DOI:
10.1039/D3RA04901A
(Paper)
RSC Adv., 2023,
13, 26302-26312
Tetranuclear lanthanide-based silsesquioxanes: towards a combination of a slow relaxation of the magnetization and a luminescent thermometry†
Received
20th July 2023
, Accepted 28th August 2023
First published on 4th September 2023
Abstract
Lanthanide-based silsesquioxanes constitute an emerging family of cage-like metallasilsesquioxanes with exciting optical and magnetic properties. We report here the synthesis, structures and luminescence properties of a series of tetranuclear lanthanide-silsesquioxane compounds of general formula [NEt4]2[(Ph4Si4O8)2(Ln/Ln′)4(NO3)6(EtOH)2(MeCN)2]·4(MeCN) with different lanthanide ions (where Ln/Ln′ = Dy3+/Eu3+ (1), Dy3+/Tb3+ (2) and Eu3+/Tb3+/Y3+ (3)) and investigate the impact of the lanthanide ions combination on magnetic and photo-luminescent properties. Compound 1 behaves as a field-induced Single Molecule Magnet (SMM) and presents temperature-dependent luminescence characteristics of Eu3+ making it an emissive thermometer working in the temperature range 293–373 K with the maximum relative sensitivity of 1.15% K−1 achieved at 293 K. Compounds 2 and 3 are paramagnets, which demonstrate a characteristic photoluminescence with Dy3+ to Tb3+ and Tb3+ to Eu3+ energy transfers, respectively.
Introduction
Coordination compounds made by an association of Ln3+ ions with different ligands attracted increasing interest in the recent decade due to both their magnetic and luminescent properties exciting not only from a fundament point of view, but also due to promising technological applications. Indeed, an important breakthrough in the field of molecular magnetism has been made by a recent discovery of mononuclear lanthanide complexes, such as dysprosium metallocenes, exhibiting Single-Molecule Magnetic (SMM) behaviour at temperatures near or even exceeding liquid nitrogen's boiling point relevant for their promising employment in future spintronic devices.1–4 On the other hand, a temperature-dependence of the lanthanide-characteristic luminescence brought a new paradigm on the use of lanthanide-based coordination complexes as a smart emissive temperature probe at micro- and nanoscales.5 They have great potential in a wide range of applications ranging from biology and medicine to cryogenics5–7 because they offer high accuracy remote temperature measurements with significant spatial and thermal resolutions, as well as with a high maximal relative sensitivity (Sr > 1% K−1).8–12 These two discoveries inspired an important development in designing numerous mono- and polynuclear lanthanide-based complexes with optimized magnetic13–18 or optical properties,19–21 which are usually observed separately. Important work has also been done for the combination of a SMM behaviour with luminescence to design multifunctional magneto-luminescent complexes, in which their optical properties have been employed to probe the magnetic relaxation mechanisms.22–25 Recently, an interesting strategy combining SMM behaviour with emissive thermometry has opened exciting perspectives in the temperature monitoring in future SMM-based devices at the molecular level.25 However, the examples of SMM exhibiting temperature dependent luminescence able to work as an efficient thermometer are very scarce. We can cite, for instance, some rare examples of Dy3+-,26 Yb3+-,27,28 Tb3+-,29 Nd3+-based complexes30 and a metallofullerene DyErScN@C80 (ref. 31) exhibiting a low temperature SMM behaviour and an emissive thermometry with, in a few rare cases, an overlap of both properties in the working temperature range.
Recently, we reported a promising family of luminescent and/or magnetic tetranuclear cage-like lanthanide-silsesquioxanes designed by a combination of lanthanide ions with the silsesquioxane repeating subunit (RSiO1.5)8 forming the robust inorganic Si–O–Si skeleton.32–34 We demonstrated that Dy3+ containing silsesquioxane of the formula (Et4N)2[(PhSiO1.5)8(Y0.75Dy0.25O1.5)4(O)(NO2.5)6(EtOH)2(MeCN)2] presented both, field-induced SMM behaviour and Dy3+ characteristic emission, while Tb3+ and Eu3+ analogues exhibit paramagnetism with a low temperature spin flip and luminescence. On the other hand, an anionic cage containing solid-state solution of mixed Tb3+/Eu3+ lanthanides demonstrated a tuneable thermosensitive Tb3+-to-Eu3+ energy transfer driven by the Tb3+ and Eu3+ emission, which permitted to propose it as an efficient temperature sensor with excellent linearity, repeatability and good thermal sensitivity.34 Note that these multifunctional lanthanide silsesquioxanes demonstrated several advantages in comparison to simple coordination complexes and hybrid materials, consisting in: (i) well definite but versatile crystal structures in which the nature of lanthanide ions and terminal ligands can be modified without impacting the structural organisation. This offers an important flexibility in designing luminescent and magnetic systems because emission and excitation wavelengths can be easily adjusted; (ii) lanthanide ions are integrated inside the cage-like rigid framework through the formation of covalent bonds with bridging oxygen atoms, which preclude any lanthanides' leaching; (iii) the presence of silsesquioxane moieties offers important chemical, photo- and thermal stabilities. Moreover, it may be considered as “lanthanide molecular cluster” with promising photo-luminescence properties.35 Remarkably, these compounds present an important stability to photobleaching at a relatively high working temperature (373 K) due to the presence of the siloxane matrix, which played a protective role. However, up to now, the combination of a SMM behaviour and the emissive thermometry has never been observed in the same silsesquioxane cage.
In the present work, we extend our approach to solid solutions of different lanthanide ions in the aims to design luminescent and magnetic cage-like silsesquioxanes with different emission colour and check their ability to work as emissive thermometers. Therefore, we report here the synthesis, crystal structures of tetranuclear lanthanide-based silsesquioxanes, [NEt4]2[(Ph4Si4O8)2(Ln/Ln′)4(NO3)6(EtOH)2(MeCN)2]·4(MeCN) where Ln/Ln′ = Dy3+/Eu3+ (1), Dy3+/Tb3+ (2), Eu3+/Tb3+/Y3+ (3) and investigate the contribution of these lanthanide ions on magnetic and photo-luminescent properties with a particular focus on an association of SMM properties and luminescent thermometry. The choice of Dy3+ ion in the couples Ln/Ln′ has been motivated by its promising ability to bring SMM behaviour due to its high magnetic anisotropy and high magnetic moment. We introduced alongside Dy3+ highly emissive Eu3+ or Tb3+ ions in order to combine a slow relaxation of the magnetization with a luminescence. Note also that the use of Y3+ permits the magnetic dilution. All of the obtained compounds present emission characteristic of constituent Ln3+ ions in solid state at room temperature. The cage-like silsesquioxane Dy3+/Eu3+ 1 presents a slow relaxation of the magnetization at low temperature making it a field induced SMM and a temperature dependent emission relevant of Eu3+ in the temperature range between 293 and 373 K. This latter characteristic allows it to operate as an emissive thermometer with the maximum relative sensitivity of 1.15% K−1 achieved at 293 K, which is comparable to other promising emissive thermometers. Note that 1 is the third example of Dy3+ based SMM exhibiting emissive thermometry properties. Compounds Dy3+/Tb3+ 2 and Tb3+/Eu3+ 3 are paramagnets, which exhibit a characteristic photoluminescence with Dy3+ to Tb3+ and Tb3+ to Eu3+ energy transfers, respectively, while their emission is not temperature dependent.
