DOI:
10.1039/D3RA04646J
(Paper)
RSC Adv., 2023,
13, 31128-31140
Gemini ionic liquid-based surfactants: efficient synthesis, surface activity, and use as inducers for the fabrication of Cu2O nanoparticles†
Received
11th July 2023
, Accepted 16th October 2023
First published on 24th October 2023
Abstract
Discovery of green and novel synthetic routes for nanoparticles (NPs) has drawn a lot of interest due to the distinct nano size and unusual features as well as applications of such particles. Ionic liquid-based surfactants (ILBSs) and gemini ionic liquid-based surfactants (GILBSs) have become some of the best choices to be used as inducers or dispersing agents for the fabrication of nanoparticles. This work involves the synthesis, spectroscopic characterization, and surface property evaluation of three novel GILBSs (4a–c), which incorporate the imidazolium cation as the polar head with an ethylene spacer. The simple synthetic route includes, first, alkylating imidazole-N1 with the as-prepared fatty alkyl chloroacetates followed by quaternization of two equivalents of imidazole-N2 with ethylene dibromide. Investigations into the compounds' surface characteristics and thermodynamic parameters were carried out. The prepared GILBSs, 4a–c, were then used as inducers at various concentrations for the preparation of cuprous oxide nanoparticles. The size and shape of the produced NPs were examined by X-ray diffraction (XRD) and transmission electron microscopy (TEM) analysis in each case to study the effect of concentration on the NP morphology and to determine the best concentration for the NPs fabrication. The XRD patterns of the produced Cu2O NPs contain distinguishable peaks, which refer to crystalline Cu2O. Also, TEM images show that the obtained Cu2O is present in form of well dispersed nanorod particles with sizes about 55 and 23 nm at concentrations of 60 and 200 ppm, respectively.
1 Introduction
Ionic liquids (ILs) are salts with low melting points1 or exist as liquids at room temperature; the latter is known as room temperature ionic liquids (RTILs). Liquids are typically composed of neutral molecules, whereas RTILs are composed of ions.2,3 Surfactants are amphiphilic compounds composed of molecules with a hydrophobic (non-polar) tail and a hydrophilic (polar) head.4 Therefore, conventional ILs can be designed to act as surfactants by attaching long hydrophobic chains to their polar heads and are thus referred to as surface active ionic liquids (SAILs) or ionic liquid-based surfactants (ILBSs).5–25
Recently, ILBSs have piqued the interest of researchers as promising replacements for conventional surfactants in order to overcome limitations and disadvantages such as high toxicity, non-biodegradability, and the need for a high concentration to form stable micelles. This is due to the promising properties of ILBSs that include high thermal stability, low vapor pressure, non-flammability, low toxicity, biodegradability, and non-volatility.1,26–28 Furthermore, they are custom-made and highly tunable, allowing them to have good surface activity in both aqueous and emulsion systems,26,29,30 and to be task-specific.
In the 1930s, a new class of surfactants, gemini surfactants, was discovered and has been found to possess more surface activity than the corresponding conventional surfactants with the same hydrophobic chain and the same hydrophilic head but in a single structure.31 Similarly, two ILBS units are linked together by a rigid or flexible spacer to form gemini ionic liquid-based surfactants (GILBSs). This can be easily exploited to produce various ILBSs and GILBSs with better physicochemical properties,15,32–35 allowing them to be utilized in a myriad of applications.
These compounds are used as solvents, as they can dissolve a broad range of inorganic, organic, and polymeric materials. In addition, they are utilized in solar cells, lubricants, separation applications,36,37 water treatment,38 drug delivery,39 as improvers for the enzymatic degradation of textile dyes,40 corrosion inhibitors,41 biocatalysts, and as inducers for nanoparticles (NPs) synthesis.42–46 A stabilizing material that acts as a capping or dispersing agent is required during the synthesis of NPs to prevent the re-accumulation of the particles. Currently, ILBSs have been used instead of conventional surfactants in the synthesis of NPs as they exhibit better dispersing properties, which make the control of NPs' shape possible. These compounds can be used not only as capping agents but also in the synthesis process.46
When ILBSs are used as stabilizers, they help detect the shape and size of NPs by acting as templates for the NPs. These templates are easily customizable to achieve the desired shape and size. Some of the factors that influence the size and shape of the NPs include the length of the hydrophobic chain and the type and number of the ionic groups in ILBSs.43,47–52 ILBSs with 8 or fewer carbon atoms in their hydrophobic chain lack the effective protection power to stabilize nanoparticles, so the particles tend to accumulate in the moment they are synthesized.44
In this work, three new gemini ionic liquid-based surfactants, including imidazolium cation as the polar head and ethane-1,2-diyl as a spacer, were synthesized and characterized. The surface properties and thermodynamic parameters of these compounds were extensively investigated. One of the prepared surfactants was then used at different concentrations in the preparation of cuprous oxide nanoparticles, and the size and shape of the nanoparticles were detected by XRD and TEM in each case to detect the effect of concentration on the morphology of the produced nanoparticles and to detect the best concentration between them in the production of nanoparticles.
2 Experimental section
Materials and instruments
1-Decanol, 1-dodecanol, 1-hexadecanol, 1,2-dibromoethane, copper sulfate pentahydrate and chloroacetic acid were obtained from Sigma-Aldrich. Potassium sodium tartarate tetrahydrate were obtained from Fisher Scientific UK. Imidazole and p-toluenesulphonic acid were obtained from Loba Chemie. Glucose, petroleum ether and diethyl ether were obtained from Oxford Lab Fine Chem. Xylene, absolute ethanol, anhydrous sodium sulfate, ethyl acetate and sodium hydroxide were obtained from AL-Nasr chemical company. FT-IR (ATR or KBr disc) were performed on Nicolet iS10 FT-IR spectrophotometer. 1H NMR spectra were generated with Bruker Avance (III) 400 MHz signal (Switzerland), using deuterated dimethyl sulfoxide (DMSO-d6) or deuterated chloroform (CDCl3) as a solvent and tetramethylsilane (TMS) as internal reference. Surface tension and interfacial tension values were measured by using a De-Noüy ring tensiometer (Kruss-K6). Miniflex 600 Rigaku XRD Instrument was used to confirm the structure of the nanoparticles and detect their sizes. The morphology of nanoparticles was determined by loading the sample on carbon coated copper grid, the grid with the sample on it was then investigated by HR-TEM (JEOL, JEM-2100, Tokyo, Japan). DLS measurements were performed at room temperature (25 ± 2 °C), with a Zetasizer Nano ZS (Malvern Instruments) equipped with a He–Ne laser (k = 633 nm) and a backscatter detector at a fixed angle of 173°.
Synthesis
General procedure for the synthesis of fatty alkyl 2-chloroacetates (2a–c). These derivatives were synthesized as reported previously.53 Typically, the corresponding fatty alcohol (1a–c, 1 equiv.) namely, 1-decanol, 1-dodecanol, or 1-hexadecanol, was added separately to chloroacetic acid (1 equiv.) in dry xylene. The reaction was catalyzed by p-toluenesulphonic acid (PTSA, 1 mol%) then the mixture was equipped by Dean–Stark under reflux with stirring at about 170 °C till the formation of one equivalent of water. The produced fatty alkyl 2-chloro-acetates 2a–c were isolated by extraction with petroleum ether, the organic phases were dried over anhydrous Na2SO4, and the solvent was evaporated to dryness in vaccou.
Decyl 2-chloroacetate (2a). Colorless viscous liquid, yield = 74%. FT-IR (ATR) ν/cm−1 2958, 2924, 2854 (C–H of aliphatic fatty chain), 1740 (C
O of ester), 1308, 1172 (C–O), 1288, 790 (C–Cl), (Fig. S1†).
