DOI:
10.1039/D3RA05047E
(Paper)
RSC Adv., 2023,
13, 27912-27922
Phyto-fabrication of ultrafine nanoscale holmium oxide HT-Ho2O3 NPs and their biomedical potential
Received
26th July 2023
, Accepted 14th September 2023
First published on 20th September 2023
Abstract
In this study holmium oxide nanoparticles (Ho2O3 NPs) are fabricated using Hyphaene thebaica extracts as a bioreductant. The XRD pattern of HT-Ho2O3 NPs (product from phyto-reduction) suggested that the nanoparticles are crystalline with no impurities. Scherrer approximation revealed grain sizes of ∼10 nm. The HR-TEM revealed HT-Ho2O3 NPs possessed a quasi-spherical morphology complemented by SEM and the particle sizes were in the range of 6–12 nm. The infrared spectra revealed characteristic Ho–O bonding at ∼603 cm−1. Raman spectra indicated five main peaks positioned at 156 cm−1, 214 cm−1, 328 cm−1, 379 cm−1 and 607 cm−1. Eg (optical bandgap) was found to be 5.1 eV. PL spectra indicated two major peaks at 415 nm and 607 nm. EDS spectra confirmed the elemental presence of holmium (Ho). Spotty rings were obtained during the SAED measurement which indicated crystallinity of HT-Ho2O3 NPs. The HT-Ho2O3 NPs were further analyzed for their antioxidant, anti-angiogenic and cytotoxic properties. The antioxidant potential was moderate i.e., 43.40 ± 0.96% at 1000 μg mL−1 which decreased in a dose dependent manner. Brine shrimp lethality was highest at 1000 μg mL−1 with the LC50 320.4 μg mL−1. Moderate anti-angiogenic potential was observed using in ova CAM assay. MTT bioassay revealed that the HT-Ho2O3 NPs inhibited the 3T3 cells (IC50 67.9 μg mL−1), however, no significant inhibition was observed against MCF-7 cells. α-Amylase and β-glucosidase inhibition revealed that the HT-Ho2O3 NPs can be of use in controlling blood glucose levels. Overall, it can be concluded that biosynthesis using aqueous extracts can be a suitable alternative in finding ecofriendly paradigms for the synthesis of nanoparticles. We suggest extended research into the bioreduced Ho2O3 NPs for establishing their biomedical potential and toxicity.
1. Introduction
Rare earth oxide materials (REMs) such as holmium oxide (Ho2O3) are known for their interesting physicochemical, optical, magnetic, electrical and biological properties. Like other sesquioxides, Ho2O3 is used in various applications including optical applications such as calibration of the wavelength in instruments, pyrolysis, catalysis, electrochemical sensing, memory devices etc.1–3 The basic and catalytic properties of Ho2O3 are reliant on the fabrication methods.4 In the REMs, Ho2O3 NPs are known for elevated lattice energy and a large magnetic moment. Under normal conditions, Ho2O3 has a bixbyite or cubic structure up to 2200 °C, however, at elevated pressures it shows polymorphic monoclinic transformation.5 Ho2O3 NPs supported on MCN have indicated promising applications in radiation therapy.6 Ho2O3 NPs have been previously explored for the photocatalytic degradation of various environmental contaminants.7–9 In medical sciences, the holmium-based lasers are widely used for lithotripsy. Laser energy released from Holmium: YAG is efficiently absorbed in aqueous environments and thus has no side effects like delayed tissue necrosis.10 Neutron-activatable Holmium based materials have been widely applied in the therapeutic radionuclide targeting of tumor cells in systemic radiation therapy.6,11
Various physicochemical processes are suggested for synthesis of Ho2O3 NPs of. These wet chemical and physical approaches includes chemical bath deposition, gel diffusion method, thermal decomposition, sono-chemical, solvothermal hydrothermal methods.1,2 Thermal decomposition of different precursors like Ho(NO3)3·5H2O and Ho2(OH)2(CO3) have been reported for the synthesis Ho2O3 NPs.12–14 Despite of showing effectiveness these physical and chemical processes possess limitations. It is well recognized that the chemical processes can produce noxious waste and are non-biocompatible due to residual chemical precursors which compromises their applications in medicine. Whereas, the physical processes are costly because of their energy needs and sophisticated requirements.15,16 In recent years the plant extracts-based fabrication of metal nanoparticles has emerged as an ecofriendly and economical paradigm.15,17 The nano-bio interface has brought stimulating results and the bio-fabrication of multifunctional metal-based nanoparticles (NPs) with appealing properties represents a trending area in material sciences.18 The abundance and diversity of the natural resources have shifted the thrust towards green chemistry-based synthesis of the nanoparticles.16 Various metal nanoparticles or composite nanomaterials have been fabricated using plant extracts. The nanoparticles reported through the bioreduction process are often reported to have high compatibility and effectiveness as compared to the nanoparticles synthesized from other routes. Generally, the phyto-fabrication is a simple one step process catalyzed by phytochemicals which plays a dual role of reducing the precursor metal salt and subsequently capping for their stabilization of the nanoparticles.
As much of the attention in phyto-reduction based synthesis of metal nanoparticles is centered on silver and gold nanoparticles and there is no report on the phyto-reduction route for the synthesis of Ho2O3 nanoparticles. We for the 1st time successfully report phyto-reduction based synthesis of Ho2O3 nanoparticles using fruit extracts of Hyphaene thebaica as chelating and capping agents. H. thebaica is also known as Egyptian doum and has been reported for diverse medicinal uses in folkloric medicines. Previously, we have successfully fabricated nanoparticles of silver, ceria, iron oxide, bismuth vanadate, zinc oxide, chromium oxide, lanthanum oxide, erbium oxide, europium oxide19–24 using H. thebaica and further evaluated their biomedical potential. Herein, the structural, optical vibrational and functional properties of HT-Ho2O3 NPs (product from phyto-reduction) are reported. Later the HT-Ho2O3 NPs were evaluated for anticancer, antiangiogenic, cytotoxic, antioxidant potential. The study scheme of the current study is indicated in Fig. 1.