Experimental
General conditions
Phenyltrimethoxysilane (98%), Et4NCl (≥98%), Eu(NO3)3·6H2O (99.9% trace metals basis), Tb(NO3)3·6H2O (99.9% trace metals basis), Dy(NO3)3·6H2O (99.9% trace metals basis), Y(NO3)3·6H2O (99.9% trace metals basis), ethanol and acetonitrile were purchased from Merck and used as received.
Synthesis of [NEt4]2[(Ph4Si4O8)2(Ln/Ln′)4(NO3)6(EtOH)2(MeCN)2]·4(MeCN) Ln = Eu3+/Dy3+ 1, Dy3+/Tb3+ 2, Eu3+/Tb3+/Y3+ 3
The synthesis of compounds 1–3 has been performed in a similar way. A mixture of PhSi(OMe)3 (0.94 mmol) and NaOH (0.94 mmol) was dissolved in 30 mL of ethanol. The resulting solution was heated to reflux for 1.0 h. Afterwards the mixture of lanthanide salts (0.47 mmol in total, see amount of each lanthanide salt below) and Et4NCl (0.47 mmol) dissolved in 30 mL of CH3CN were added at once. The resulted mixture was heated to reflux for 3 h and the solution was filtered from the insoluble residue providing a colourless solution. Crystalline materials were obtained by slow evaporation of solvents in a period of a few days. The single crystals suitable for a single crystal X-ray diffraction were collected. The products were dried in vacuum to perform elemental analysis and to calculate the yield.
[NEt4]2[(Ph4Si4O8)2Dy0.87Eu3.12(NO3)6(EtOH)(MeCN)2]·4MeCN 1. Reactants loadings: PhSi(OMe)3 (0.186 g, 0.94 mmol), NaOH (0.038 g, 0.94 mmol), Eu(NO3)3·6H2O (0.16 g, 0.35 mmol), Dy(NO3)3·6H2O (0.05 g, 0.12 mmol), Et4NCl (0.08 g, 0.47 mmol). Yield = 33% (0.09 g).Anal. calcd (for the theoretical Eu3Dy-compound): % Dy, 6.92; Eu, 19.41; Si, 9.57. Found (for Eu3.12Dy0.87-compound): % Dy, 6.03; Eu, 20.21; Si, 9.58.
[NEt4]2[(Ph4Si4O8)2Dy3.12Tb0.88(NO3)6(EtOH)2(MeCN)2]·4MeCN 2. Reactants loadings: PhSi(OMe)3 (0.186 g, 0.94 mmol), NaOH (0.038 g, 0.94 mmol), Dy(NO3)3·6H2O (0.16 g, 0.35 mmol), Tb(NO3)3·6H2O (0.05 g, 0.12 mmol), Et4NCl (0.08 g, 0.47 mmol). Yield = 40% (0.11 g).Anal. calcd (for the theoretical Dy3Tb-compound): % Dy, 20.51; Si, 9.45; Tb, 6.69. Found (for the Dy3.12Tb0.88-compound): % Dy, 21.33; Si, 9.45; Tb, 5.88.
[NEt4]2[(Ph4Si4O8)2Eu0.87Tb1.01Y2.11(NO3)6(EtOH)2(MeCN)2]·2EtOH 3. Reactants loadings: PhSi(OMe)3 (0.186 g, 0.94 mmol), NaOH (0.038 g, 0.94 mmol), Y(NO3)3·6H2O (0.09 g, 0.23 mmol), Eu(NO3)3·6H2O (0.05 g, 0.12 mmol), Tb(NO3)3·6H2O (0.05 g, 0.12 mmol), Et4NCl (0.08 g, 0.47 mmol). Yield = 42% (0.11 g).Anal. calcd (for the theoretical EuTbY2-compound): % Eu, 6.85; Si, 10.13; Tb, 7.16; Y, 8.01. Found (for the Eu0.87Tb1.01Y2.11-compound): % Eu, 5.89; Si, 10.17; Tb, 7.26; Y, 8.49.
X-ray crystallography
X-ray diffraction data for 1–3 were collected on a four-circle Rigaku Synergy S diffractometer equipped with a HyPix6000HE detector (T = 100 K, λ(MoKα)-radiation, kappa geometry, shutterless φ and ω-scan mode). The data were integrated and corrected for absorption by the CrysAlisPro program.36 For details, see Table S1.† The structures were solved by a direct method and refined by full-matrix least squares technique on F2 with anisotropic displacement parameters for non-hydrogen atoms. All attempts to model and refine positions of the acetonitrile solvate molecules in 3 were unsuccessful. Therefore, their contribution to the total scattering pattern was removed by use of the utility SQUEEZE in PLATON15.37 The hydrogen atoms of the OH-groups were localized in the difference-Fourier maps and refined isotropically with fixed displacement parameters [Uiso(H) = 1.5Ueq(O)]. The other hydrogen atoms were placed in calculated positions and refined within riding model with fixed isotropic displacement parameters [Uiso(H) = 1.5Ueq(C) for the CH3-groups and 1.2Ueq(C) for the other groups]. All calculations were carried out using the SHELXTL program suite.38
Crystallographic data have been deposited with the Cambridge Crystallographic Data Centre, CCDC 2240602 (1), CCDC 2240603 (2), CCDC 2240604 (3).
Crystal structure determination was performed in the Department of Structural Studies of Zelinsky Institute of Organic Chemistry, Moscow, Russia.
Characterizations
IR spectra (KBr pellets) were recorded using PerkinElmer Spectrum Two FT-IR Spectrometer. Compounds exhibit the similar set of signals: 1600–1400 cm−1 (νC
C, νC
N) 1130 cm−1 (νPh–Si), 945–1060 cm−1 (νasSi–O, νasSi–O–Si), 750–680 cm−1 (σC–H of mono-substituted phenyl group). The quantifications of lanthanides and Si elements were performed by using scanning electron microscope and energy dispersive X-ray analysis (SEM-EDX) on a FEI Quanta FEG 200 instrument. The powders were deposited on an adhesive carbon film and analysed under vacuum. The quantification of the heavy elements was carried out with the INCA software, with a dwell time of 3 μs.