Dodecyl 2-chloroacetate (2b). Off-white semisolid, yield = 69%. FT-IR (ATR) ν/cm−1 2952, 2923, 2853 (C–H of aliphatic fatty chain), 1736 (C
O of ester), 1308, 1170 (C–O), 1288, 791 (C–Cl), (Fig. S2†).
General procedure for the synthesis of fatty alkyl 2-(1H-imidazol-1-yl) acetates (3a–c)54. Sodium (1 equiv.) was dissolved in a suitable amount of absolute ethanol, then imidazole (1 equiv.) was added. The mixture was stirred at room temperature till the completion of the reaction that had been monitored by TLC. The excess of solvent was evaporated, and the produced white powder (sodium imidazole) was collected. To a solution of sodium imidazole (1 equiv.) in ethyl acetate, chloroacetates (2a–c, 1 equiv.), diluted with the same solvent, were separately added. The mixture was heated under reflux with stirring at 80 °C for about 12 h and then extracted with ethyl acetate. The products were obtained after evaporating the solvent from the dried organic phases.
Decyl 2-(1H-imidazol-1-yl)acetate (3a). Yellow oil, yield = 83%. FT-IR (KBr disc) ν/cm−1 3123 (C–H of imidazole), 2926, 2854 (C–H of aliphatic fatty chain), 1747 (C
O of ester), 1626 (C
N of imidazole), 1073 (C–O). 1H NMR (400 MHz, CDCl3) δ 7.76 (s, 1H, imidazole), 7.08 (s, 1H, imidazole), 6.97 (s, 1H, imidazole), 4.75 (s, 2H, N–CH2), 4.14 (t, J = 6.8 Hz, 2H, O–CH2), 1.71–1.56 (m, 2H, CH2), 1.38–1.20 (m, 14H, 7CH2), 0.84 (t, J = 6.8 Hz, 3H, CH3), (Fig. S3†).
General procedure for the synthesis of GILBSs (4a–c). 1,2-Dibromoethane (0.5 equiv.) was added to a solution of 1-substituted imidazole (3a–c, 1 equiv.) in ethyl acetate. The mixture was stirred at 70 °C for long time (≈90 h), then the products were obtained in pure form by evaporating the ethyl acetate and washing the residues several times by diethyl ether.
3,3′-(Ethane-1,2-diyl)bis(1-(2-(decyloxy)-2-oxoethyl)-1H-imidazol-3-ium) bromide (4a). Colorless viscous liquid, yield = 63%. FT-IR (ATR) ν/cm−1 3418 (OH, hygroscopic water), 3137 (C–H of imidazole), 2958, 2920, 2850 (C–H, aliphatic fatty chain), 1747 (C
O of ester), 1624 (C
N of imidazole), 1183 (C–O). 1H NMR (400 MHz, DMSO-d6) δ 9.18 (s, 2H), 7.79 (s, 4H), 5.34 (s, 4H), 4.15 (t, J = 6.5 Hz, 4H), 3.36 (t, J = 6.5 Hz, 4H), 1.70–1.50 (m, 4H), 1.43–1.12 (m, 28H), 0.86 (t, J = 6.8 Hz, 6H). GC-EIMS for C32H56Br2N4O4, RT (min) = 24.82 with molecular weight higher than 700.
3,3′-(Ethane-1,2-diyl)bis(1-(2-(dodecyloxy)-2-oxoethyl)-1H-imidazol-3-ium) bromide (4b). White solid, m. p. = 96–98 °C, yield = 59%. FT-IR (ATR) ν/cm−1 3417 (OH, hygroscopic water), 3111 (C–H of imidazole), 2958, 2921, 2851 (C–H, aliphatic fatty chain), 1750 (C
O of ester), 1627 (C
N of imidazole), 1180 (C–O). 1H NMR (400 MHz, DMSO-d6) δ 9.17 (s, 2H), 7.79 (d, J = 3.0 Hz, 4H), 5.34 (s, 4H), 4.14 (t, J = 6.6 Hz, 4H), 3.38–3.15 (m, 4H), 1.70–1.52 (m, 4H), 1.52–1.19 (m, 36H), 0.85 (t, J = 6.8 Hz, 6H). GC-EIMS for C36H64Br2N4O4, RT (min) = 26.78 with molecular weight higher than 700.
3,3′-(Ethane-1,2-diyl)bis(1-(2-(hexadecyloxy)-2-oxoethyl)-1H-imidazol-3-ium) bromide (4c). Off-white solid, m. p. = 128–130 °C, yield = 67%. FT-IR (ATR) ν/cm−1 3405 (OH, hygroscopic water), 3109 (C–H of imidazole), 2956, 2918, 2849 (C–H, aliphatic fatty chain), 1751 (C
O of ester), 1624 (C
N of imidazole), 1181 (C–O). 1H NMR (400 MHz, DMSO-d6) δ 9.12 (s, 2H), 7.78 (d, J = 3.5, 4H), 5.32 (s, 2H), 5.09 (s, 2H), 4.13 (t, J = 6.7 Hz, 4H), 3.36 (t, J = 6.4 Hz, 4H), 1.60 (m, 2H), 1.27 (m, 52H), 0.85 (t, J = 6.8 Hz, 6H). GC-EIMS for C44H80Br2N4O4, RT (min) = 35.79 with molecular weight higher than 800.
Surface properties
(a) Surface and interfacial tension measurements. The surface tension (ST) was measured using a Krüss (Model K 6) tensiometer with a platinum ring.51 Using distilled water and a ST of 72 dyne cm−1 at 25 °C, a 0.1% weight surfactant solution was created. Before use, the platinum ring was cleaned with acetone and water. At least three times were required to set the ST value before the average was approved. For the ionic liquid solution-oil system, interfacial tension (IT) was measured at 25 °C using the same ionic liquid solution. The IT value at which the ring broke apart at the interface between the water and oil is identified. The combined IT value of the used paraffin oil and distilled water is 56.2 dyne per cm−1.53–55
(b) Foam height. In a 500 mL graduated cylinder set at 25 °C, 100 mL of an ionic liquid aqueous solution at 0.1% (wt) was violently shaken for about 20 seconds. The height of the created foam at the instant the shaking stopped was measured in millimeters (mm).53,55
(c) Emulsion stability. A 25 mL graduated cylinder containing 10 mL of a 0.1% (wt) aqueous solution of the ionic liquid and 5 mL of paraffin oil was vigorously shaken for approximately two minutes at 25 °C. The time it took to separate 9 mL of a pure aqueous solution of the ionic liquid from the emulsion system with the cylinder in a steady state was noted as a measure of the emulsion stability.56,57
Preparation of cuprous oxide NPs
Fehling's solutions were prepared as follows: Fehling A was prepared by dissolving 3.45 g of CuSO4·5H2O in 500 mL of distilled water. Fehling B was prepared by dissolving 17.3 g of potassium sodium tartarate tetrahydrate and 6.0 g sodium hydroxide in 500 mL of distilled water.
50 mL of different concentrations (60, 200, and 400 ppm) of 4b was prepared. For compounds 4a and 4c, only 200 ppm were prepared.
(5 mL) of each of (FA) and (FB) were mixed in a beaker and (50 mL) of the prepared concentration of 4a, 4b or 4c was added to the mixture and stirred for (15 min). Then an aqueous solution of (0.5 g) glucose in (5 mL) distilled water was added to the previous mixture and the whole content was then heated at about (60 °C) under continuous vigorous stirring. After about (15–20 min.) of the reaction time a brick-red precipitate of Cu2O was formed, the precipitate was then filtered off, washed several times by deionized water then with ethanol and it was dried in an oven at (60 °C) for 4 h. The produced cuprous oxide nanoparticles were investigated by XRD and TEM to determine the particle size in each case and comparing the effect of different concentrations on the produced nanoparticles' sizes.
