 V vs. NHE). Therefore, interaction between H2O and OH− and the photogenerated holes of B-TiO2 leads to the production of ˙OH (eqn (5) and (6)).52,53 Eventually, Gatifloxacin will be gradually degraded under the action of ˙OH, ˙O2− and h+, eventually becoming H2O, CO2, F−, and NH4+ (eqn (7)).
|
Au/B-TiO2 + hv = Au/B-TiO2 (e− + h+)
| (3) |
|
˙OH/˙O2−/h + Gatifloxacin = CO2 + H2O + F− + NH4+
| (7) |
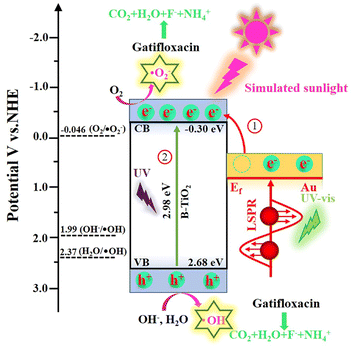 |
| Fig. 11 Mechanism for Gatifloxacin decomposition by Au/B-TiO2 system. | |
4. Conclusions
In this work, an effective heterostructure catalyst composed of Au nanoparticles sensitized blue TiO2 nanorods arrays (Au/B-TiO2) was developed for the removal of Gatifloxacin. The photocatalytic removal ability of this catalyst was proven to be highly efficient with a maximum efficiency of 95.0% and a reaction constant Kα of 0.02007 min−1. Three possible photodegradation pathways of Gatifloxacin were proposed. The remarkable photocatalytic performance of Au/B-TiO2 is a result of combined effect of its each component, such as the TiO2 nanorod arrays which serve as efficient transfer channels and provide a larger surface area for Au deposition, the presence of oxygen vacancies in blue TiO2 nanorod arrays which enhance the catalytic activity, the surface plasmon resonance (SPR) effect of Au which generates high-energy hot electrons, and the improvement of light capture capability. This research presents a successful approach for the production of highly efficient photocatalysts, which can improve the light absorption capacity, promote the efficient separation of photogenerated carriers, and significantly improve the photocatalytic performance.
Author contributions
Jun Guo: conceptualization, methodology, investigation, data curation, and writing–original draft. Wei Gan: investigation, and methodology. Ruixin Chen: data curation. Miao Zhang: funding acquisition, and resources. Zhaoqi Sun: supervision, project administration, and funding acquisition.
Conflicts of interest
There are no conflicts to declare.
Acknowledgements
This work was funded by National Natural Science Foundation of China (No. 51772003 and 51701001). We would also like to express our gratitude to shiyanjia lab (https://www.shiyanjia.com) for ESR test and TOC test.
Notes and references
- A. Arjyal, B. Basnyat, H. T. Nhan, S. Koirala, A. Giri, N. Joshi, M. Shakya, K. R. Pathak, S. P. Mahat, S. P. Prajapati, N. Adhikari, R. Thapa, L. Merson, D. Gajurel, K. Lamsal, D. Lamsal, B. K. Yadav, G. Shah, P. Shrestha, S. Dongol, A. Karkey, C. N. Thompson, N. T. V. Thieu, D. P. Thanh, S. Baker, G. E. Thwaites, M. Wolbers and C. Dolecek, Lancet Infect. Dis., 2016, 16, 535–545 CrossRef CAS PubMed.
- M. Li, J.-W. Xu, J. Li, W. Wang, C. Luo, H. Han, Z.-K. Xu and K. Yao, Bioact. Mater., 2023, 20, 271–285 CrossRef CAS PubMed.
- F. Huang, Z. An, M. J. Moran and F. Liu, J. Hazard. Mater., 2020, 399, 122813 CrossRef CAS PubMed.
- K. Wang, X. Yang and Y. Pei, J. Cleaner Prod., 2023, 409, 137125 CrossRef CAS.
- J. Yan, L. Gong, S. Chai, C. Guo, W. Zhang and H. Wan, Sep. Purif. Technol., 2022, 302, 122016 CrossRef CAS.
- Q. Zhang, J. Chen, X. Gao, H. Che, P. Wang and Y. Ao, Appl. Catal., B, 2022, 313, 121443 CrossRef CAS.
- F. Chang, Y. Xie, J. Zhang, J. Chen, C. Li, J. Wang, J. Luo, B. Deng and X. Hu, RSC Adv., 2014, 4, 28519–28528 RSC.
- Z. Xing, J. Zhang, J. Cui, J. Yin, T. Zhao, J. Kuang, Z. Xiu, N. Wan and W. Zhou, Appl. Catal., B, 2018, 225, 452–467 CrossRef CAS.