The emission and excitation spectra were evaluated in solid state (powders) at room (298 K) and low (77 K) temperatures using a spectrofluorimeter Edinburgh FLS-920 by using a 450 W Xe arc lamp as the excitation source. The spectra were corrected for detection and optical spectral response of the spectrofluorimeter. The photoluminescent measurements as a function of temperature were done in solid state by using the temperature setup incorporated in the Edinburgh spectrofluorimeter. The emission spectra were recorded in the temperature range from 293 to 373 K. At each temperature step, a period of 2 min was given to allow the temperature to stabilize, and then 5 emission spectra were recorded with a dwell time of 0.3 s and a step of 1 nm. Magnetic susceptibility data were collected with a Quantum Design MPMS-XL SQUID magnetometer working between 1.8 and 350 K with the magnetic field up to 7 T. The sample was prepared in ambient condition. The data were corrected for the sample holder and the diamagnetic contributions calculated from the Pascal's constants.
Results and discussions
Synthesis and crystal structures
The synthetic strategy implies the formation of tetranuclear anionic cage-like silsesquioxanes [(Ph4Si4O8)2(Ln/Ln′)4(NO3)4(OH)(EtOH)3(H2O)]2− in association with a relatively bulky (NEt4)+ non-coordinating cation. A series of complexes [NEt4]2[(Ph4Si4O8)2(Ln/Ln′)4(NO3)6(EtOH)2(MeCN)2]·4(MeCN), where Ln/Ln′ = Dy3+/Eu3+ 1, Dy3+/Tb3+ 2, Eu3+/Tb3+/Y3+ 3, were synthesized by employing a two-step approach. First, the in situ formation of [PhSi(O)ONa]x moiety with their further reaction with Et4NCl salt and the corresponding Ln3+ salts. The SEM-EDX and the elemental analysis permitted to determine the composition of compounds 1–3.
Single crystals X-ray diffraction analysis performed on compounds 1–3 revealed that they are isostructural and crystallize in the P
space groups (Table S1, ESI†). Their crystal structures are similar to the previously published tetranuclear cage-like silsesquioxanes [Cat]2[(Ph4Si4O8)2(Ln)(NO3)6(EtOH)2(MeCN)2]·solv (where Ln = Tb, Eu and Dy).32–34 The crystal structure of 1 is described in details (Fig. 1) and the ones of 2 and 3 are shown in Fig. S1 and S2, ESI† and the crystallographic parameters are gathered in Tables S1 and S2, ESI.†
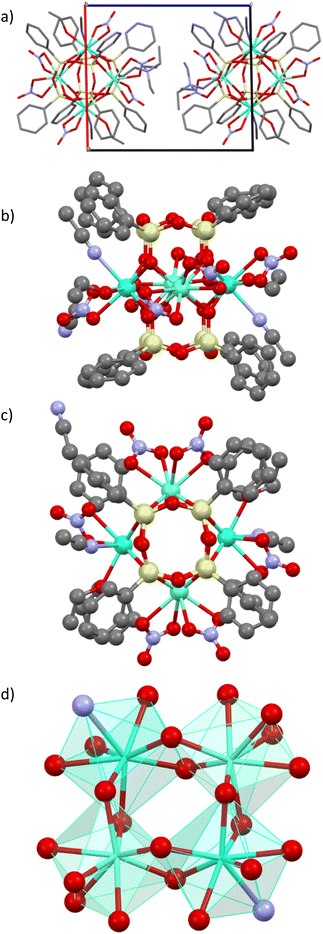 |
| Fig. 1 (a) Perspective view of the crystal packing for 1 along the crystallographic axis b; (b) and (c) molecular structure of 1 showing the prism-like polyhedron in the form of a new year paper lantern; (d) polyhedron of Dy/Eu atoms in the (Dy0.92/Eu3.08O8)O core. Colour code: light green Dy/Eu; light yellow Si; red O; blue N; grey C. Hydrogen atoms and crystallized acetonitrile molecules have been omitted for clarity. | |
The crystal packing of compound 1 may be described as an assembly of di-anionic tetranuclear lanthanide-germanoxane molecules along the c axis alternated with NEt4+ cations. The unit cell possesses two motives with two different units arranged perpendicular to each other (Fig. 1a). The shortest intermolecular Eu/Dy⋯Eu/Dy distance is equal to 12.628 Å. The molecular structure of 1 may be described as a prism made by eight Si atoms comprising the Dy0.87Eu3.12O8 core in the middle providing an appearance of a new year paper lantern to the molecule (Fig. 1b and c). In this lanthanide-based core, each Dy3+/Eu3+ ion is linked to two others through two bridging oxygen atoms to form a distorted square (Fig. 1d). There are two different eight-coordinated Dy/Eu sites, which adopt a distorted square antiprismatic geometry (Fig. 1d). In two of theme, lanthanide atoms are coordinated by four bridging oxygen atoms from the core and four oxygen atoms from two terminal bidentate nitrate ligands, while in two others, Dy/Eu is linked to four bridging oxygens, two terminal oxygens from one nitrate, one ethanol and one acetonitrile molecules. The (Dy/Eu)–O distances are in the range 2.310(9)–2.563(3) Å, while the (Dy/Eu)–N one is equal to 2.551(4) and 2.5514(10) Å. The O–(Dy/Eu)–O angles in the square are equal to 71.62 and 72.90°. (Table S2, ESI†). The intramolecular Dy/Eu⋯Dy/Eu distances in the square are equal to 3.724 and 3.773 Å, which indicates that lanthanides are relatively close.
Magnetic properties
The magnetic properties of compounds 1–3, were investigated with a SQUID MPMS3 magnetometer working between 1.8 and 310 K up to 7 T. The temperature dependences of the magnetic susceptibility performed in direct current (dc) mode were performed under an applied magnetic field of 1000 Oe. At room temperature, the χT values are in a relatively good agreement with the expected theoretical values (see Table S3, ESI†). The χT product decreases as the temperature decreases, which reflects the thermal depopulation of the mJ levels and/or the presence of antiferromagnetic interactions between the lanthanide ions (Fig. 2a).
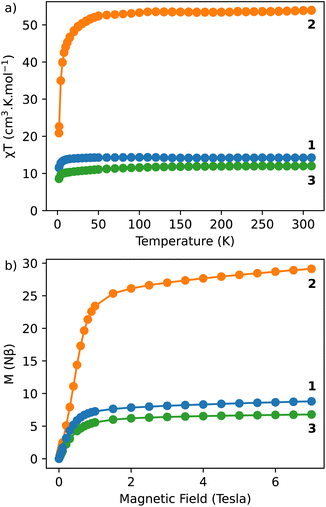 |
| Fig. 2 (a) χT vs. T curves performed under an applied magnetic field of 1000 Oe for 1–3; (b) magnetization vs. field curves obtained at 1.8 K for 1–3. | |
The field dependences of the magnetization performed at 1.8 K show a linear and a rapid increase of the magnetization in the low field region and then a slow increase after 1 tesla. But the saturation is not reached at 7 tesla, which indicates the presence of a significant magnetic anisotropy, as usually observed in lanthanide-based complexes (Fig. 2b).