3 Results and discussion
Synthesis
Cationic gemini surfactants showed potent behavior in inhibiting corrosion of metals, controlling sulfate-reducing bacteria and synthesis of nanoparticles.58–60 Ionic liquid-based surfactants and especially gemini ionic liquid-based surfactants (GILBSs) are promising compounds due to their advanced properties and variable applications. A series of new GILBSs were synthesized according to the following steps (Scheme 1). Firstly, fatty alcohols (1-decanol, 1-dodecanol, 1-hexadecanol) were esterified with chloroacetic acid to produce the corresponding chloroacetates (2a–c). FT-IR spectra of these acetates showed characteristic absorption band for carbonyl-ester at around 1750 cm−1 beside bands for C–O and C–Cl at 1180 and 790 cm−1, respectively, (Fig. S1 and S2†). Then, these esters were reacted with the as-prepared sodium imidazole to give 1-alkyl imidazole derivatives (3a–c). New peaks in FT-IR spectra at around 3100 and 1620 cm−1, which confirm the presence of aromatic C–H and C
N bonds of imidazole, respectively. In addition, their 1H NMR spectra exhibited three signals of aromatic protons at 7–8 ppm, (Fig. S3†).
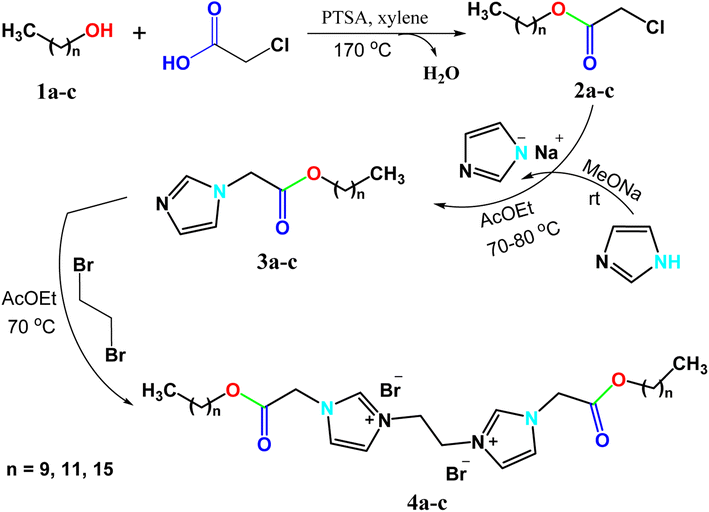 |
| Scheme 1 Synthetic pathway for the targeted GILBSs 4a–c. | |
Finally, the targeted GILBSs 4a–c were obtained via gentle reflux of alkyl imidazoles with 1,2-dibromoethane. In addition to the signals of aromatic and fatty chain protons, new signals appeared in the 1H NMR spectra of GILBSs 4a–c at around 5.2–5.4 ppm attributable to ethylene spacer protons as clearly shown in Fig. 1, S4, and S5.†
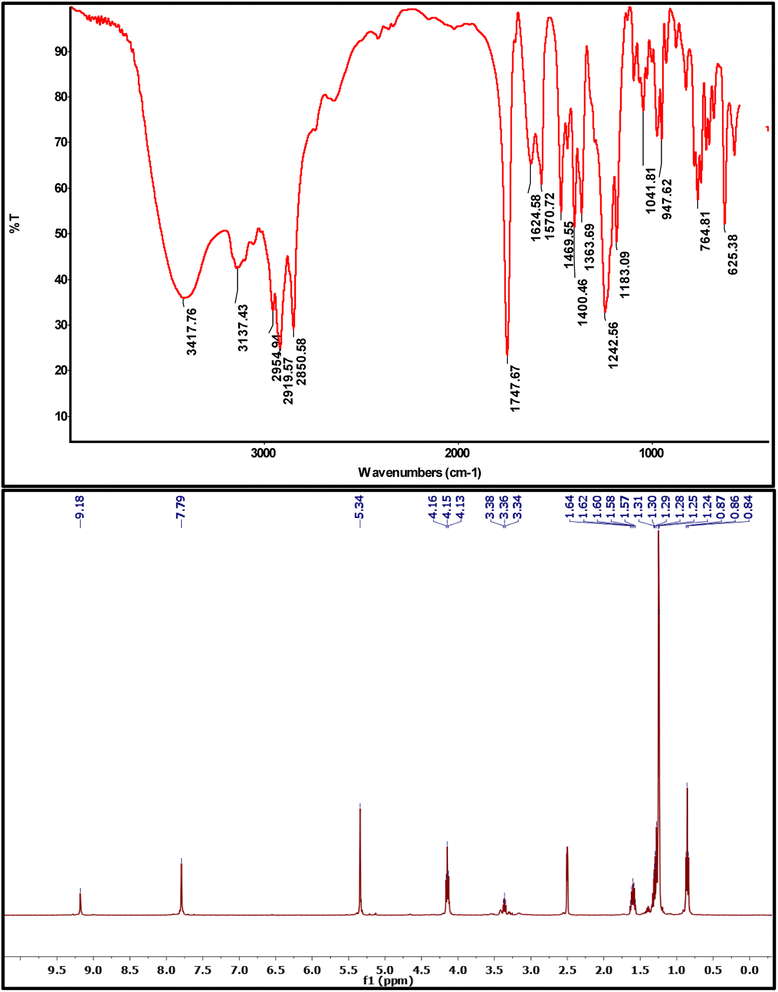 |
| Fig. 1 FT-IR and 1H NMR (DMSO-d6) spectra for the GILBS 4a. | |
Surface properties
Surface-active attributes measurements of the synthetic ionic liquid (GILBSs) were performed to assess their surface activity, and the resulting data are shown in Table 1.
Table 1 Surface properties of the synthesized GILBSs 4a–c
GILBS |
Surface tension (mN m−1) |
Interfacial tension (mN m−1) |
Foam height 0.1% (mm) |
Emulsion stability (min:sec) |
CMC/mM |
4a |
35.03 |
13 |
140 |
34:35 |
2.4 |
4b |
40.77 |
16.3 |
120 |
28:14 |
2.2 |
4c |
46.07 |
17.3 |
110 |
14:26 |
3.1 |
Surface and interfacial tension
From Table 1, it is clear that by adding GILBSs compounds, the surface tension of water decreases, as well as the interfacial tension, proving that these compounds have the ability to affect the surface tension and work in the interfaces. The table also shows that the surface tension rises as the hydrocarbon chain lengthens, suggesting that the compounds' influence on surface tension reduces as the lengthening of the hydrocarbon chain.
Emulsion stability (ES)
The higher the emulsion stability capacity of these compounds, the longer it takes to separate the emulsion. It is evident from Table 1 that the synthetic ionic liquids (GILBSs) have a significant capacity for emulsion formation; however, when hydrophobicity increases, this capacity for emulsion formation decreases, especially with compound 4c.
Foam height (FH)
The types of applications of GILBSs depend on their capacity to foam and the height of their foam, which is a significant occurrence. All of the prepared GILBSs had a high foaming height, as shown by the data in Table 1. Additionally, the values of foam height often decrease as the length of the fatty chain rises.
Critical micelle concentration (CMC)
Due to the hydrophobic group that these compounds contain, when they are dissolved in water, the molecules start to rise to the water's surface, which helps to lower surface tension. This effect lasts until the molecules stop rising, regardless of how much the concentration of the dissolved substances rises. This occurs as a result of water molecules forming micelles around themselves. This concentration is known as the CMC. Utilizing the surface tension method, it is determined. It was determined by plotting the aqueous GILBSs solutions' corresponding logarithms of concentration and surface tension (Fig. 2). The information is displayed in Table 1, which demonstrates that the CMC values of compounds 4a and 4b decrease as the size of the hydrophobic group increases but increase once more in compound 4c. This might be the fact that 4c has a huge size makes mobility more challenging, and it hardly ever migrates to the surface.