- S. Peiris, H. B. Silva, K. N. Ranasinghe, S. V. Bandara and I. R. Perera, J. Chin. Chem. Soc., 2021, 68, 738–769 CrossRef CAS.
- Y. Wei, Q. Wu, H. Meng, Y. Zhang and C. Cao, RSC Adv., 2023, 13, 20584–20597 RSC.
- T. H. A. Nguyen, D. T. Quang, L. V. Tan and T. K. Vo, RSC Adv., 2023, 13, 5859–5868 RSC.
- W. Gan, J. Guo, X. Fu, J. Jin, M. Zhang, R. Chen, C. Ding, Y. Lu, J. Li and Z. Sun, Sep. Purif. Technol., 2023, 317, 123791 CrossRef CAS.
- Q. Zhu, Y. Peng, L. Lin, C.-M. Fan, G.-Q. Gao, R.-X. Wang and A.-W. Xu, J. Mater. Chem. A, 2014, 2, 4429–4437 RSC.
- R. Wang, R. Jiang, C. Dong, T. Tong, Z. Li, H. Liu and X.-W. Du, Ind. Eng. Chem. Res., 2021, 60, 273–280 CrossRef CAS.
- A. Davó-Quiñonero, E. Bailón-García, S. López-Rodríguez, J. Juan-Juan, D. Lozano-Castelló, M. García-Melchor, F. C. Herrera, E. Pellegrin, C. Escudero and A. Bueno-López, ACS Catal., 2020, 10, 6532–6545 CrossRef.
- Y. Chen, L. Wang, R. Gao, Y.-C. Zhang, L. Pan, C. Huang, K. Liu, X.-Y. Chang, X. Zhang and J.-J. Zou, Appl. Catal., B, 2019, 259, 118079 CrossRef CAS.
- S. Wu, M. Y. Manuputty, Y. Sheng, H. Wang, Y. Yan, M. Kraft and R. Xu, Small Methods, 2021, 5, 2000928 CrossRef CAS PubMed.
- Z. Hao, Q. Chen, W. Dai, Y. Ren, Y. Zhou, J. Yang, S. Xie, Y. Shen, J. Wu, W. Chen and G. Q. Xu, Adv. Energy Mater., 2020, 10, 1903107 CrossRef CAS.
- L. Liao, M. Wang, Z. Li, X. Wang and W. Zhou, Nanomaterials, 2023, 13, 468 CrossRef CAS PubMed.
- J. Cai, M. Zhou, X. Du and X. Xu, Sep. Purif. Technol., 2021, 254, 117560 CrossRef CAS.
- A. Martinez-Oviedo, S. K. Ray, H. P. Nguyen and S. W. Lee, J. Photochem. Photobiol., A, 2019, 370, 18–25 CrossRef CAS.
- D. P. Kumar, A. P. Rangappa, H. S. Shim, K. H. Do, Y. Hong, M. Gopannagari, K. A. J. Reddy, P. Bhavani, D. A. Reddy, J. K. Song and T. K. Kim, Mater. Today Chem., 2022, 24, 100827 CrossRef CAS.
- Y. Xu, R. Feng, M. Zhang, C. Yan, J. Liu, T. Zhang and X. Wang, J. Environ. Sci., 2023, 126, 590–601 CrossRef PubMed.
- Y. Zhang, X. Xu, J. Cai, Y. Pan and M. Zhou, Chemosphere, 2021, 266, 129063 CrossRef CAS PubMed.
- D. Lan, W. Sheng, Q. Fu and J. Ge, Nano Res., 2023, 16, 9310–9317 CrossRef CAS.
- S. Cai, J. Chen, Q. Li and H. Jia, ACS Appl. Mater. Interfaces, 2021, 13, 14221–14229 CrossRef CAS PubMed.
- A. Meng, L. Zhang, B. Cheng and J. Yu, Adv. Mater., 2019, 31, 1807660 CrossRef PubMed.
- A. Philip and A. R. Kumar, Coord. Chem. Rev., 2022, 458, 214424 CrossRef CAS.
- S. K. Md Saad, A. Ali Umar, M. I. Ali Umar, M. Tomitori, M. Y. A. Rahman, M. Mat Salleh and M. Oyama, ACS Omega, 2018, 3, 2579–2587 CrossRef CAS PubMed.
- C. Li, T. Wang, Z. J. Zhao, W. Yang, J. F. Li, A. Li, Z. Yang, G. A. Ozin and J. Gong, Angew. Chem., Int. Ed., 2018, 57, 5278–5282 CrossRef CAS PubMed.