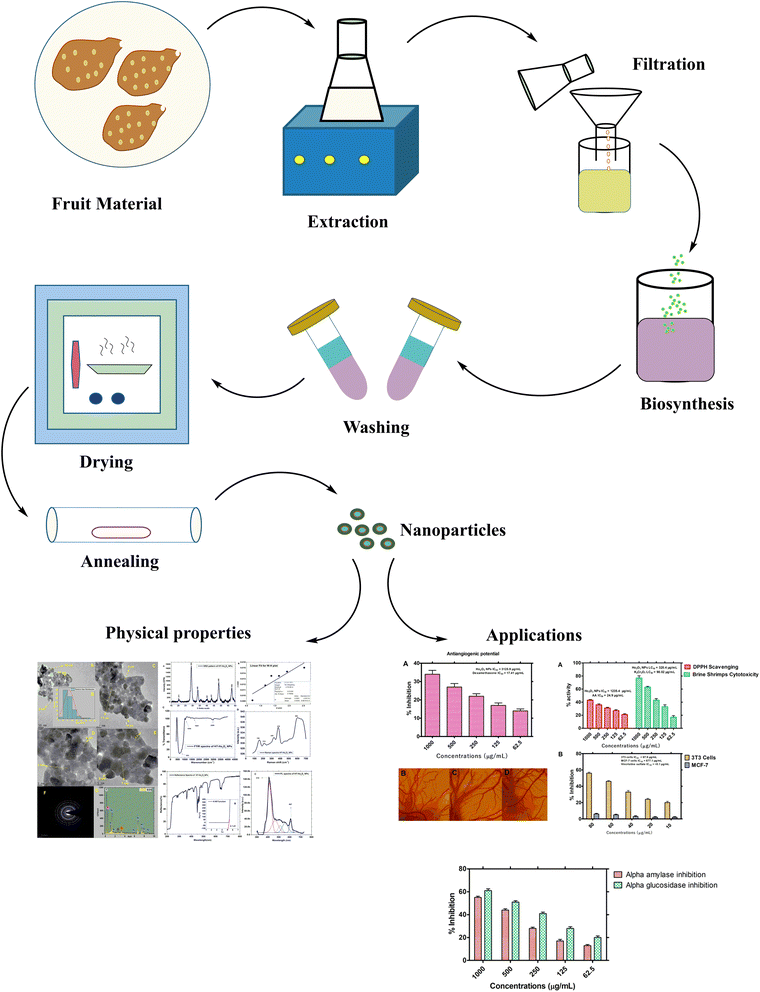 |
| Fig. 1 Study scheme. | |
2. Experimental
2.1. Plant material collection and identification
The plant material was collected in Aswan (Egypt) and the taxonomic identification was done by Dr E. Ammar by comparing it with the already available voucher specimen “29
469” in herbarium, Aswan Botanical Garden, Aswan, Egypt. The plant H. thebaica is commonly available in Egypt and its not a threatened or endangered species. H. thebaica fruits are commonly available in egyptian markets and can also be purchased. The fruit material was rinsed in distil water and dried in shade which followed by grounding them to powder and finally stored in zipper bags. 10 g powdered material was added to dH2O (200 mL), heated for 2 h at 80 °C on a magnetic hotplate. Obtained aqueous extracts were filtered to remove leftover material and the optically transparent liquid extracts were used for further experiments.25
2.2. Biosynthesis of HT-Ho2O3 NPs
For biosynthesis of HT-Ho2O3 NPs, the 100 mL aqueous extracts were treated with 3.5 g precursor salt i.e., holmium nitrate and the reaction mix was heated for 2 h at 60 °C and cooled to room temperature, centrifuged at 5000 RPM for 15 min for collecting the precipitates which were washed thrice in distil water at 5000 RPM. Finally, the precipitates kept in oven at 70 °C for 2 h for drying and later kept in the glass tube furnace for 2 h at 500 °C for annealing.26 The annealed powder assumed as HT-Ho2O3 NPs were used for characterization.
2.3. HT-Ho2O3 NPs characterization
Room temperature X-ray diffraction pattern was obtained to obtain the structural properties of HT-Ho2O3 NPs. The spectral data was used to calculate major XRD values (Table 1). Debye Scherrer approximation (eqn (1)) and W–H plot method (eqn (2)) were applied for the crystallite size estimation. Dislocation density was determined (eqn (3)). FTIR spectra and Raman spectra was used to identify the functional and vibrational characteristics of HT-Ho2O3 NPs. Optical properties were established by diffuse reflectance and photoluminescence spectra. Bandgap was calculating from reflectance by applying K–M function (eqn (4) and (5)). Nanoparticles shape and morphology was observed using HR-TEM and SEM. Image J was used for the processing of the HR-TEM images and calculation of the particle size distribution. Elemental composition was identified by EDS spectra and the SAED pattern was obtained. After establishing the room temperature properties of HT-Ho2O3 NPs, they were subjected to extensive investigations for assessing their biomedical potential.27 |
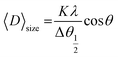 | (1) |
whereas “λ” denote the Cu-Kα radiation X-ray wavelength (1.54 Å), and “K” represents Scherrer constant (0.9), “θ” is the Bragg angle and “β” is the FWHM and “D” represents the crystallite size. |
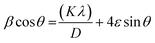 | (2) |
Table 1 Major calculations from XRD data
Miller indices (hkl) |
2θ |
FWHM-β |
D (nm) |
Dislokasyon-δ |
Strain-ε |
211 |
20.41481 |
0.51883 |
14.89634 |
0.004506515 |
0.002228 |
222 |
29.07565 |
0.84919 |
8.9515 |
0.01247982 |
0.003587 |
400 |
33.71933 |
0.70524 |
10.65655 |
0.008805762 |
0.002945 |
332 |
40.12402 |
0.80642 |
9.147165 |
0.011951625 |
0.003305 |
431 |
43.37143 |
0.63955 |
11.40984 |
0.007681407 |
0.002593 |
440 |
48.44382 |
0.84803 |
8.444997 |
0.01402171 |
0.003374 |
622 |
57.56025 |
0.92962 |
7.404007 |
0.018241745 |
0.003555 |
Average |
10.13006 |
0.011098369 |
0.003084 |
On the x-axis, β
cos
θ was plotted against 4
sin
θ followed by obtaining a linear fit for obtaining the y-intercept and strain (ε) for calculating the crystallite size (D) by W–H method.28 Dislocation density “δ” was obtained by eqn (3);29
|
 | (3) |
Optical band gap was obtained using Kubelka–Munk function using eqn (4) and (5). Here, “R” is the diffused reflectance and “F(R)” is the K–M function.22
|
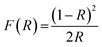 | (4) |
2.4. In vitro FRSA potential
FRSA (Free Radical Scavenging) of HT-Ho2O3 NPs was investigated using DPPH assay.30,31 The DPPH reagent solution (0.004%) was prepared in methanol. Different serial dilutions (62.5 μg mL−1 to 1000 μg mL−1) of HT-Ho2O3 NPs were prepared in distil water, from which 0.1 mL was treated with 3 mL free radical's solution. The reaction mix was incubated in the dark for 30 min. Finally, the readings were recorded at 517 nm by UV spectrophotometer. Ascorbic acid was taken as a positive control in the assay. Percent free radical scavenging was obtained using the following equation; |
 | (6) |
whereas the abs. ct is the absorbance by control and abs. sp was absorbance shown by the test sample.