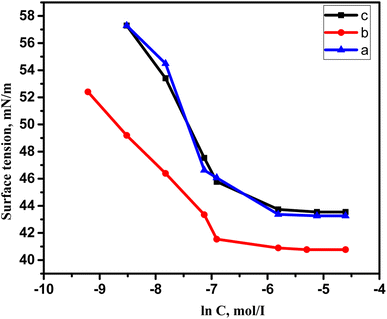 |
| Fig. 2 Variation of surface tension vs. the concentration of the synthesized cationic gemini surfactant at 25 °C. | |
Effectiveness (πCMC)
The calculation of πCMC is used to find out how these compounds can alter the water's surface. The higher the value of πCMC, the greater the compound's capacity to lower the surface tension of water. It was determined using the formula given below using the difference between the surface tension of pure water (γo) and the surface tension of the surfactant's aqueous solution at CMC (γCMC).61 Results from the πCMC calculations GILBSs are shown in Table 2.
Table 2 Thermodynamic parameters of the synthesized GILBSs 4a–c
Compd |
CMC/mM |
γCMC/mN m−1 |
ΠCMC/mN m−1 |
Γmax × 1011/mol cm−2 |
Amin/nm2 |
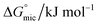
|

|
4a |
2.4 |
43.62 |
28.38 |
5.05 |
3.2 |
−14.86 |
−20.30 |
4b |
2.2 |
40.89 |
31.11 |
3.61 |
4.6 |
−15.11 |
−23.69 |
4c |
3.1 |
43.37 |
28.63 |
5.29 |
3.15 |
−14.36 |
−19.77 |
Maximum surface excess (Γmax)
Γmax calculations are made in order to determine the material particle concentration on the water's surface. The amount of ionic liquid particles on the surface has an impact on the water's surface tension, as the greater the number of particles on the surface of the water, the lower the surface tension of the water. Γmax was determined using the Gibbs adsorption isotherm eqn (2).62 |
Γmax = −(δγ/δ log c)T/2.303nRT
| (2) |
where, (δγ/δ
log
c) is the slope of the straight-line portion in the surface tension vs. −log concentration at 25 °C, n = 2 in case of conventional surfactants, R is the gas constant, T is the absolute temperature. Table 2 shows that the concentration of molecules at the air/water interface falls as the hydrocarbon chain lengthens in compounds 4a and 4b before increasing once more in compound 4c.
Minimum surface area (Amin)
The average surface area occupied by a molecule at the air/water interface is known as Amin, eqn (3) has been used to calculate Amin.63where Amin is in nm2 and NA, is Avogadro's constant (6.022 × 1023 mol−1). The space available for each molecule at the air/water contact shrinks as its population grows. According to the findings in Table 2, the Amin increases in compound 4a and 4b as the number of molecules on the surface decreases.
Thermodynamic parameters of the synthesized ionic liquid
The most crucial characteristics of ionic liquid to be researched are their thermodynamic properties, specifically their adsorption free energy
and micellization free energy
. Through its calculations, we can judge whether the compounds prefer to work inside the solution and form a micelle or migrate to the intersurface. Gibbs adsorption equations (eqn (4) and (5)) were used to calculate micellization and adsorption free energy.64 |
 | (4) |
|
 | (5) |
Table 2 displays the values of
, which came from eqn (4) and (5). The outcomes show that the micellization and adsorption of Gibbs free energies are both negative. According to fundamental thermodynamic principles, all of the molecules of the ionic liquid being studied tend to spontaneously form micelles in the solution or become adsorbed at the interface.
It is also obvious that the values are more negative than those of, indicating a higher capacity for absorption at the air/water interface than micellization into the solution. From Table 2, it is evident that the ionic liquid compounds have a better probability of being absorbed at the interface as the length of the hydrocarbon chain increases, as opposed to micellization into the solution, as in the case of compounds 4a and 4b. This interpretation holds true for compound 4c, however due to the length of its chain, which makes it harder for it to move, it require a larger energy to have either micellization or adsorption on the interface.
Preparation and characterization of Cu2O NPs aided by GILBSs
Cuprous oxide stands out as an important oxide due to its several functions. Solar cell,65 catalysis,66 and batteries67 are a few examples of how cuprous oxide is used in chemistry. Many ways have been developed to synthesize Cu2O NPs such as solution-phase,68 thermal decomposition,69 and sonication70 methods. The surfactant-assisted approach is a quick, convenient, and inexpensive way to create size-controllable nanocrystals. Surfactant molecules may work as regulators for the growth of particles or inhibitors for their agglomeration. To prevent the newly formed particles from aggregating, a covering film is formed on top of them.71,72
In this work, we used different concentrations of the synthesized GILBS 4b (60, 200, and 400 ppm) as inducer for the facile preparation of Cu2O NPs. The conversion of Cu2+ to Cu+ is brought about by heating Fehling's solution in the presence of a weak reducing agent, like glucose. An orange to red precipitate of Cu2O is formed as a result of the reduction because the tartarate ion cannot coordinate with the cuprous ion. Actually, when the cupric ion in the tartarate complex comes into touch with the aldehyde group of the glucose molecule, it is reduced to a cuprous ion, and the aldehyde is oxidized to carboxylic acid (Scheme 2). Therefore, we developed a simple technique for the production of Cu2O NPs employing the GILBS 4b as surfactants to control the size of these particles by utilizing Fehling's solution's reactivity with glucose.
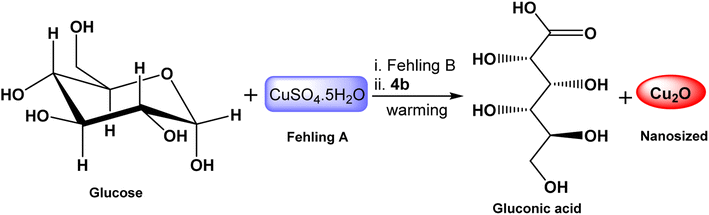 |
| Scheme 2 Preparation route for the Cu2O NPs induced by GILBS 4b. | |
The transmission electron microscopy (TEM) images of the obtained Cu2O showed well dispersed roughly nanorod particles (Fig. 3). The mean sizes of Cu2O nanorods are about 55 nm and 23 nm for the GILBS 4b at 60 and 200 ppm, respectively. The size of nanoparticles obtained from the TEM studies are in close agreement with those calculated from XRD diffraction patterns.
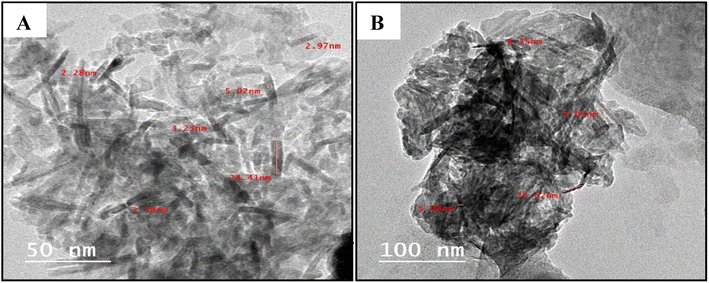 |
| Fig. 3 TEM images of the prepared Cu2O NPs induced by GILBS 4b at a concentration of (A) 60 ppm, and (B) 200 ppm. | |
Patterns for powder XRD for the nanosized Cu2O were given in Fig. 4 which contain five clearly distinguishable peaks. They are all precisely indexed to crystalline Cu2O both in terms of peak position and relative intensity. The crystal planes 110, 111, 200, 220, 311 and 222 of crystalline Cu2O are represented by the peaks with 2θ values of 29.601, 36.521, 42.441, 61.541, 73.691 and 77.611, respectively. The absence of distinctive Cu–O or Cu metal peaks in the XRD patterns suggests that phase-pure cuprous oxide can be produced easily.