- K. Wang, M. Cao, J. Lu, Y. Lu, C. H. Lau, Y. Zheng and X. Fan, Appl. Catal., B, 2021, 296, 120341 CrossRef CAS.
- N. Celebi, M. Y. Aydin, F. Soysal, Y. O. Ciftci and K. Salimi, J. Alloys Compd., 2021, 860, 157908 CrossRef CAS.
- K. Wang, K. Yoshiiri, L. Rosa, Z. Wei, S. Juodkazis, B. Ohtani and E. Kowalska, Catal. Today, 2022, 397–399, 257–264 CAS.
- J. W. Gregory, Y. Gong, Y. Han, S. Huband, R. I. Walton, V. Hessel and E. V. Rebrov, Catal. Today, 2023, 418, 114145 CrossRef CAS.
- Y. Qin, Y. Guo, Z. Liang, Y. Xue, X. Zhang, L. Yang and J. Tian, Chin. Chem. Lett., 2021, 32, 1523–1526 CrossRef CAS.
- Y. Lu, C. Ding, J. Guo, W. Gan, P. Chen, M. Zhang and Z. Sun, J. Alloys Compd., 2022, 924, 166533 CrossRef CAS.
- Y. Yu, X. a. Dong, P. Chen, Q. Geng, H. Wang, J. Li, Y. Zhou and F. Dong, ACS Nano, 2021, 15, 14453–14464 CrossRef CAS PubMed.
- A. Wang, S. Wu, J. Dong, R. Wang, J. Wang, J. Zhang, S. Zhong and S. Bai, Chem. Eng. J., 2021, 404, 127145 CrossRef CAS.
- J. Guo, C. Ding, W. Gan, P. Chen, Y. Lu, J. Li, R. Chen, M. Zhang and Z. Sun, Sep. Purif. Technol., 2023, 307, 122838 CrossRef CAS.
- B. Kim, N. Lee, S. Park, T. Park, J. Song, S. Han, H. Park, D. Lee, H. Kim and H. Jeon, J. Alloys Compd., 2021, 857, 157931 CrossRef CAS.
- D. Dahlan, S. K. Md Saad, A. U. Berli, A. Bajili and A. A. Umar, Phys. E, 2017, 91, 185–189 CrossRef CAS.
- F. Chang, B. Lei, C. Yang, J. Wang and X. Hu, Chem. Eng. J., 2021, 413, 127443 CrossRef CAS.
- G. Liao, Y. Gong, L. Zhang, H. Gao, G.-J. Yang and B. Fang, Environ. Sci. Technol., 2019, 12, 2080–2147 CAS.
- G. Liao, C. Li, X. Li and B. Fang, Cell Rep. Phys. Sci., 2021, 2, 100355 CrossRef CAS.
- J. Guo, W. Gan, C. Ding, Y. Lu, J. Li, S. Qi, M. Zhang and Z. Sun, Sep. Purif. Technol., 2022, 297, 121454 CrossRef CAS.
- S. Wu, H. Hu, Y. Lin, J. Zhang and Y. H. Hu, Chem. Eng. J., 2020, 382, 122842 CrossRef CAS.
- H. Kim, J. Lim, S. Lee, H.-H. Kim, C. Lee, J. Lee and W. Choi, Environ. Sci. Technol., 2019, 53, 2918–2925 CrossRef CAS PubMed.
- J. Yang, L. Li, F. Fu, H. Xu, K. Da, S. Cao, W. Chen, L. Yang and X. Fan, Appl. Surf. Sci., 2023, 610, 155598 CrossRef CAS.
- Z. Yang, X. Xia, L. Shao, L. wang and Y. Liu, Chem. Eng. J., 2021, 410, 128454 CrossRef CAS.
- D. Wibowo, M. Z. Muzakkar, S. K. M. Saad, F. Mustapa, M. Maulidiyah, M. Nurdin and A. A. Umar, J. Photochem. Photobiol., A, 2020, 398, 112589 CrossRef CAS.
- J. S. Adams, A. Chemburkar, P. Priyadarshini, T. Ricciardulli, Y. Lu, V. Maliekkal, A. Sampath, S. Winikoff, A. M. Karim, M. Neurock and D. W. Flaherty, Science, 2021, 371, 626–632 CrossRef CAS PubMed.
- B. He, Z. Wang, P. Xiao, T. Chen, J. Yu and L. Zhang, Adv. Mater., 2022, 34, e2203225 CrossRef PubMed.
- Y. G. Kim and W. K. Jo, J. Hazard. Mater., 2019, 361, 64–72 CrossRef CAS PubMed.
|
This journal is © The Royal Society of Chemistry 2023 |