2.5. In vitro brine shrimp lethality
Brine shrimps or Artemia salina were used in the investigation of the cytotoxic properties of HT-Ho2O3 NPs.32 Artificial sea water (ASW) was used for hatching the larvae. The preparation of ASW included sea salt (40 g) in 1 L distilled water. Shrimps' eggs (2 g) were introduced to the ASW and suspended using an air pump. The container was incubated for 48 h in a warm condition for hatching with a light source on top. Hatched larvae (10 in number) were collected in a separate container and transferred to microplate, which was pre-treated with triplicate serial dilutions (62.5 μg mL−1 to 1000 μg mL−1) of HT-Ho2O3 NPs, followed by incubation for 24 h. After incubation, live and dead larvae were counted for calculation of percent cytotoxicity and LC50. For positive control, Potassium dichromate was applied.
2.6. In ovo antiangiogenic assay
The antiangiogenic properties of HT-Ho2O3 NPs were studied using chorioallantoic membrane assay (CAM).33 Briefly, after incubating fertilized eggs for 4 days incubation at 37 °C the location of embryo was identified followed by removal of 1 mL albumin. Under aseptic conditions, the CAM surface was accessed by creating a small opening. HT-Ho2O3 NPs serial dilutions (62.5 μg mL−1 to 1000 μg mL−1) were prepared in normal saline and applied to the CAM surface by a filter paper disc. For positive and negative controls dexamethasone and normal saline were used respectively. After application of the test samples, the opening was properly sealed and incubated for 48 hours. The antiangiogenic properties were established by counting the blood vessels using the below formula; |
 | (7) |
whereas; “CAMn” is number of blood vessels in CAM treated with normal saline and “CAMs” is blood vessels number in CAM treated with HT-Ho2O3 NPs.
2.7. MTT assay
The MTT (3-(4,5-dimethylthiazolyl-2)-2,5-diphenyltetrazolium bromide) assay for HT-Ho2O3 NPs were performed on MCF-7 and 3T3 cell lines.34,35 Dulbecco's Modified Eagle Medium (DMEM) medium was used for culturing cell lines in microplate. DMEM was supplemented with streptomycin and penicillin at 50 units per mL each and FBS (10%). The cultures were grown up to the density of 6 × 104 cells per well and incubated with different concentration of HT-Ho2O3 NPs after replacing with fresh DMEM media, and kept for 24 h. 20 μL MTT reagent prepared in PBS was added to each well and followed by incubation for 4 h at 37 °C. Finally, DMSO (200 μL) was introduced which dissolved the formazan crystals and the readings were taken at 570 nm. Cellular viability was obtained using eqn (8). |
 | (8) |
2.8. Alpha glucosidase inhibitory assay
Previously described procedure was applied to assess the inhibitory properties of HT-Ho2O3 NPs α-glucosidase enzyme.36 For obtaining the reagent solution the α-glucosidase enzyme (0.5 μg mL−1) was introduced to 120 μL of phosphate buffer (pH 6.9). Serial dilutions of the test sample (1000–62.5 μg mL−1) were prepared. The reagent solution and the serial dilutions were treated initially for 20 min and kept at 37 °C. Afterwards, substrate solution (20 μL) was introduced to reaction mix and incubated for 15 min at 37 °C. Finally, Na2O3 solution (80 μL) was introduced and the absorbance taken at 405 nm. Acarbose was taken as a positive control and a blank mixture without inhibitor agents was used as a negative control. Eqn (9) was applied for calculating the % α-glucosidase inhibition. |
 | (9) |
2.9. Alpha-amylase inhibitory assay
Alpha-amylase enzyme inhibitory effect of the HT-Ho2O3 NPs was performed as described previously.37 Briefly, a 20 μL alpha-amylase and 200 μL from the 0.02 M sodium phosphate buffer was introduced to different concentrations (1000–62.5 μg mL−1) of the test sample, followed by incubation for 10 min at room temperature. The reaction mixture was further added with starch (200 μL) and 400 μL of DNS (dinitrosalicylic acid) reagent and incubated for 5 minutes in a water bath. Finally, 15 mL of double-distilled water was introduced to the mixture and absorbance was recorded at 540 nm. Acarbose taken as a standard α-amylase inhibitor and the percent inhibitory effect was calculated using the formula. |
 | (10) |
3. Results
3.1. Physical properties
3.1.1. Structural properties. Multiple procedures were applied to establish the properties of HT-Ho2O3 NPs. The inset Fig. 2A indicate the XRD pattern of HT-Ho2O3 NPs which suggest that the HT-Ho2O3 NPs were crystalline with no impurities. The obtained Bragg peaks were centered as 20°, 29°, 33°, 39°, 43°, 48° and 57° are ascribed to the crystallographic reflections from (211), (222), (400), (332), (431), (440) and (622) respectively. The observed Bragg peaks corelated to the JCPDS Pattern 044-1268 belonging to the body centered cubic Ho2O3 and space group Ia
. Lattice constants were deduced as <aexp> = 10.61 Å and <zexp> = 16 Å. The crystalline size determined by the Debye–Scherrer equation by using the diffraction data was found to be 10 nm. Major XRD values are summarized in Table 1. For further identifying the crystallite size, the Williamson–Hall equation was used which revealed crystallite size of 17.2 nm. Fig. 2B shows the linear fit after plotting β
cos
θ and 4
sin
θ for obtaining y-intercept and strain. Strain was calculated as 0.003. The Debye Scherrer approximation revealed an average crystallite size of ∼10 nm.