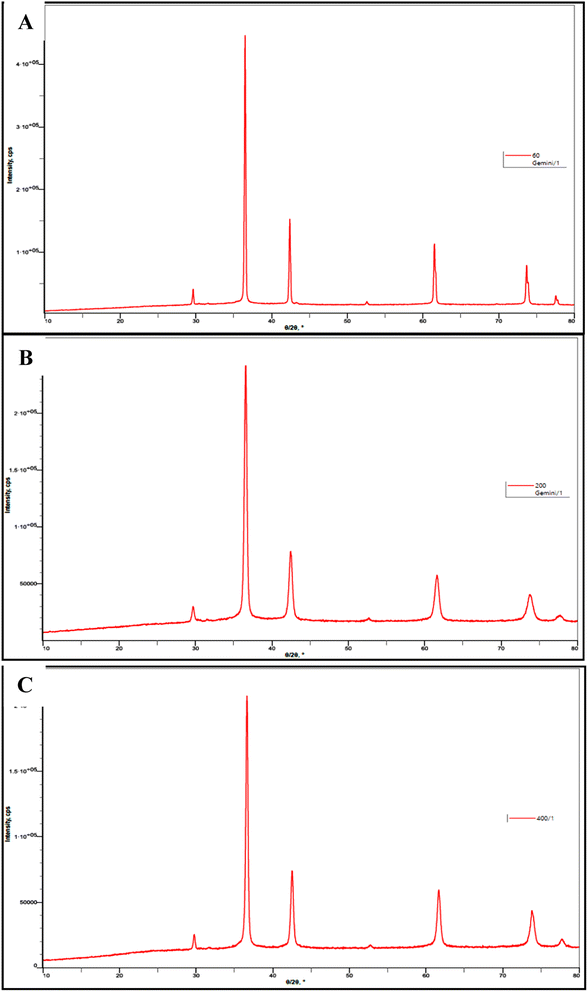 |
| Fig. 4 Powder XRD patterns of the prepared Cu2O NPs induced by 4b at (A) 60 ppm, (B) 200 ppm, and (C) 400 ppm. | |
Nanowires of Cu2O with diameter of about 100 nm and lengths of about 16 μm have been prepared by potentiostatic deposition by reduction of alkaline cupric lactate solution at the cathode on polycarbonate membrane. Limitations of this method includes scalability issues, pH management, and accurate temperature control.73 Firmansyah, et al. reported the effect of ethanol on the preparation of Cu2O NPs by copper nitrate spray pyrolysis. The produced NPs changed, by addition of ethanol, from shell-like to solid-spherical, and their sizes decreased with increasing ethanol volume to 50 nm. This process has some drawbacks, including high temperature, expensive costs, and careful temperature control.74
Furthermore, Cu2O NPs were synthesized by reduction of copper(II) sulfate present in an aqueous solution containing polyvinylpyrrolidone (PVP), sodium citrate and sodium carbonate, then exposing the produced solution to air for 16 days. The formed NPs have truncated octahedral inner hollow shape with size about 700 nm and inner edge length about 300 nm. Time-consuming procedure and relatively large particle size limit the application of this method.75
For further clarification, nanoparticles formed by using the GILBS 4b at 200 ppm were examined using Dynamic Light Scattering (DLS) technique. Fig. 5 illustrates that the prepared nanoparticles have an average hydrodynamic diameter of ∼531 nm and polydispersity index of 0.796. These results indicate some aggregates formation in the applied dispersant media (water). Furthermore, the crystallite estimated from the XRD results are in good agreement with the TEM results, whereas the size values obtained with DLS are larger. This is due to the fact that the DLS technique measures the hydrodynamic size of the particles. This finding indicates the presence of the solvation layer around the synthesized nanoparticles. However, detailed analysis of the solvation shell is not a subject of this study.
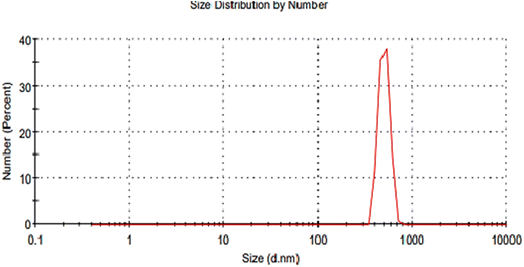 |
| Fig. 5 DLS analysis of the prepared Cu2O NPs induced by GILBS 4b at a concentration of 200 ppm. | |
Depending on the previous results, after studying the effect of compound 4b on the process of Cu2O NPs preparation, 200 ppm is the most effective concentration in the process of Cu2O NPs preparation. As a result, experiments were conducted for the other synthesized GILBS 4a and 4c at the same concentration.
TEM images of the obtained Cu2O showed well-dispersed roughly spherical particles and the mean particle sizes of Cu2O are about 18 nm using compound GILBS 4c, whereas those prepared using GILBS 4a are complete and incomplete spherical shape with high agglomeration and not well dispersed with particle size 61.5 nm (Fig. 6).
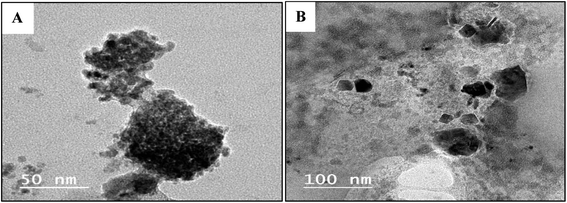 |
| Fig. 6 TEM images of the prepared Cu2O NPs induced by GILBS 4a (A) and 4c (B) at a concentration of 200 ppm. | |
By comparing the results obtained based on the hydrocarbon chain length, it is evident that the process of Cu2O NPs preparation is as follows: 61.5 nm, 23 nm, and 18 nm by using GILBS 4a, 4b and 4c at 200 ppm, respectively, which indicates that by increasing the hydrocarbon chain length, these compounds are more effective in forming nanoparticles. This could be because GILBS molecules are more repellent to one another as chain length increases, which helps the molecules spread apart.
XRD data in agreement with those obtained from TEM, with all the peaks related to cuprous oxide and absence of distinctive Cu–O or Cu metal peaks, as in case of 4b, that can be evidenced on phase-pure of prepared NPs (Fig. 7).
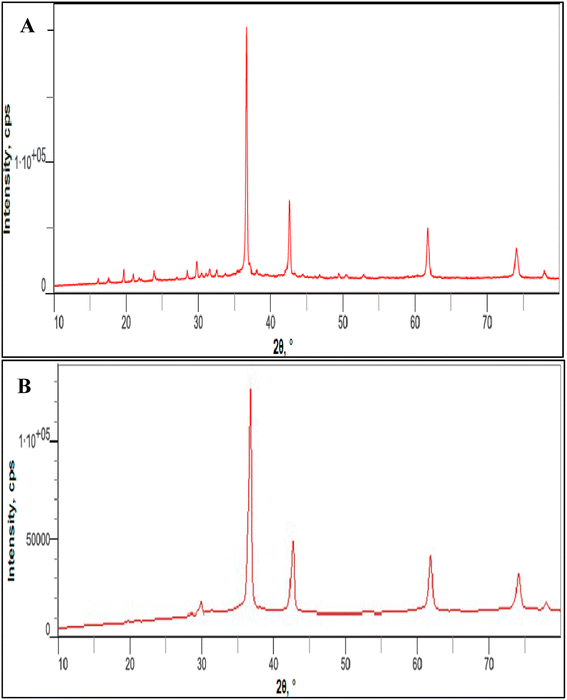 |
| Fig. 7 Powder XRD patterns of the prepared Cu2O NPs induced by GILBS 4a (A) and 4c (B) at a concentration of 200 ppm. | |
Bai group prepared Cu2O nanoparticles with different shapes and sizes by reduction of aqueous solution of copper(II) acetate by ascorbic acid, the solution contained NaOH and PVP as a stabilizer. They managed this by changing the concentrations of the different reagents and heating temperature.76 Similarly, Huang and Chiu synthesized Cu2O NPs by redox reaction from copper chloride in presence of NaOH, hydroxyl amine and SDS as an inducer. The particle size ranges from 40 to 420 nm.77 Our strategy utilizes a novel synthesized GILBSs as inducers for the fabrication of nano-sized cuprous oxide. It could overcome almost all the limitations of the previously reported methods as it does not need pH management, high temperature, fine chemicals, and long time. It produces nanoparticles with relatively small sizes.