The dynamic measurements in the ac mode have been performed for all compounds, but only sample 1 demonstrated a slow relaxation of the magnetization. This fact may be explained by the presence of magnetic interactions between Dy3+ in 2, which negatively affects the relaxation dynamics.18,33 For 1, the in-phase, χ′, and the out-of-phase, χ′′, components of the ac susceptibility does not present an important signal under a zero-dc field, which may be ascribed to the presence of the fast Quantum Tunnelling of the Magnetization (QTM). In order to avoid its influence, the frequency dependence of the ac susceptibility was measured under different applied dc fields, which shows an appearance of a signal for both components under an applied field (Fig. S3, ESI†). The temperature and the field dependences of the relaxation time can be fitted by using the eqn (1):39
|
 | (1) |
where the first, second, third and fourth terms represent the relaxation time for the direct, QTM, Orbach and Raman processes, respectively (
Fig. 3). To avoid the over parametrization, the
eqn (1) can be rewritten into
eqn (2):
|
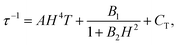 | (2) |
where
CT is a term corresponding to possible Orbach and Raman processes, which depends on temperature but does not depend on magnetic field. The obtained fit values are
CT = 672 ± 12 s
−1,
A = (6.71 ± 0.85) × 10
4 s
−1 T
−4 K
−1,
B1 = (3.05 ± 0.41) × 10
3 s
−1 and
B2 = (1.37 ± 0.32) × 10
4 T
−2. The fitting of the field dependence of the relaxation time with
eqn (2) permitted to determine the optimum magnetic field of 1000 Oe, which corresponds to the higher relaxation time (Fig. S3d
†).
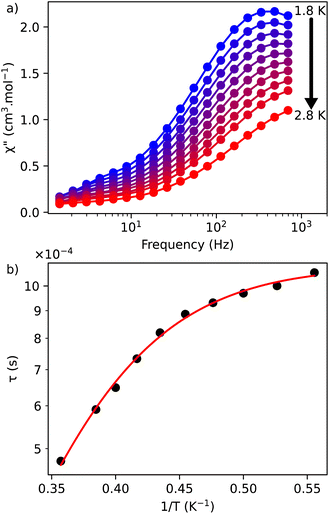 |
| Fig. 3 (a) Out-of-phase, χ′′, component of the ac magnetic susceptibility as a function of frequency at different temperatures measured under an optimal dc field of 1000 Oe for 1; (b) temperature dependence of the relaxation time for 1 under 1000 Oe. The red solid line represents the fits done by using the eqn (3). | |
The frequency dependence of the ac susceptibility under the optimal field of 1000 Oe reveals the presence of a series of peaks, for which the maxima shift towards higher frequencies as the temperature increases, suggesting the occurrence of a field-induced slow relaxation of the magnetization (Fig. 3a, S4a and b†). Eqn (3) can be rewritten from the eqn (1) to avoid the over parametrisation:
|
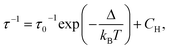 | (3) |
where
CH is a term which takes into account QTM and which depends on magnetic field but is constant as a function of temperature. Several combinations of the relaxation time as a function of the temperature were tested, and only the one corresponding to
eqn (3) was relevant. The obtained value of the effective energy barrier,
Δ, is equal to 11.3 ± 0.6 cm
−1 with
τ0 = (2.5 ± 0.8) × 10
−6 s. The obtained value of
CH is equal to 901 ± 22 s
−1 (
Fig. 3b). The analysis of the dynamic magnetic properties therefore indicates that compound
1 is a field-induced SMM in which the QTM process can be partially supressed by an application of the magnetic field.
Photoluminescence investigations
The photoluminescence of 1–3 was investigated in solid-state at room temperature (298 K). All compounds exhibit the typical Ln3+-characteristic emissions.
Luminescence of Dy3+/Eu3+ containing compound 1
The excitation spectrum of Dy3+/Eu3+ containing compound 1 were recorded by monitoring the main emission of Eu3+ at 612 nm (5D0 → 7F2) (Fig. 4a). A series of narrow lines appeared that can be attributed to transitions between 7F0 and different excited states indicating that Eu3+ is populated through a direct excitation into the intra-4f8 lines and that the antenna effect is not operational in this case. The excitation spectrum recorded with the main emission of Dy3+ expected at around 570 nm did not reveal the bands characteristic for this ion.
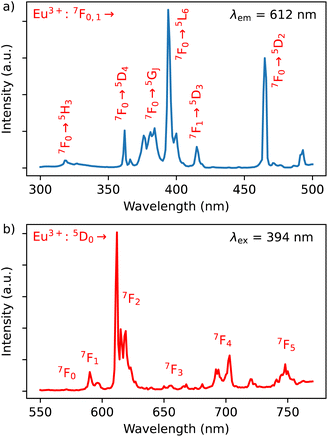 |
| Fig. 4 (a) Excitation spectrum of 1 monitored at λem = 612 nm (top) in solid state at room temperature; (b) emission spectrum of 1 performed with λex = 394 nm in solid state at room temperature. | |
The emission spectrum of 1 at room temperature recorded under excitation at 395 nm exhibit a series of classical Eu3+ 5D0 → 7F0–4 characteristic emission lines with the most intense red emission centred at 612 nm corresponding to 5D0 → 7F2 transition (Fig. 4b). The very weak 5D0 → 7F0 transition observed at 580 nm confirms the low symmetry of the coordination environment of the Eu3+ sites.40 The complicated profile of the 5D0 → 7F1 transition (several components) is in agreement with the presence of two europium sites in distorted square antiprismatic geometry. As expected for compounds with a relatively low symmetry of Eu sites, the main transition provides a red 5D0 → 7F2 emission. Moreover, since the transitions 5D0 → 7F2 and 5D0 → 7F4 are very sensitive to the coordination environment of the Eu site, their splitting in six or five components, respectively, is not surprising.40 Note that the direct excitation in the expected Dy3+ related transitions (for instance in the 6H15/2 → 4I5/2 transition expected at 450 nm) did not provide the appearance of the Dy3+ characteristic emission, which can be explained by the Eu/Dy ratio = 3.6 and high Eu3+ emission intensity in comparison with the Dy3+ ion.