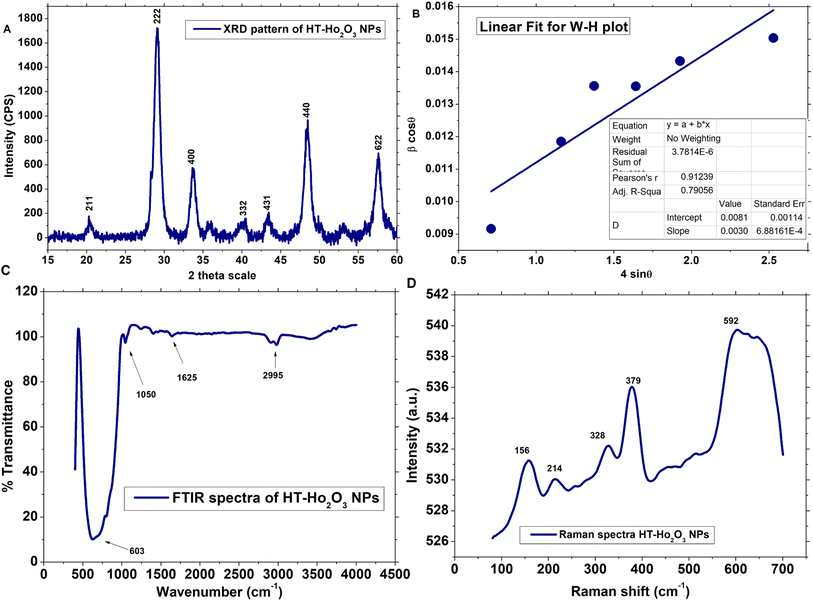 |
| Fig. 2 Structural and functional characterization of the HT-Ho2O3 NPs; (A) XRD pattern (B) W–H plot; (C) FTIR; (D) Raman spectrum. | |
3.1.2. Vibrational properties. The vibrational and functional features of the HT-Ho2O3 NPs were established by FTIR and Raman spectra as shown in Fig. 2C and D respectively. FTIR spectra reveals vibrations centered at ∼603 cm−1, 1050 cm−1, 1625 cm−1 and 2995 cm−1. The intense vibration positioned at ∼603 cm−1 can be ascribed to the traditional metal and oxygen bonding i.e., Ho–O.38 Small peaks located at 1050 cm−1 and 1625 cm−1 can be ascribed to the carbonyl (C
O) and alkenes (C
C) functional group. Fig. 1D reveals the Raman spectra HT-Ho2O3 NPs which indicates five distinct peaks positioned at 156 cm−1, 214 cm−1, 328 cm−1, 379 cm−1 and 590 cm−1 respectively. Generally, the Raman spectra of the rare earth oxide materials is often complex and depend on the excitation wavelength, while it may include PL signals from the metal ions. The intensive Raman peak for rare earth oxide materials is usually observed in the region of 320–420 cm−1. A strong peak at ∼379 cm−1 (Fg + Ag) is the indicative of the well-crystalline Ho2O3 NPs.12,39 Vibration modes at ∼156 cm−1, ∼328 cm−1 and ∼590 cm−1 are also previously reported.39,40
3.1.3. Optical properties. The optical properties of HT-Ho2O3 NPs were established using DRS and PL spectra as indicated in inset Fig. 3A–C. The DRS was analyzed by K-M function for the determination of the optical bandgap (Eg) which was calculated as 5.1 eV which is well agreed in the literature and can be attributed to the blue shift due to decrease in the size.2 Other authors have reported Eg values of 3.2 eV,7 4.95 eV,1 5.0 eV (ref. 41) etc. The PL spectra of the HT-Ho2O3 NPs is indicated in Fig. 2C revealing two major emission peaks at 415 nm and a relatively less intense peak at 607 nm reflects electronic transition from ground state of 5I8 to 5G5 and 5F5 5I8 → 5G5 for 415 nm and 5I8 → 5F5.
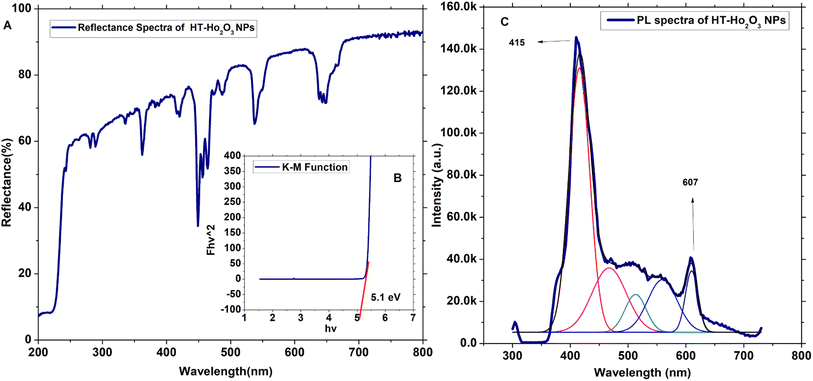 |
| Fig. 3 Optical characteristics of HT-Ho2O3 NPs; (A) DRS spectrum; (B) Eg calculation by K–M function; (C) PL spectrum. | |
3.1.4. Surface morphology and composition. HT-Ho2O3 NPs morphology was established using HR-TEM as indicated in the inset Fig. 4A, C and D. The nanoparticles were having spherical to cuboidal shapes with most of the nanoparticles were in the range of 6–10 nm as indicated in Fig. 4B. Selected area electron diffraction pattern revealed spotty circular rings which reflects the crystalline nature of the HT-Ho2O3 NPs. The energy dispersive spectra confirmed the presence of holmium and oxygen. In the EDS spectra other peaks i.e., carbon and potassium. Presence of carbon is attributed to which carbon coated grid whereas the elemental presence of potassium can be ascribed to the plant material. SEM images were acquired to study the surface morphology of the nanoparticles which showed that the nanoparticles were mostly spherical in shape. The SEM images are indicated in Fig. 5A and B.
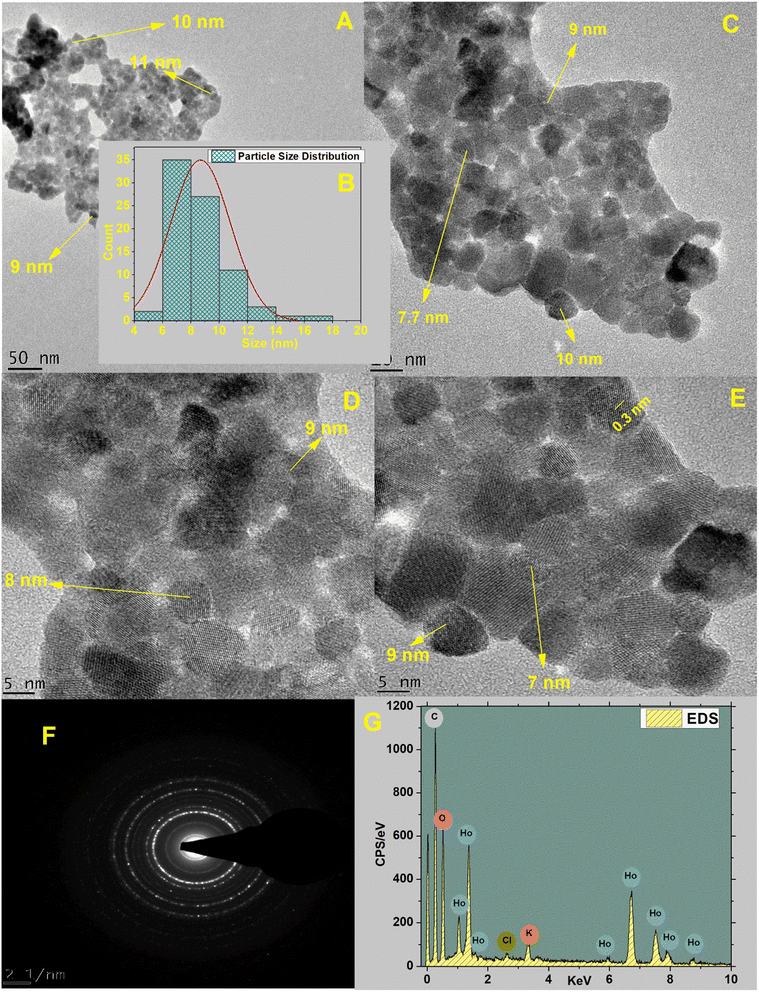 |
| Fig. 4 Various characterization techniques applied on HT-Ho2O3 NPs; (A; C; D; E) HRTEM images acquired at changed magnifications; (B) particle size distribution; (F) selected area electron diffraction pattern; (G) EDS spectrum. | |
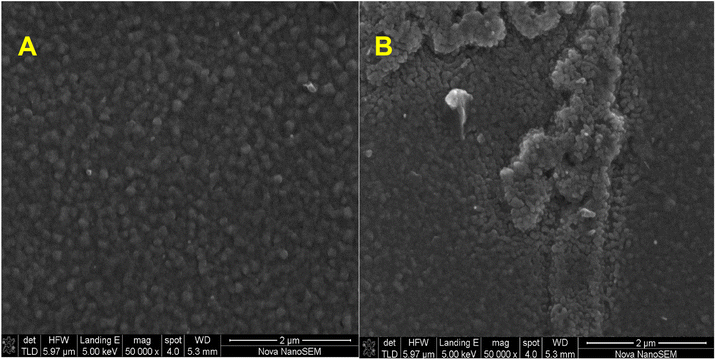 |
| Fig. 5 (A) and (B) SEM images of the HT-Ho2O3 NPs. | |
3.2. Biomedical potentials