4 Conclusion
In this study, three new gemini ionic liquid-based surfactants GILBSs were synthesized and characterized. The polar head of these surfactants included the imidazolium cation with ethane-1,2-diyl as the spacer. Investigations into the surface properties and thermodynamic parameters of these compounds were extensively reported. In order to fabricate cuprous oxide nanoparticles, the prepared surfactants, 4a–c were utilized in a variety of concentrations. Additionally, the impact of concentration on the morphology of the targeted nanoparticles was evaluated and to choose the optimal concentration among them, the size and shape of the nanoparticles were afterwards evaluated by XRD and TEM in each case. The size of the nanoparticles determined by TEM examinations and the ones estimated from XRD diffraction patterns are almost identical, and present indeed in the nano size with good size ranges even at very low concentrations.
Ethical statement
The study was conducted and approved according to the guidelines of the declaration of the ethical committee of the Faculty of Science, Benha University (No. 42 chm).
Conflicts of interest
All authors declare that they have no conflict of interest.
References
- S. Marullo, C. Rizzo, N. T. Dintcheva, F. Giannici and F. D'Anna, Ionic liquids gels: Soft materials for environmental remediation, J. Colloid Interface Sci., 2018, 517, 182 CrossRef CAS PubMed.
- Z. Zeng, B. S. Phillips, J.-C. Xiao and J. n. M. Shreeve, Polyfluoroalkyl, Polyethylene Glycol, 1,4-Bismethylenebenzene, or 1,4-Bismethylene-2,3,5,6-Tetrafluorobenzene Bridged Functionalized Dicationic Ionic Liquids: Synthesis and Properties as High Temperature Lubricants, Chem. Mater., 2008, 20, 2719 CrossRef CAS.
- Y. Cao, J. Wu, J. Zhang, H. Li, Y. Zhang and J. He, Room temperature ionic liquids (RTILs): A new and versatile platform for cellulose processing and derivatization, Chem. Eng. J., 2009, 147, 13 CrossRef CAS.
- S. Sasi, M. P. Rayaroth, C. T. Aravindakumar and U. K. Aravind, Identification of surfactants and its correlation with physicochemical parameters at the confluence region of Vembanad Lake in India, Environ. Sci. Pollut. Res. Int., 2018, 25, 20527 CrossRef CAS PubMed.
- M. Anouti, J. Jones, A. Boisset, J. Jacquemin, M. Caillon-Caravanier and D. Lemordant, Aggregation behavior in water of new imidazolium and pyrrolidinium alkycarboxylates protic ionic liquids, J. Colloid Interface Sci., 2009, 340, 104 CrossRef CAS PubMed.
- N. Cheng, X. Ma, X. Sheng, T. Wang, R. Wang, J. Jiao and L. Yu, Aggregation behavior of anionic surface active ionic liquids with double hydrocarbon chains in aqueous solution: Experimental and theoretical investigations, Colloids Surf., A, 2014, 453, 53 CrossRef CAS.
- M. Ao and D. Kim, Aggregation Behavior of Aqueous Solutions of 1-Dodecyl-3-methylimidazolium Salts with Different Halide Anions, J. Chem. Eng. Data, 2013, 58, 1529 CrossRef CAS.
- T. Singh and A. Kumar, Aggregation Behavior of Ionic Liquids in Aqueous Solutions: Effect of Alkyl Chain Length, Cations, and Anions, J. Phys. Chem. B, 2007, 111, 7843 CrossRef CAS PubMed.
- B. Dong, X. Zhao, L. Zheng, J. Zhang, N. Li and T. Inoue, Aggregation behavior of long-chain imidazolium ionic liquids in aqueous solution: Micellization and characterization of micelle microenvironment, Colloids Surf., A, 2008, 317, 666 CrossRef CAS.
- M. A. Rather, G. M. Rather, S. A. Pandit, S. A. Bhat and M. A. Bhat, Determination of cmc of imidazolium based surface active ionic liquids through probe-less UV-vis spectrophotometry, Talanta, 2015, 131, 55 CrossRef CAS PubMed.
- N. V. Sastry, N. M. Vaghela and V. K. Aswal, Effect of alkyl chain length and head group on surface active and aggregation behavior of ionic liquids in water, Fluid Phase Equilib., 2012, 327, 22 CrossRef CAS.
- T. Singh, K. S. Rao and A. Kumar, Effect of ethylene glycol and its derivatives on the aggregation behavior of an ionic liquid 1-butyl-3-methyl imidazolium octylsulfate in aqueous medium, J. Phys. Chem. B, 2012, 116, 1612 CrossRef CAS PubMed.
- T. Inoue, H. Ebina, B. Dong and L. Zheng, Electrical conductivity study on micelle formation of long-chain imidazolium ionic liquids in aqueous solution, J. Colloid Interface Sci., 2007, 314, 236 CrossRef CAS PubMed.
- A. Modaressi, H. Sifaoui, M. Mielcarz, U. Domańska and M. Rogalski, Influence of the molecular structure on the aggregation of imidazolium ionic liquids in aqueous solutions, Colloids Surf., A, 2007, 302, 181 CrossRef CAS.
- O. A. El Seoud, N. Keppeler, N. I. Malek and P. D. Galgano, Ionic Liquid-Based Surfactants: Recent Advances in Their Syntheses, Solution Properties, and Applications, Polymers, 2021, 13, 1100 CrossRef CAS PubMed.
- N. I. Malek, Z. S. Vaid, U. U. More and O. A. El Seoud, Ionic-liquid-based surfactants with unsaturated head group: synthesis and micellar properties of 1-(n-alkyl)-3-vinylimidazolium bromides, Colloid Polym. Sci., 2015, 293, 3213 CrossRef CAS.
- R. Vanyúr, L. Biczók and Z. Miskolczy, Micelle formation of 1-alkyl-3-methylimidazolium bromide ionic liquids in aqueous solution, Colloids Surf., A, 2007, 299, 256 CrossRef.
- F. Geng, J. Liu, L. Zheng, L. Yu, Z. Li, G. Li and C. Tung, Micelle Formation of Long-Chain Imidazolium Ionic Liquids in Aqueous Solution Measured by Isothermal Titration Microcalorimetry, J. Chem. Eng. Data, 2010, 55, 147 CrossRef CAS.
- M. Blesic, A. n. Lopes, E. Melo, Z. Petrovski, N. V. Plechkova, J. N. C. Lopes, K. R. Seddon and L. s. P. N. Rebelo, On the Self-Aggregation and Fluorescence Quenching Aptitude of Surfactant Ionic Liquids, J. Phys. Chem. B, 2008, 112, 8645 CrossRef CAS PubMed.
- A. Cornellas, L. Perez, F. Comelles, I. Ribosa, A. Manresa and M. T. Garcia, Self-aggregation and antimicrobial activity of imidazolium and pyridinium based ionic liquids in aqueous solution, J. Colloid Interface Sci., 2011, 355, 164 CrossRef CAS PubMed.
- M. Blesic, M. H. Marques, N. V. Plechkova, K. R. Seddon, L. P. N. Rebelo and A. n. Lopes, Self-aggregation of ionic liquids: micelle formation in aqueous solution, Green Chem., 2007, 9, 481 RSC.