In order to investigate the possibility to use Dy3+/Eu3+ compound 1 as an emissive thermometer, we adapted an optical thermometry approach involving the temperature dependence of the thermal population of low-lying levels of Eu3+ situated just above the ground level.41 The population of electronic configuration states follows the Boltzmann statistic:
|
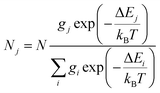 | (4) |
The partition function of the system is:
|
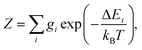 | (5) |
where Δ
Ei is the energy difference between the level
i and the ground level,
gi is the degeneracy of the level i. In the case of Eu
3+, the quantity of electronic configuration in the state
7F
J is defined as:
|
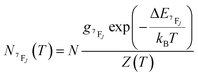 | (6) |
It depends on the partition function, the energy level, the degeneracy of the state and the temperature. The partition function is also temperature dependent. If electronic configurations are excited from the 7FJ state to the 5D0 state, then the luminescence intensity
will be proportional to 
|
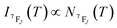 | (7) |
In the considered thermometry mechanism, we monitor the temperature profiles of the 5D0 → 7F4 emissions obtained by using two direct excitations, 7F1 → 5D0 and 7F2 → 5D0 (Fig. 5d). The luminescence intensity ratio (LIR) between these emissions can be written as:
|
 | (8) |
where
C is a constant, which depends on the degeneracy and Δ
E is the energy difference between
7F
1 and
7F
2.
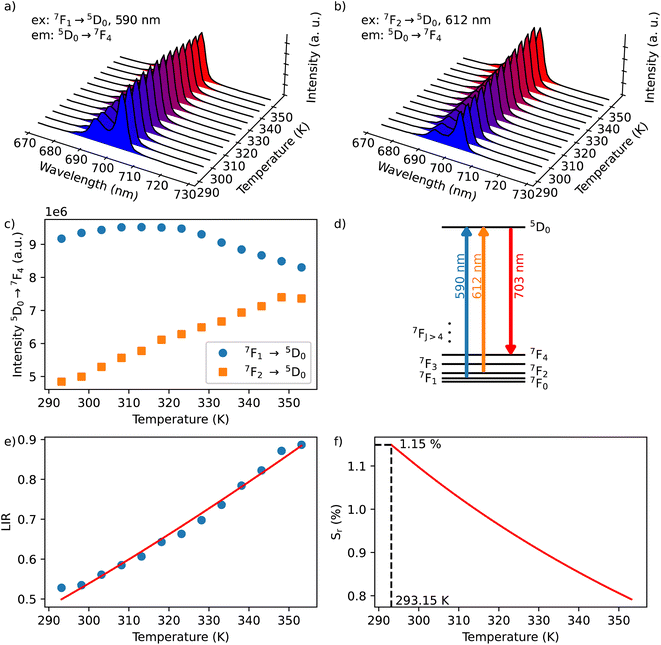 |
| Fig. 5 Emission spectra for 1 performed in the 293 to 373 K temperature range in solid state under excitation at λex = 590 nm (a) and at 612 nm (b); (c) temperature dependences of 5D0 → 7F4 transition upon 590 (7F1 → 5D0) and 612 nm (7F2 → 5D0) excitations; (d) simplified energy diagram showing transitions excited from 7F2,1 to 5D0 and corresponding emission originating from 5D0 to 7F4 used for thermometry measurements; (e) normalized luminescence intensity ratio (LIR) at 700 nm monitored under excitations at 612 and 590 nm (LIR, I700Ex612/I700Ex590) as a function of temperature (circles) and the associated fit (red curve); (f) temperature dependence of the thermal sensitivity (Sr) for 1 showing a maximum value of 1.15% K−1 at 293 K. | |
The emission spectra of 1 measured under excitations at 590 (7F1 → 5D0) and 612 nm (7F2 → 5D0) in the temperature range 293–353 K are shown in Fig. 5a and b and the corresponding luminescence intensities at 703 nm (transition 5D0 → 7F4) as a function of temperature is exhibited in Fig. 5c. As expected, the integrated intensity of the 5D0 → 7F4 transition (the integrated area is 670–730 nm) under excitation at 590 nm increases with temperature, then decreases, while the one under the excitation at 612 nm (the integrated area is 670–730 nm) increases constantly. The temperature dependence of their LIR(I1/I2) shown in Fig. 5e is fitted with eqn (8) providing the optimized parameters ΔE = 686 ± 24 cm−1 and C = 14.5 ± 1.5. Note that the former obtained value of ΔE is comparable to the expected one of 609 cm−1.
The relative thermal sensitivity (Sr) is the parameter allowing the comparison of thermometric performances among different types of thermometers.42 The Sr value represents the variation of the experimental parameter (LIR in the present case) per degree of temperature, expressed as:
|
Sr(T) = |∂LIR(T)/∂T|/LIR(T)
| (9) |
The temperature dependence of Sr is shown in Fig. 5f. The maximum Sr value is equal to 1.15% K−1 at 293 K. It is close to a frequently considered high relative thermal sensitivity (∼1% K−1),9 and in proximity with the best Sr values reported for mixed Eu3+/Tb3+ compounds.43
Luminescence of Tb3+/Dy3+ compound 2
The Dy3+ ion is a well-known sensitizer to activate Tb3+ ions in doped glasses because the 5D4 level of Tb3+ can be populated by the 4F9/2 level of Dy3+ through energy transfer.44–46 However, this phenomenon has been relatively scarcely exploited in molecular complexes. The excitation spectra of Tb3+/Dy3+ containing compound 2 was monitored at room temperature within: (a) the main 5D4 → 7F5 transition of Tb3+ at 543 nm, and (b) the 4F9/2 → 6H13/2 transition of Dy3+ at 572 nm. As shown in Fig. 6a, the excitation spectrum recorded under 543 nm emission wavelength of Tb3+ shows ten excitation bands among which six are assigned to Tb3+ (7F6 → 5H7, 5L7,8, 5H7, 5L9, 5L10, 5G6 shown in green colour) and four are attributed to Dy3+ ion (6H15/2 → 6P3/2, 4I13/2, 4G11/2, 4I5/2 shown in blue colour).32,33,44 The presence of excitation bands due to Dy3+ ion under the monitoring of the emission wavelength of Tb3+ indicates the presence of the Dy3+ to Tb3+ energy transfer. The excitation spectrum monitored at 572 nm (Dy3+ emission) exhibits similar transitions, while the intensities related to the Tb3+ transitions are lower, which could be explained by the back energy transfer (Fig. 6c).