3.2.1. In vitro antioxidant and cytotoxic properties. It was observed that the free radical potential of the HT-Ho2O3 NPs was dose responsive. Visual observation indicated slight changes in the purple color of the DPPH reagent, reflecting their moderate free radical scavenging potential. From the absorbance values the percentage radical scavenging were calculated. At different tested concentrations of 62.5, 125, 250, 500 and 1000 μg mL−1, the percent inhibition was 21 ± 1.37%, 27.43 ± 1.50%, 31.30 ± 1.17%, 36.31 ± 1.43% and 43.40 ± 0.96% respectively, as shown in Fig. 6A. An IC50 value of 1235.4 μg mL−1 was obtained which was significantly less as compared to the ascorbic acid (IC50: 24.9 μg mL−1). In the brine shrimp cytotoxicity assay, the different concentration HT-Ho2O3 NPs were tested on Artemia salina nauplii. HT-Ho2O3 NPs revealed a dose dependent cytotoxicity i.e., 17 ± 2.46%, 33.33 ± 3.05%, 43.33 ± 2.41%, 63.33 ± 1.27% and 76.67 ± 3.3% at concentration of 62.5, 125, 250, 500 and 1000 μg mL−1 respectively, as shown in Fig. 6A. The LC50 value of 320.4 μg mL−1 was obtained for HT-Ho2O3 NPs whereas LC50 value 98.02 μg mL−1 for the reference potassium dichromate.
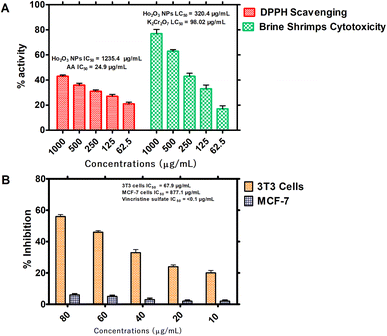 |
| Fig. 6 In vitro bioassays on the HT- Ho2O3 NPs; (A) percent free radical scavenging effect at different concentrations and their brine shrimp cytotoxicity potential; (B) the in vitro MTT cytotoxicity assay on 3T3 and MCF-7 cells. | |
3.2.2. Cell culture assays. Anticancer characteristics of HT-Ho2O3 NPs was established using MCF-7 and 3T3 cancer cell lines as indicated in Fig. 6B. It was observed that the HT-Ho2O3 NPs actively inhibited the 3T3 cells as compared to the MCF-7. At concentration 80 μg mL−1, the HT-Ho2O3 NPs revealed cytotoxicity of 56 ± 1.23% and 6.5 ± 0.80% for 3T3 and MCF-7 cancer cells. The IC50 value for MCF-7 cells was 877.1 μg mL−1 while the 3T3 cells show an IC50 value of 67.9 μg mL−1. Vincristine sulfate used as a positive control exhibited an IC50 value <0.1 μg mL−1. The results are presented in Fig. 6B.
3.2.3. Anti-angiogenic assay. Angiogenesis is a vital step in the development or growth of tumor, therefore, HT-Ho2O3 NPs were evaluated for anti-angiogenic potential by CAM assay. For this purpose, the reliable and inexpensive in ovo CAM assay was used for assessing the anti-angiogenic potential of HT-Ho2O3 NPs. The results are indicated in Fig. 7A–D. Across the tested concentration i.e., 62.5, 125, 250, 500 and 1000 μg mL−1 of the HT-Ho2O3, the percent inhibition was observed as 3.84 ± 1.05%, 16.96 ± 1.81%, 21.88 ± 1.37%, 27.15 ± 1.92% and 34.41 ± 2.09% respectively. The IC50 value calculated for HT-Ho2O3 NPs was 3125.9 μg mL−1 while the positive control dexamethasone exhibited an IC50 value of 17.41 μg mL−1.
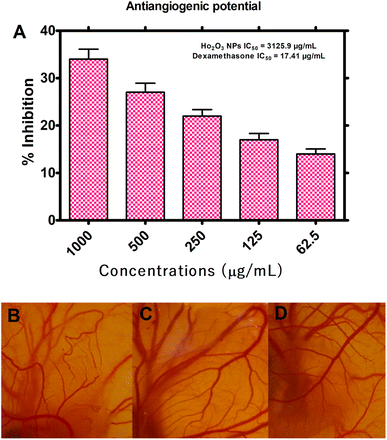 |
| Fig. 7 Antiangiogenic potential of the HT-Ho2O3 NPs (A) percent inhibition of angiogenesis in ova at different concentrations; (B–D) selected photos of the in ovo CAM bioassay at different concentrations. | |
3.2.4. Antidiabetic potential. The antidiabetic potential of the HT-Ho2O3 NPs was examined through in vitro α-amylase and α-glucosidase inhibition as indicated in Fig. 8. At different tested concentration i.e., 1000, 500, 250, 125, and 62.5 μg mL−1, the percent α-glucosidase inhibition of 61.32 ± 1.61%, 50.94 ± 1.27%, 40.57 ± 1.19%, 27.99 ± 1.44% and 20.13 ± 1.37% was observed respectively. The IC50 value of the nanoparticles was determined to be 467.1 μg mL−1 while the positive reference acarbose exhibited an IC50 value of 20.4 μg mL−1 in this assay. Similarly, on the same doses, the α-amylase inhibition was observed to be 54.70 ± 1.05%, 44.34 ± 1.14%, 28.19 ± 1.10%, 16.87 ± 1.37% and 13.01 ± 0.91%. The IC50 value in the α-amylase inhibition assay was 741 μg mL−1 for the HT-Ho2O3 NPs and 36.6 μg mL−1 for acarbose which was used as positive control.