- H. Wang, J. Wang, S. Zhang and X. Xuan, Structural Effects of Anions and Cations on the Aggregation Behavior of Ionic Liquids in Aqueous Solutions, J. Phys. Chem. B, 2008, 112, 16682 CrossRef CAS PubMed.
- X. Wang, J. Liu, L. Yu, J. Jiao, R. Wang and L. Sun, Surface adsorption and micelle formation of imidazolium-based zwitterionic surface active ionic liquids in aqueous solution, J. Colloid Interface Sci., 2013, 391, 103 CrossRef CAS PubMed.
- B. Dong, N. Li, L. Zheng, L. Yu and T. Inouen, Surface Adsorption and Micelle Formation of Surface Active Ionic Liquids in Aqueous Solution, Langmuir, 2007, 23, 4178 CrossRef CAS PubMed.
- O. A. El Seoud, P. A. Pires, T. Abdel-Moghny and E. L. Bastos, Synthesis and micellar properties of surface-active ionic liquids: 1-alkyl-3-methylimidazolium chlorides, J. Colloid Interface Sci., 2007, 313, 296 CrossRef CAS PubMed.
- M. K. Ali, R. M. Moshikur, R. Wakabayashi, Y. Tahara, M. Moniruzzaman, N. Kamiya and M. Goto, Synthesis and characterization of choline-fatty-acid-based ionic liquids: A new biocompatible surfactant, J. Colloid Interface Sci., 2019, 551, 72 CrossRef CAS PubMed.
- T. J. Trivedi, K. S. Rao, T. Singh, S. K. Mandal, N. Sutradhar, A. B. Panda and A. Kumar, Task-specific, biodegradable amino acid ionic liquid surfactants, ChemSusChem, 2011, 4, 604 CrossRef CAS PubMed.
- P. S. Gehlot, A. Kulshrestha, P. Bharmoria, K. Damarla, K. Chokshi and A. Kumar, Surface-Active Ionic Liquid Cholinium Dodecylbenzenesulfonate: Self-Assembling Behavior and Interaction with Cellulase, ACS Omega, 2017, 2, 7451 CrossRef CAS PubMed.
- M. U. H. Shah, M. Sivapragasam, M. Moniruzzaman, M. M. R. Talukder, S. B. Yusup and M. Goto, Aggregation behavior and antimicrobial activity of a micellar system of binary ionic liquids, J. Mol. Liq., 2018, 266, 568 CrossRef CAS.
- K. S. Rao, P. S. Gehlot, T. J. Trivedi and A. Kumar, Self-assembly of new surface active ionic liquids based on Aerosol-OT in aqueous media, J. Colloid Interface Sci., 2014, 428, 267 CrossRef PubMed.
- M. J. Rosen and D. J. Tracy, Gemini Surfactants, J. Surfactants Deterg., 1998, 1, 547 CrossRef CAS.
- R. Zana, Alkanediyl-alpha,omega-bis(dimethylalkylammonium bromide) surfactants 10. Behavior in aqueous solution at concentrations below the critical micellization concentration: an electrical conductivity study, J. Colloid Interface Sci., 2002, 246, 182 CrossRef CAS PubMed.
- F. M. Menger and C. A. Littau, Gemini Surfactants: A New Class of Self-Assembling Molecules, J. Am. Chem. Soc., 1993, 115, 10083 CrossRef CAS.
- J. r. m. Gaucheron, T. Wong, K. F. Wong, N. Maurer and P. R. Cullis, Synthesis and Properties of Novel Tetraalkyl Cationic Lipids, Bioconjugate Chem., 2002, 13, 671–675 CrossRef CAS PubMed.
- E. Fisicaro, C. Compari, E. Duce, G. Donofrio, B. Rozycka-Roszak and E. Wozniak, Biologically active bisquaternary ammonium chlorides: physico-chemical properties of long chain amphiphiles and their evaluation as non-viral vectors for gene delivery, Biochim. Biophys. Acta, 2005, 1722, 224 CrossRef CAS PubMed.
- S. M. Rajput, U. U. More, Z. S. Vaid, K. D. Prajapati and N. I. Malek, Impact of organic solvents on the micellization and interfacial behavior of ionic liquid based surfactants, Colloids Surf., A, 2016, 507, 182 CrossRef CAS.
- S. M. Tawfik, Simple one step synthesis of gemini cationic surfactant-based ionic liquids: Physicochemical, surface properties and biological activity, J. Mol. Liq., 2015, 209, 320 CrossRef CAS.
- R. Goutham, P. Rohit, S. S. Vigneshwar, A. Swetha, J. Arun, K. P. Gopinath and A. Pugazhendhi, Ionic liquids in wastewater treatment: A review on pollutant removal and degradation, recovery of ionic liquids, economics and future perspectives, J. Mol. Liq., 2022, 349, 118150 CrossRef CAS.
- R. M. Moshikur, M. K. Ali, M. Moniruzzaman and M. Goto, Recent advances in surface-active ionic liquid-assisted self-assembly systems for drug delivery, Curr. Opin. Colloid Interface Sci., 2021, 56, 101515 CrossRef CAS.
- R. M. F. Bento, M. R. Almeida, P. Bharmoria, M. G. Freire and A. P. M. Tavares, Improvements in the enzymatic degradation of textile dyes using ionic-liquid-based surfactants, Sep. Purif. Technol., 2020, 235, 116191 CrossRef CAS.
- K. Qiao and Y. Zeng, Comparative study on two imidazolium-based ionic liquid surfactants as corrosion inhibitors for N80 steel in 15% hydrochloric acid solution, Mater. Corros., 2020, 71, 1913 CrossRef CAS.
- R. Bussamara, W. W. Melo, J. D. Scholten, P. Migowski, G. Marin, M. J. Zapata, G. Machado, S. R. Teixeira, M. A. Novak and J. Dupont, Controlled synthesis of Mn3O4 nanoparticles in ionic liquids, Dalton Trans., 2013, 42, 14473 RSC.
- K.-S. Kim, D. Demberelnyamba and H. Lee, Size-Selective Synthesis of Gold and Platinum Nanoparticles Using Novel Thiol-Functionalized Ionic Liquids, Langmuir, 2004, 20, 556 CrossRef CAS PubMed.
- O. Naderi, M. Nyman, M. Amiri and R. Sadeghi, Synthesis and characterization of silver nanoparticles in aqueous solutions of surface active imidazolium-based ionic liquids and traditional surfactants SDS and DTAB, J. Mol. Liq., 2019, 273, 645 CrossRef CAS.
- K. Schutte, H. Meyer, C. Gemel, J. Barthel, R. A. Fischer and C. Janiak, Synthesis of Cu, Zn and Cu/Zn brass alloy nanoparticles from metal amidinate precursors in ionic liquids or propylene carbonate with relevance to methanol synthesis, Nanoscale, 2014, 6, 3116 RSC.
- G. Singh, K. Komal, G. Singh, M. Kaur and T. S. Kang, A new sustainable approach towards preparation of sunlight active Ag/AgBr Janus nanoparticles using non-toxic surface active ionic liquid, J. Mater. Chem. A, 2019, 7, 5185 RSC.
- D. S. Jacob, L. Bitton, J. Grinblat, I. Felner, Y. Koltypin and A. Gedanken, Are Ionic Liquids Really a Boon for the Synthesis of Inorganic Materials? A General Method for the Fabrication of Nanosized Metal Fluorides, Chem. Mater., 2006, 18, 3162 CrossRef CAS.
- C. M. Lee, H. J. Jeong, S. T. Lim, M. H. Sohn and D. W. Kim, Synthesis of iron oxide nanoparticles with control over shape using imidazolium-based ionic liquids, ACS Appl. Mater. Interfaces, 2010, 2, 756 CrossRef CAS PubMed.