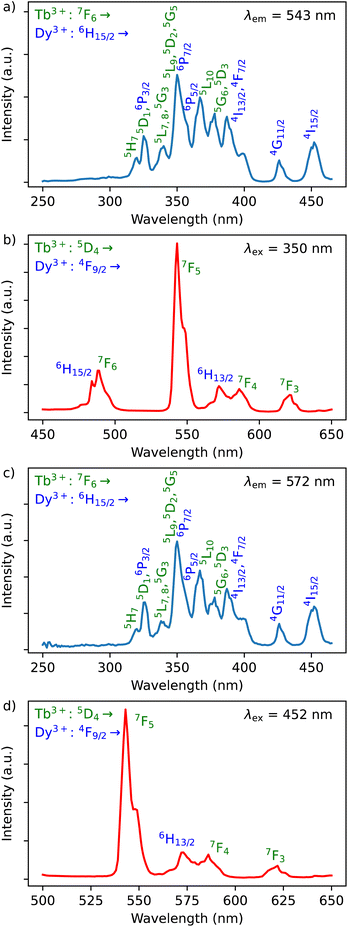 |
| Fig. 6 (a) Excitation spectrum of 2 monitored at λem = 543 nm (Tb3+ transition) in solid state at room temperature; (b) emission spectra of 2 performed with λex = 350 nm (Tb3+ transition) in solid state at room temperature; (c) excitation spectrum of 2 monitored at λem = 572 nm (Dy3+ transition) in solid state at room temperature; (d) emission spectra of 2 performed with λex = 452 nm (Dy3+ transition) in solid state at room temperature. The Tb3+ related transitions are notified in green, while Dy3+ ones are in blue. | |
The solid-state emission spectrum of 2 at room temperature recorded under excitation at the most intense band at 350 nm exhibits six emission bands among which four of them can be attributed to Tb3+ 5D4 → 7F6–3 characteristic transitions with the main 5D4 → 7F5 one centred at 543 nm and two of them correspond to Dy3+ transitions 4F9/2 → 6H15/2, 6H13/2 (Fig. 6b). In order to demonstrate the presence of the Dy3+ to Tb3+ energy transfer, the excitation was performed in the 6H15/2 → 4I5/2 Dy3+ transition at 452 nm. The emission spectrum demonstrating the presence of characteristic Dy3+ bands along with the Tb3+ transitions, which is dominated by a main band assigned to the 5D4 → 7F5 Tb3+ transition is shown in Fig. 6d. This signifies the energy transmission from Dy3+ to Tb3+ ions. Note that the intramolecular energy transfer between Dy3+ and Tb3+ has not been frequently demonstrated in coordination compounds of lanthanide ions, but its presence is not surprising considering that the shortest direct Ln3+–Ln3+ distance in 2 is equal to 3.648(2) Å. The corresponding simplified energy diagram showing the Dy3+ to Tb3+ energy transfer is shown Fig. 7. Note that compound 2 does not exhibit a temperature dependence of its emission allowing to use it for emissive thermometry.
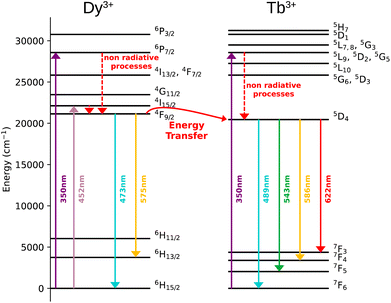 |
| Fig. 7 Simplified energy diagram showing the Dy3+ to Tb3+ energy transfer. | |
Luminescence of Y3+/Tb3+/Eu3+-compound 3
The excitation spectra of Tb3+/Eu3+/Y3+ – containing compound 3 by monitoring the main emission of Eu3+ at 612 nm (5D0 → 7F2) and the one of Tb3+ at 543 nm (5D4 → 7F5) with the assignment of different transitions characteristic of intra-4f transitions of Eu3+ and Tb3+ are shown in Fig. 8a and c. The observed transitions are coherent with previously published Tb3+/Eu3+-containing siloxane of similar structure.34 The maximum intensity of excitation for Eu3+ red emission is located at 394 nm, which corresponds to the Eu3+ transition 7F0 → 5L6. As for Tb3+ green emission, the main band is observed at 378 nm, which is attributed to the 7F6 → 5G6,5D3 transitions. The Tb3+-to-Eu3+ energy transfer is evidenced by the presence of the Tb3+ transition lines in the excitation spectra monitored in the Eu3+ emission (at 612 nm).
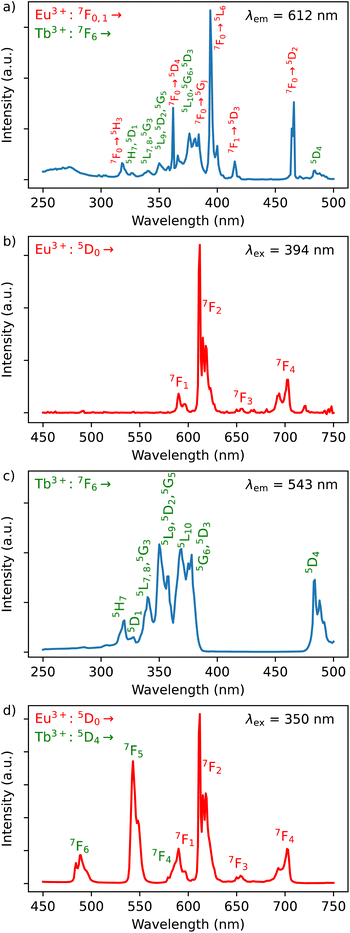 |
| Fig. 8 Solid state excitation spectra of 3 monitored at room temperature at λem = 612 nm (Eu3+ transition) (a) and at λem = 543 nm (Tb3+ transition) (c); solid state emission spectra of 3 performed at room temperature with λex = 394 nm (Eu3+ transition) (b) and at λex = 350 nm (Tb3+ transition) (d). The Tb3+ related transitions are notified in green, while Eu3+ ones are in red. | |
The emission spectra of 3 were measured under excitations at 394 and 350 nm (Fig. 8b and d). Upon an irradiation at 394 nm, the Eu3+ ion is selectively excited to the 5L6 energy level and non-radiative relaxations lead to the population of the 5D0 emitting state (Fig. 8d). The Eu3+ transitions are then observed from 5D0 to 7F0–4 manifold. Moreover, the excitation at 350 nm (Tb3+ band) leads to the main Eu3+ emission 5D0 → 7F0–4 along with the appearance of characteristic Tb3+ transitions from 5D4 to 7F2–6. This result clearly indicates the occurrence of an intramolecular Tb3+-to-Eu3+ energy transfer to the detriment of the Tb3+ emission considering the relatively short distances between lanthanides in the core (the shortest direct Ln3+–Ln3+ distance in 3 is 3.497 Å and the shortest Ln3+–Ln3+ distance through the bridging oxygen atom is 4.625 Å). The corresponding simplified energy diagram showing the Tb3+ to Eu3+energy transfer is shown Fig. 9. This observation is in agreement with the previously observed behaviour of Tb3+/Eu3+-containing siloxane.34 Note that unfortunately, compound 3 does not demonstrated a temperature dependence of its emission useful to establish emissive thermometer.