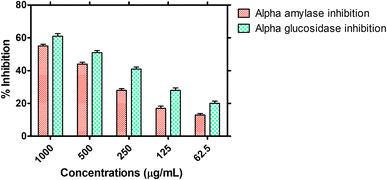 |
| Fig. 8 Antidiabetic potential of the HT-Ho2O3 NPs. | |
4. Discussion
Bio-fabrication of the nanomaterials owes several advantages over other physical and chemical means. These includes their sustainable nature, easiness of the process and enhanced compatibility. Plants constitute of diverse secondary metabolites that possesses different pharmacological properties and also has potential to reduce bulk metals into their ions. Such bioactive molecules act as green reducing and stabilizing agents.42–44 The therapeutic potential of the fruits of H. thebaica is well established. Previously, we have reported H. thebaica mediated synthesis of Fe2O3 (ref. 22), CeO2 (ref. 21), Cr2O3 (ref. 23), BiVO4,45 ZnO,46 CuO,47 Er2O3 (ref. 48) and Ag nanoparticles 20. The biosynthesis of holmium oxide nanoparticles is reported for the 1st time. The plant H. thebaica possesses a rich phytochemistry which constitutes different organic acids and other components like naringin, quercetin, glycosides, tannins, saponins etc. are also reported in the H. thebaica.49,50 These plant constituents have the capacity to catalyze redox reactions and stabilize the nanoparticles.51 XRD pattern was consistent with previous reports.52 Similarly, the FTIR spectrum revealed a characteristic metal oxide vibration at ∼603 cm−1. “Eg” was calculated as 5.1 eV which is in agreement with previous reports.2 The biomedical applications of the holmium oxide nanoparticles are not exclusively covered in the literature.
We have reported for the 1st time the anticancer and antidiabetic potential of biogenic HT-Ho2O3 NPs. The anticancer applications revealed that the nanoparticles exhibited toxicity against 3T3 cell lines while insignificant anticancer potential was observed on MCF-7 cell lines. The anticancer properties of holmium-based materials have been reported. For example, the holmium ferrite nanoparticles coated with cyclodextrin and polyethylene glycol loaded with camptothecin, an anticancer drug revealed anticancer potential 79.42% when tested at 250 μg mL−1.53 A similar nanocarrier system based on holmium ferrite was described for the potential treatment of human glioma cancer.54 The anticancer mechanism of the rare earth lanthanides is mostly ascribed to the oxidative stress generated by the reactive oxygen species, retarding the calcium transport, direct interaction with cellular organelle and DNA, endoplasmic reticulum stress, activating the mitogen-activated protein kinase (MAPK) etc.55–57 One of the key aspects of the cancer progression is the formation of new vasculature i.e., angiogenesis which facilitate the influx of nutrients to the cancer cells for keeping them alive.58 Keeping in view, we have tested the HT-Ho2O3 NPs through in ovo CAM assay which is routinely performed for the assessment of inhibition of angiogenesis. Our results clearly indicate that the HT-Ho2O3 NPs can have applications in the inhibition of angiogenesis.
Furthermore, we have evaluated the antidiabetic potential of the as fabricated HT-Ho2O3 NPs. One of the popular strategies to control postprandial levels of glucose in the body relates to the inhibition of the enzymes that actively breakdown the carbohydrates into glucose and raise their levels in the body. Hence, the diabetic treatment research revolves around the effective α-amylase and α-glucosidase inhibitors. These enzymes tends to facilitate the breakdown of complex carbohydrates to glucose and their inhibition reduces glucose absorption in the intestine.59 We have shown that the HT-Ho2O3 NPs possesses the ability to inhibit the α-amylase and α-glucosidase enzymes.
The chemical and physical means of synthesis of the nanoparticles possess disadvantages like generating hazardous waste and being expensive, whereas plant extracts-based synthesis is embodying the principals of green chemistry provides a cost effective and green alternative for the synthesis of the metal nanoparticles. Among biological resources, plants are mostly preferred for the biosynthesis of nanoparticles as they have very simple requirements as compared to other biological resources such as microorganisms which possess expensive requirements such as maintenance of the sterile conditions, culture media and other chemicals.60 The phytochemical stabilization of the nanoparticles is generally considered to improve the various pharmacognostic properties of the nanoparticles. It has been well reported that phytochemically stabilized nanoparticles shows enhanced biological and physicochemical properties as compared to the nanoparticles synthesized from other routes. Previously, nano-sulfur synthesized using the extracts of Punica granatum revealed elevated anticancer potential as compared to the chemically synthesized nano-sulfur.61 Similarly, in a comparative study of the chemogenic and biogenic titanium oxide nanoparticles, the biogenic titanium oxide nanoparticles revealed enhanced antibacterial properties as compared to the chemogenic.62 Enhancement in the physical properties using biogenic synthesis has also been reported. The green synthesized silicon oxide nanoparticles using Rhus coriaria are reported to have enhanced stability as compared to the chemically synthesized silicon oxide nanoparticles.63 The enhanced properties of the biogenic nanoparticles are attributed to their phytochemical stabilization which enhance surface functionalization properties and increase the physicochemical interactions.64 In addition, the phytochemical stabilization usually includes the phenols, flavonoids and other secondary metabolites that impart differential characteristics to the biogenic nanoparticles and enhances their efficacy.
5. Conclusion
In this work, the holmium oxide nanoparticles were bio-fabricated using a cost effective and green process that constituted the application of the aqueous extracts of H. thebaica. The nanoparticles were in the range of 6–10 nm, highly crystalline and with spherical to cuboidal morphologies. The optical bandgap (Eg) which was calculated to be 5.1 eV with major emission peaks at 415 nm and 607 nm obtained by PL spectrum. The biological response in different bioassays was dose dependent. The anticancer activities revealed that the holmium oxide was more effective against 3T3 cell lines as compared to the MCF-7. Furthermore, the nanoparticles were able to inhibit the formation of new vasculature during the in ovo CAM assay. Moderate antioxidant properties were observed. The antidiabetic potential of the holmium oxide nanoparticles revealed inhibitory potential against the enzymes involved in postprandial carbohydrate metabolism. Our findings suggest that the biological synthesis of holmium oxide nanoparticles is a suitable alternative to chemical and physical synthesis methods. Moreover, new avenues regarding the biomedical potential of the nano holmium oxide are revealed. We recommend further research on the synthesis and application of holmium oxide especially from the toxicity and nanomedicine perspective.
Data availability
The datasets used and/or analysed during the current study available from the corresponding author on reasonable request.
Conflicts of interest
Authors declare to have no conflict of interest.
Acknowledgements
Authors acknowledges partial financial support of HEC Pakistan (Award No. 9452/KPK/NRPU/R&D/HEC/2017). We also acknowledge the support from the UNESCO UNISA Africa Chair in Nanosciences and NANOAFNET.