- E. Redel, R. Thomann and C. Janiak, First Correlation of Nanoparticle Size-Dependent Formation with the Ionic Liquid Anion Molecular Volume, Inorg. Chem., 2008, 47, 14–16 CrossRef CAS PubMed.
- H. J. Ryu, L. Sanchez, H. A. Keul, A. Raj and M. R. Bockstaller, Imidazolium-based ionic liquids as efficient shape-regulating solvents for the synthesis of gold nanorods, Angew. Chem., Int. Ed., 2008, 47, 7639 CrossRef CAS PubMed.
- Y. Wang and H. Yang, Synthesis of iron oxide nanorods and nanocubes in an imidazolium ionic liquid, Chem. Eng. J., 2009, 147, 71 CrossRef CAS.
- Y. J. Zhu, W. W. Wang, R. J. Qi and X. L. Hu, Microwave-assisted synthesis of single-crystalline tellurium nanorods and nanowires in ionic liquids, Angew. Chem., Int. Ed., 2004, 43, 1410 CrossRef CAS PubMed.
- M. Abo-Riya, A. H. Tantawy and W. El-Dougdoug, Synthesis and evaluation of novel cationic gemini surfactants based on Guava crude fat as petroleum-collecting and dispersing agents, J. Mol. Liq., 2016, 221, 642 CrossRef CAS.
- S. Livi, J. Duchet-Rumeau, T. N. Pham and J. F. Gerard, Synthesis and physical properties of new surfactants based on ionic liquids: Improvement of thermal stability and mechanical behaviour of high density polyethylene nanocomposites, J. Colloid Interface Sci., 2011, 354, 555 CrossRef CAS PubMed.
- M. J. Schick and E. A. Beyer, Foaming Properties of Nonionic Detergents, J. Am. Oil Chem. Soc., 1963, 40, 66 CrossRef CAS.
- S. M. Alazrak, S. Awad, A. A. Khalil and W. El-Dougdoug, Synthesis and evaluation of new cationic polymeric surfactant based on N-phthalimidomethyl methacrylate. Egypt, J. Chem., 2021, 64, 3861 Search PubMed.
- H. I. Mohamed, M. Z. Basyouni, A. A. Khalil, K. A. Hebash and A. H. Tantawy, Petroleum-dispersing and antimicrobial activity of newly synthesized polymeric surfactants tethering tetrachlorophthalimide moiety, J. Iran. Chem. Soc., 2021, 18, 265 CrossRef CAS.
- B. A. A. Bader, A. H. Tantawy, M. M. Azab and W. El-Dougdoug, Novel Synthesized Cationic Gemini Surfactants Bearing Amido Group and Their Application in Nanotechonoly, Curr. Sci. Int., 2022, 11, 184 Search PubMed.
- W. I. El-Dougdoug, A. S. Al-Gorair, A. Abou Elsaoud, H. Hawsawi, A. Elaraby, E. S. Mabrouk and M. Abdallah, Synthesis and assessment of Gemini cationic surfactants as inhibitors for corrosion of carbon steel in hydrochloric acid, Green Chem. Lett. Rev., 2022, 15, 796 CrossRef CAS.
- A. Abd-ElHamid, W. El-dougdoug, S. M. Syam, I. Aiad, S. M. Shaban and D.-H. Kim, Synthesis of gemini cationic surfactants-based pyridine Schiff base for steel corrosion and sulfate reducing bacteria mitigation, J. Mol. Liq., 2023, 369, 120890 CrossRef CAS.
- A. M. Badawi, M. A. S. Mohamed, M. Z. Mohamed and M. M. Khowdairy, Surface and antitumor activity of some novel metal-based cationic surfactants, J. Cancer Res. Ther., 2007, 3, 198 CrossRef CAS PubMed.
- N. A. Negm and S. M. Tawfik, Characterization, surface properties and biological activity of some synthesized anionic surfactants, J. Ind. Eng. Chem., 2014, 20, 4463 CrossRef CAS.
- C. Ren, F. Wang, Z. Zhang, H. Nie, N. Li and M. Cui, Synthesis, surface activity and aggregation behavior of Gemini imidazolium surfactants 1,3-bis(3-alkylimidazolium-1-yl) propane bromide, Colloids Surf., A, 2015, 467, 1 CrossRef CAS.
- N. A. Negm, A. F. El-Farargy, S. M. Tawfik, A. M. Abdelnour, H. H. Hefni and M. M. Khowdiary, Synthesis, Surface and Thermodynamic Properties of Substituted Polytriethanolamine Nonionic Surfactants, J. Surfactants Deterg., 2012, 16, 333 CrossRef.
- A. Mittiga, E. Salza, F. Sarto, M. Tucci and R. Vasanthi, Heterojunction solar cell with 2% efficiency based on a Cu2O substrate, Appl. Phys. Lett., 2006, 88, 163502 CrossRef.
- M. B. Gawande, A. Goswami, F. X. Felpin, T. Asefa, X. Huang, R. Silva, X. Zou, R. Zboril and R. S. Varma, Cu and Cu-Based Nanoparticles: Synthesis and Applications in Catalysis, Chem. Rev., 2016, 116, 3722 CrossRef CAS PubMed.
- J. C. Park, J. Kim, H. Kwon and H. Song, Gram-Scale Synthesis of Cu2O Nanocubes and Subsequent Oxidation to CuO Hollow Nanostructures for Lithium-Ion Battery Anode Materials, Adv. Mater., 2009, 21, 803 CrossRef CAS.
- L. Gou and C. J. Murphy, Solution-Phase Synthesis of Cu2O Nanocubes, Nano Lett., 2003, 3, 231 CrossRef CAS.
- M. Salavati-Niasari and F. Davar, Synthesis of copper and copper(I) oxide nanoparticles
by thermal decomposition of a new precursor, Mater. Lett., 2009, 63, 441 CrossRef CAS.
- R. V. Kumar, Y. Mastai, Y. Diamant and A. Gedanken, Sonochemical synthesis of amorphous Cu and nanocrystalline Cu2O embedded in a polyaniline matrix, J. Mater. Chem., 2001, 11, 1209 RSC.
- X. M. Sun, X. Chen, Z. X. Deng and Y. D. Li, A CTAB-assisted hydrothermal orientation growth of ZnO nanorods, Mater. Chem. Phys., 2002, 78, 99 CrossRef CAS.
- M. Kooti and L. Matouri, Fabrication of Nanosized Cuprous Oxide Using Fehling's Solution, Trans. F, 2010, 17, 73 CAS.
- A.-L. Daltin, A. Addad and J.-P. Chopart, Potentiostatic deposition and characterization of cuprous oxide films and nanowires, J. Cryst. Growth, 2005, 282, 414 CrossRef CAS.
- D. A. Firmansyah, T. Kim, S. Kim, K. Sullivan, M. R. Zachariah and D. Lee, Crystalline phase reduction of cuprous oxide (Cu2O) nanoparticles accompanied by a morphology change during ethanol-assisted spray pyrolysis, Langmuir, 2009, 25, 7063 CrossRef CAS PubMed.
- Y. Sui, Y. Zeng, W. Zheng, B. Liu, B. Zou and H. Yang, Synthesis of polyhedron hollow structure Cu2O and their gas-sensing properties, Sens. Actuators, B, 2012, 171–172, 135 CrossRef CAS.
- Y. Bai, T. Yang, Q. Gu, G. Cheng and R. Zheng, Shape control mechanism of cuprous oxide nanoparticles in aqueous colloidal solutions, Powder Technol., 2012, 227, 35 CrossRef CAS.
- M. H. Huang and C.-Y. Chiu, Achieving polyhedral nanocrystal growth with systematic shape control, J. Mater. Chem. A, 2013, 1, 8081 RSC.
|
This journal is © The Royal Society of Chemistry 2023 |
Click here to see how this site uses Cookies. View our privacy policy here.