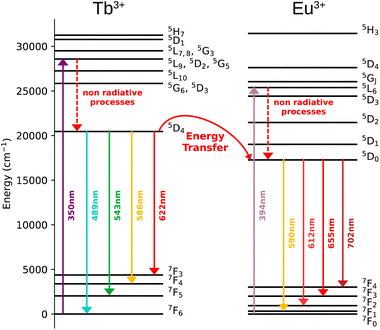 |
| Fig. 9 Simplified energy diagram showing the Tb3+ to Eu3+ energy transfer. | |
Conclusions
In summary, we reported a new series of anionic tetranuclear cage-like silsesquioxanes [NEt4]2[(Ph4Si4O8)2(Ln/Ln′)4(NO3)6(EtOH)2(MeCN)2]·4(MeCN) containing solid solutions of mixed lanthanide Ln/Ln′ ions Dy3+/Eu3+, Dy3+/Tb3+ and Eu3+/Tb3+/Y3+ investigating the impact of the lanthanide ions on the magnetic and optical properties. All compounds present prism-like molecular crystal structure where four lanthanide ions are linked through oxygen atoms by forming a core, which is located between two cyclic tetraphenylcyclotetrasiloxanolate moieties. The investigation of their magnetic properties reveals that Dy3+/Eu3+ compound 1 presents slow relaxation of the magnetisation under an applied optimal magnetic field of 1000 Oe to partially supress the QTM process, making it a field induced SMM. Note that Dy3+/Tb3+ compound 2 does not present the SMM behaviour certainly due to the dipolar interactions between Dy3+ ions, which negatively impact the relaxation dynamic. All compounds present lanthanide characteristic luminescence at room temperature. The Eu3+ emission of Dy3+/Eu3+ compound 1 has been used to establish an original self-referenced thermometry mechanism involving the temperature dependence of the thermal population of low-lying levels of Eu3+ situated just above the ground level. The obtained maximum Sr value is equal to 1.15% K−1 at 293 K. Therefore, this compound can be considered as a multifunctional field induced SMM and emissive thermometer with a relatively high relative thermal sensitivity (∼1% K−1).9 It represents the third example of Dy3+ SMM where slow relaxation of the magnetization is combined with luminescent thermometry in the same compound. Dy3+/Tb3+ and Y3+/Tb3+/Eu3+ compounds demonstrated a characteristic photoluminescence with the presence of the Dy3+ to Tb3+ and Tb3+ to Eu3+ energy transfers, respectively. These results point at significant potential in design of functional metallasesquioxanes47 including lanthanide centres.48–50
Conflicts of interest
There are no conflicts to declare.
Acknowledgements
This research (synthetic and photophysical studies) was funded by the Russian Science Foundation, grant number 22-13-00250. This work (elemental analyses) was in part supported by the Ministry of Science and Higher Education of the Russian Federation (Contract No. 075-03-2023-642) and was performed employing the equipment of Center for Molecular Composition Studies of INEOS RAS. J. L., S. S., G. F. and Y. G. thank the University of Montpellier and CNRS for financial support (project PRC2287 Premium 2019–2021 and IRP “Magnetic and luminescent complexes and cage molecules: from information storage at the molecular scale to nanothermometers”). J. L., S. S., G. F. and Y. G. are grateful to Platform of Analysis and Characterization (PAC) of ICGM for magnetic measurements. J. L. and A. N. K. are grateful for Vernadski program (Embassy of France in Russian Federation). This publication (structural studies) has been supported by the RUDN University Scientific Projects Grant System, project no. 0252392174.
Notes and references
- F.-S. Guo, B. M. Day, Y.-C. Chen, M.-L. Tong, A. Mansikkamäki and R. A. Layfield, Science, 2018, 362, 1400–1403 CrossRef CAS PubMed.
- K. R. McClain, C. A. Gould, K. Chakarawet, S. J. Teat, T. J. Groshens, J. R. Long and B. G. Harvey, Chem. Sci., 2018, 9, 8492–8503 RSC.
- C. A. P. Goodwin, F. Ortu, D. Reta, N. F. Chilton and D. P. Mills, Nature, 2017, 548, 439–442 CrossRef CAS PubMed.
- F.-S. Guo, B. M. Day, Y.-C. Chen, M.-L. Tong, A. Mansikkamäki and R. A. Layfield, Angew Chem. Int. Ed. Engl., 2017, 56, 11445–11449 CrossRef CAS.
- M. D. Dramićanin, J. Appl. Phys., 2020, 128, 040902 CrossRef.
- M. Bettinelli, L. Carlos and X. Liu, Phys. Today, 2015, 68, 38–44 CrossRef CAS.
- Y. Cui, H. Xu, Y. Yue, Z. Guo, J. Yu, Z. Chen, J. Gao, Y. Yang, G. Qian and B. Chen, J. Am. Chem. Soc., 2012, 134, 3979–3982 CrossRef CAS PubMed.
- C. D. S. Brites, A. Millán and L. D. Carlos, in Handbook on the Physics and Chemistry of Rare Earths, ed. B. Jean-Claude and K. P. Vitalij, Elsevier, 2016, vol. 49, pp. 339–427 Search PubMed.
- C. D. S. Brites, S. Balabhadra and L. D. Carlos, Adv. Opt. Mater., 2019, 7, 1801239 CrossRef.
- C. D. S. Brites, P. P. Lima, N. J. O. Silva, A. Millán, V. S. Amaral, F. Palacio and L. D. Carlos, Nanoscale, 2012, 4, 4799–4829 RSC.
- D. Jaque and F. Vetrone, Nanoscale, 2012, 4, 4301–4326 RSC.
- Luminescence Thermometry: Methods, Materials and Applications – 1st Edition, https://www.elsevier.com/books/luminescence-thermometry/dramicanin/978-0-08-102029-6, accessed June 13, 2022 Search PubMed.
- Y.-S. Ding, T. Han, Y.-Q. Zhai, D. Reta, N. F. Chilton, R. E. P. Winpenny and Y.-Z. Zheng, Chem.–Eur. J., 2020, 26, 5893–5902 CrossRef CAS.
- S. K. Gupta, T. Rajeshkumar, G. Rajaraman and R. Murugavel, Chem. Sci., 2016, 7, 5181–5191 RSC.
- J. Liu, Y.-C. Chen, J.-L. Liu, V. Vieru, L. Ungur, J.-H. Jia, L. F. Chibotaru, Y. Lan, W. Wernsdorfer, S. Gao, X.-M. Chen and M.-L. Tong, J. Am. Chem. Soc., 2016, 138, 5441–5450 CrossRef CAS PubMed.
- C. Wang, R. Sun, Y. Chen, B.-W. Wang, Z.-M. Wang and S. Gao, CCS Chem., 2020, 2, 362–368 CrossRef CAS.
- N. F. Chilton, C. A. P. Goodwin, D. P. Mills and R. E. P. Winpenny, Chem. Commun., 2014, 51, 101–103 RSC.
- D. N. Woodruff, R. E. P. Winpenny and R. A. Layfield, Chem. Rev., 2013, 113, 5110–5148 CrossRef CAS.
- M. Hasegawa, H. Ohmagari, H. Tanaka and K. Machida, J. Photochem. Photobiol., C, 2022, 50, 100484 CrossRef CAS.
- J.-C. G. Bünzli, Coord. Chem. Rev., 2015, 293–294, 19–47 CrossRef.
- G. F. de Sá, O. L. Malta, C. de Mello Donegá, A. M. Simas, R. L. Longo, P. A. Santa-Cruz and E. F. da Silva, Coord. Chem. Rev., 2000, 196, 165–195 CrossRef.