References
- L. S. de la Rosa, M. C. Portillo, M. Mora-Ramirez, V. C. Téllez, M. P. Castillo, H. J. Santiesteban, A. C. Santiago and O. P. Moreno, Optik, 2020, 216, 164875 CrossRef CAS.
- S. Mortazavi-Derazkola, S. Zinatloo-Ajabshir and M. Salavati-Niasari, Ceram. Int., 2015, 41, 9593–9601 CrossRef CAS.
- H. E. A. Mohamed, A. T. Khalil, K. Hkiri, M. Ayaz, A. Usman, A. Sadiq, F. Ullah, M. A. Khan, A. Islam and M. Ovais, Appl. Organomet. Chem., 2023, 37, e7091 CrossRef CAS.
- G. Hussein, B. Balboul and G. Mekhemer, J. Anal. Appl. Pyrolysis, 2000, 56, 263–272 CrossRef CAS.
- B. M. Abu-Zied and A. M. Asiri, J. Rare Earths, 2019, 37, 185–192 CrossRef CAS.
- J. Kim, Z.-X. Luo, Y. Wu, X. Lu and M. Jay, Carbon, 2017, 117, 92–99 CrossRef CAS PubMed.
- S. Zinatloo-Ajabshir, S. Mortazavi-Derazkola and M. Salavati-Niasari, Int. J. Hydrogen Energy, 2017, 42, 15178–15188 CrossRef CAS.
- S. Zinatloo-Ajabshir, S. Mortazavi-Derazkola and M. Salavati-Niasari, Ultrason. Sonochem., 2017, 39, 452–460 CrossRef CAS PubMed.
- S. Mortazavi-Derazkola, S. Zinatloo-Ajabshir and M. Salavati-Niasari, J. Mater. Sci.: Mater. Electron., 2017, 28, 1914–1924 CrossRef CAS.
- P. Gastaldi, E. El-Khoury, M. Haddad, E. Mille, A. Dariel, T. Merrot and A. Faure, J. Pediatr. Urol., 2022, 18, 367 Search PubMed.
- C. Cerqueira-Coutinho, L. P. Vidal, S. R. Pinto and R. Santos-Oliveira, Appl. Radiat. Isot., 2016, 112, 27–30 CrossRef CAS PubMed.
- M. Abdusalyamova, F. Makhmudov, E. Shairmardanov, I. Kovalev, P. Fursikov, I. Khodos and Y. Shulga, J. Alloys Compd., 2014, 601, 31–37 CrossRef CAS.
- B. A. Balboul, Powder Technol., 2000, 107, 168–174 CrossRef CAS.
- L. M. d'Assuncao, I. Giolito and M. Ionashiro, Thermochim. Acta, 1989, 137, 319–330 CrossRef.
- V. K. Chaturvedi, B. Sharma, A. D. Tripathi, D. P. Yadav, K. R. Singh, J. Singh and R. P. Singh, Front. Med. Technol., 2023, 5 Search PubMed.
- A. J. Kora and R. B. Sashidhar, J. Antibiot., 2015, 68, 88–97 CrossRef CAS PubMed.
- A. T. Khalil, J. Iqbal, A. Shah, M. Z. Haque, I. Khan, M. Ayaz, I. Ahmad, S. Tasneem and H. Shah, 2021.
- A. Shah, K. Ahmad, A. T. Khalil, F. Amin, G. Lutfullah, K. Khan, G. Shah and A. Ahmad, Mater. Res. Express, 2019, 6, 125416 CrossRef CAS.
- H. E. A. Mohamed, S. Afridi, A. T. Khalil, D. Zia, Z. K. Shinwari, M. S. Dhlamini and M. Maaza, J. Inorg. Organomet. Polym. Mater., 2020, 30, 3241–3254 CrossRef CAS.
- H. E. A. Mohamed, S. Afridi, A. T. Khalil, D. Zia, J. Iqbal, I. Ullah, Z. K. Shinwari and M. Maaza, Mater. Res. Express, 2019, 6, 1050c1059 Search PubMed.
- H. E. A. Mohamed, S. Afridi, A. T. Khalil, M. Ali, T. Zohra, R. Akhtar, A. Ikram, Z. K. Shinwari and M. Maaza, Nanomedicine, 2020, 15, 467–488 CrossRef CAS PubMed.
- H. E. A. Mohamed, S. Afridi, A. T. Khalil, M. Ali, T. Zohra, M. Salman, A. Ikram, Z. K. Shinwari and M. Maaza, Mater. Sci. Eng., C, 2020, 112, 110890 CrossRef CAS PubMed.
- H. E. Ahmed Mohamed, S. Afridi, A. T. Khalil, T. Zohra, M. Ali, M. M. Alam, A. Ikram, Z. K. Shinwari and M. Maaza, Nanomedicine, 2020, 15, 1653–1669 CrossRef CAS PubMed.
- H. E. A. Mohamed, S. Afridi, A. T. Khalil, T. Zohra, M. M. Alam, A. Ikram, Z. K. Shinwari and M. Maaza, AMB Express, 2019, 9, 1–14 CrossRef CAS PubMed.
- M. Q. Nasar, M. Shah, A. T. Khalil, M. Q. Kakar, M. Ayaz, A. S. Dablool and Z. K. Shinwari, Inorg. Chem. Commun., 2022, 137, 109252 CrossRef CAS.
- A. Sani, D. Hassan, A. T. Khalil, A. Mughal, A. El-Mallul, M. Ayaz, Z. Yessimbekov, Z. K. Shinwari and M. Maaza, J. Biomol. Struct. Dyn., 2021, 39, 4133–4147 CrossRef CAS PubMed.
- A. T. Khalil, M. Ayaz, M. Ovais, A. Wadood, M. Ali, Z. K. Shinwari and M. Maaza, Inorg. Nano-Met. Chem., 2018, 48, 441–448 CrossRef CAS.
- A. K. Zak, W. A. Majid, M. E. Abrishami and R. Yousefi, Solid State Sci., 2011, 13, 251–256 CrossRef.
- P. Bindu and S. Thomas, J. Theor. Appl. Phys., 2014, 8, 123–134 CrossRef.
- S. M. Shah, M. Ayaz, A.-u. Khan, F. Ullah, K. Farhan, A.-u.-H. A. Shah, H. Iqbal and S. Hussain, Toxicol. Ind. Health, 2015, 31, 1037–1043 CrossRef CAS PubMed.
- A. T. Khalil, M. D. Khan, S. Razzaque, S. Afridi, I. Ullah, J. Iqbal, S. Tasneem, A. Shah, Z. K. Shinwari and N. Revaprasadu, Appl. Nanosci., 2021, 11, 2489–2502 CrossRef CAS.
- M. Ghufran, A. U. Rehman, M. Ayaz, Z. Ul-Haq, R. Uddin, S. S. Azam and A. Wadood, J. Biomol. Struct. Dyn., 2022, 1–15 Search PubMed.