- J. Long, Y. Guari, R. A. S. Ferreira, L. D. Carlos and J. Larionova, Coord. Chem. Rev., 2018, 363, 57–70 CrossRef CAS.
- J.-H. Jia, Q.-W. Li, Y.-C. Chen, J.-L. Liu and M.-L. Tong, Coord. Chem. Rev., 2019, 378, 365–381 CrossRef CAS.
- F. Pointillart, O. Cador, B. Le Guennic and L. Ouahab, Coord. Chem. Rev., 2017, 346, 150–175 CrossRef CAS.
- R. Marin, G. Brunet and M. Murugesu, Angew. Chem., Int. Ed., 2021, 60, 1728–1746 CrossRef CAS PubMed.
- D. Errulat, R. Marin, D. A. Gálico, K. L. M. Harriman, A. Pialat, B. Gabidullin, F. Iikawa, O. D. D. Couto, J. O. Moilanen, E. Hemmer, F. A. Sigoli and M. Murugesu, ACS Cent. Sci., 2019, 5, 1187–1198 CrossRef CAS PubMed.
- G. Brunet, R. Marin, M.-J. Monk, U. Resch-Genger, D. A. Gálico, F. A. Sigoli, E. A. Suturina, E. Hemmer and M. Murugesu, Chem. Sci., 2019, 10, 6799–6808 RSC.
- K. Karachousos-Spiliotakopoulos, V. Tangoulis, N. Panagiotou, A. Tasiopoulos, E. Moreno-Pineda, W. Wernsdorfer, M. Schulze, A. M. P. Botas and L. D. Carlos, Dalton Trans., 2022, 51, 8208–8216 RSC.
- D. A. Gálico, R. Marin, G. Brunet, D. Errulat, E. Hemmer, F. A. Sigoli, J. O. Moilanen and M. Murugesu, Chem.–Eur. J., 2019, 25, 14625–14637 CrossRef PubMed.
- K. Kumar, D. Abe, K. Komori-Orisaku, O. Stefańczyk, K. Nakabayashi, J. R. Shakirova, S. P. Tunik and S. Ohkoshi, RSC Adv., 2019, 9, 23444–23449 RSC.
- M. Nie, J. Xiong, C. Zhao, H. Meng, K. Zhang, Y. Han, J. Li, B. Wang, L. Feng, C. Wang and T. Wang, Nano Res., 2019, 12, 1727–1731 CrossRef CAS.
- A. N. Kulakova, A. N. Bilyachenko, M. M. Levitsky, V. N. Khrustalev, E. S. Shubina, G. Felix, E. Mamontova, J. Long, Y. Guari and J. Larionova, Chem.–Eur. J., 2020, 26, 16594–16598 CrossRef CAS PubMed.
- A. N. Kulakova, K. Nigoghossian, G. Félix, V. N. Khrustalev, E. S. Shubina, J. Long, Y. Guari, L. D. Carlos, A. N. Bilyachenko and J. Larionova, Eur. J. Inorg. Chem., 2021, 2021, 2696–2701 CrossRef CAS.
- K. Nigoghossian, A. N. Kulakova, G. Félix, V. N. Khrustalev, E. S. Shubina, J. Long, Y. Guari, S. Sene, L. D. Carlos, A. N. Bilyachenko and J. Larionova, RSC Adv., 2021, 11, 34735–34741 RSC.
- D. A. Gálico, C. M. S. Calado and M. Murugesu, Chem. Sci., 2023, 14, 5827–5841 RSC.
- Rigaku Oxford Diffraction, CrysAlisPro, Version 1.171.41.106a Search PubMed.
- A. L. Spek, PLATON, A Multipurpose Crystallographic Tool, Utrecht University, The Netherlands, https://slideplayer.com/slide/8528251/, accessed February 8, 2023 Search PubMed.
- G. M. Sheldrick, Acta Crystallogr., Sect. C: Struct. Chem., 2015, 71, 3–8 Search PubMed.
- S. T. Liddle and J. van Slageren, Chem. Soc. Rev., 2015, 44, 6655–6669 RSC.
- K. Binnemans, Coord. Chem. Rev., 2015, 295, 1–45 CrossRef CAS.
- L. Zhao, J. Cai, F. Hu, X. Li, Z. Cao, X. Wei, Y. Chen, M. Yin and C.-K. Duan, RSC Adv., 2017, 7, 7198–7202 RSC.
- A. Bednarkiewicz, L. Marciniak, L. D. Carlos and D. Jaque, Nanoscale, 2020, 12, 14405–14421 RSC.
- V. Trannoy, A. N. Carneiro Neto, C. D. S. Brites, L. D. Carlos and H. Serier-Brault, Adv. Opt. Mater., 2021, 9, 2001938 CrossRef CAS.
- Ravita and A. S. Rao, J. Lumin., 2021, 239, 118325 CrossRef CAS.
- J. Juárez-Batalla, A. N. Meza-Rocha, H. G. Muñoz and U. Caldiño, Opt. Mater., 2017, 64, 33–39 CrossRef.
- M. Vijayakumar, K. Viswanathan and K. Marimuthu, J. Alloys Compd., 2018, 745, 306–318 CrossRef CAS.
- M. M. Levitsky, Y. V. Zubavichus, A. A. Korlyukov, V. N. Khrustalev, E. S. Shubina and A. N. Bilyachenko, J. Cluster Sci., 2019, 30, 1283–1316 CrossRef CAS.
-
(a) P. Wytrych, J. Utko, M. Stefanski, J. Kłak, T. Lis and Ł. John, Inorg. Chem., 2023, 62, 2913–2923 CrossRef CAS PubMed;
(b) G. Félix, A. Kulakova, S. Sene, C. Charlot, A. N. Bilyachenko, A. A. Korlyukov, A. D. Volodin, E. S. Shubina, I. G. Elizbarian, Y. Guari and J. Larionova, Organometallics, 2023 DOI:10.1021/acs.organomet.3c00009.
- K. Sheng, W.-D. Si, R. Wang, W.-Z. Wang, J. Dou, Z.-Y. Gao, L.-K. Wang, C.-H. Tung and D. Sun, Chem. Mater., 2022, 34, 4186–4194 CrossRef CAS.
- K. Sheng, R. Wang, A. Bilyachenko, V. Khrustalev, M. Jagodič, Z. Jagličić, Z. Li, L. Wang, C. Tung and D. Sun, ChemPhysMater, 2022, 1, 247–251 CrossRef.
Footnote |
† Electronic supplementary information (ESI) available: Additional structural and magnetic and ab initio calculations data. CCDC 2240602 (1), 2240603 (2), 2240604 (3). For ESI and crystallographic data in CIF or other electronic format see DOI: https://doi.org/10.1039/d3ra04901a |
|
This journal is © The Royal Society of Chemistry 2023 |