- S. Ahmad, F. Ullah, M. Ayaz, A. Zeb, F. Ullah and A. Sadiq, Biol. Res., 2016, 49, 1–9 CrossRef PubMed.
- T. Kanwal, M. Kawish, R. Maharjan, I. Ghaffar, H. S. Ali, M. Imran, S. Perveen, S. Saifullah, S. U. Simjee and M. R. Shah, J. Mol. Liq., 2019, 289, 111098 CrossRef CAS.
- H. Rehman, W. Ali, M. Ali, N. Z. Khan, M. Aasim, A. A. Khan, T. Khan, M. Ali, A. Ali and M. Ayaz, PLoS One, 2023, 18, e0280553 CrossRef CAS PubMed.
- F. Hussain, Z. Khan, M. S. Jan, S. Ahmad, A. Ahmad, U. Rashid, F. Ullah, M. Ayaz and A. Sadiq, Bioorg. Chem., 2019, 91, 103128 CrossRef CAS PubMed.
- M. H. Mahnashi, Y. S. Alqahtani, B. A. Alyami, A. O. Alqarni, S. A. Alqahl, F. Ullah, A. Sadiq, A. Zeb, M. Ghufran and A. Kuraev, BMC Complementary Med. Ther., 2022, 22, 26 CrossRef CAS PubMed.
- S. Manjunatha, T. Machappa, Y. Ravikiran, B. Chethan and M. Revanasiddappa, Appl. Phys. A: Mater. Sci. Process., 2019, 125, 1–10 CrossRef CAS.
- Y. Jinqiu, C. Lei, H. Huaqiang, Y. Shihong, H. Yunsheng and W. Hao, J. Rare Earths, 2014, 32, 1–4 CrossRef.
- N. Dilawar, S. Mehrotra, D. Varandani, B. Kumaraswamy, S. Haldar and A. Bandyopadhyay, Mater. Charact., 2008, 59, 462–467 CrossRef CAS.
- T. Wiktorczyk, Thin Solid Films, 2002, 405, 238–242 CrossRef CAS.
- S. R. Alizadeh, M. Seyedabadi, M. Montazeri, B. A. Khan and M. A. Ebrahimzadeh, Mater. Chem. Phys., 2023, 296, 127240 CrossRef CAS.
- E. R. Camelo, J. D. S. Castro and C. F. das Virgens, J. Therm. Anal. Calorim., 2023, 148, 49–62 CrossRef CAS.
- L. Wu, G. Huang, T. Xie, A. Zhang and Y. Fu, Colloids Surf., A, 2023, 658, 130773 CrossRef CAS.
- H. E. A. Mohamed, S. Afridi, A. T. Khalil, T. Zohra, M. M. Alam, A. Ikram, Z. K. Shinwari and M. Maaza, AMB Express, 2019, 9, 1–14 CrossRef CAS PubMed.
- H. E. A. Mohamed, S. Afridi, A. T. Khalil, D. Zia, Z. K. Shinwari, M. S. Dhlamini and M. Maaza, J. Inorg. Organomet. Polym. Mater., 2020, 30, 3241–3254 CrossRef CAS.
- H. Mohamed, T. Thema and M. Dhlamini, Mater. Today: Proc., 2021, 36, 591–594 CrossRef CAS.
- H. E. A. Mohamed, A. T. Khalil, K. Hkiri, M. Ayaz, J. A. Abbasi, A. Sadiq, F. Ullah, A. Nawaz, I. Ullah and M. Maaza, AMB Express, 2023, 13, 24 CrossRef CAS PubMed.
- A. N. A. Rahman, M. A. AbdelMageed, M. E. M. Assayed, H. S. A.-R. Gharib, M. A. Nasr, G. E. Elshopakey, H. A. Moniem, S. E. Shahin, E. ELHusseiny and S. A. Ahmed, Aquaculture, 2023, 564, 739058 CrossRef.
- F. R. Saber, S. H. Aly, M. A. Khallaf, H. A. El-Nashar, N. M. Fahmy, M. El-Shazly, R. Radha, S. Prakash, M. Kumar and D. Taha, Food Anal. Methods, 2022, 1–21 Search PubMed.
- A. Pridyantari, A. Ningrum, W. Handayani and C. Imawan, 2020.
- K. Shinde, W. Nan, M. Tien, H. Lin, H.-R. Park, S.-C. Yu, K. Chung and D.-H. Kim, J. Magn. Magn. Mater., 2020, 500, 166391 CrossRef CAS.
- K. Kaliyamoorthi, S. Ramasamy, A. S. Pillai, A. Alexander, A. Arivarasu and I. V. Enoch, Mater. Lett., 2021, 285, 129164 CrossRef CAS.
- Y. Zeng, H. Chen, F. Yang, H. Li and P. Yang, Mater. Res. Express, 2022, 9, 115011 CrossRef.
- N. S. Chundawat, S. Jadoun, P. Zarrintaj and N. P. S. Chauhan, Polyhedron, 2021, 207, 115387 CrossRef CAS.
- M. Ghufran, A. U. Rehman, M. Shah, M. Ayaz, H. L. Ng and A. Wadood, J. Biomol. Struct. Dyn., 2020, 38, 5488–5499 CrossRef CAS PubMed.
- M. Ghufran, H. A. Khan, M. Ullah, S. Ghufran, M. Ayaz, M. Siddiq, S. S. u. Hassan and S. Bungau, Cancers, 2022, 14, 4884 CrossRef CAS PubMed.
- M. H. Mahnashi, Y. S. Alqahtani, B. A. Alyami, A. O. Alqarni, F. Ullah, A. Wadood, A. Sadiq, A. Shareef and M. Ayaz, BMC Complementary Med. Ther., 2021, 21, 1–14 CrossRef PubMed.
- H. Gin and V. Rigalleau, Diabetes Metab., 2000, 26, 265–272 CAS.
- N. S. Hamed, E. F. Taha and S. Khateeb, Cell Biochem. Funct., 2023, 1–18 Search PubMed.
- S. Krishnappa, C. M. Naganna, H. K. Rajan, S. Rajashekarappa and H. B. Gowdru, ACS Omega, 2021, 6, 32548–32562 CrossRef CAS PubMed.
- S. M. Hunagund, V. R. Desai, J. S. Kadadevarmath, D. A. Barretto, S. Vootla and A. H. Sidarai, RSC Adv., 2016, 6, 97438–97444 RSC.
- C. Y. Rahimzadeh, A. A. Barzinjy, A. S. Mohammed and S. M. Hamad, PLoS One, 2022, 17, e0268184 CrossRef CAS PubMed.
- N. T. T. Nguyen, L. M. Nguyen, T. T. T. Nguyen, T. T. Nguyen, D. T. C. Nguyen and T. V. Tran, Environ. Chem. Lett., 2022, 20, 2531–2571 CrossRef CAS PubMed.
|
This journal is © The Royal Society of Chemistry 2023 |