DOI:
10.1039/D3RA04520J
(Paper)
RSC Adv., 2023,
13, 25276-25283
Alternating current properties of bulk- and nanosheet-graphitic carbon nitride compacts at elevated temperatures†
Received
7th July 2023
, Accepted 16th August 2023
First published on 23rd August 2023
Abstract
The investigations of temperature-dependent electrical properties in graphitic carbon nitride (g-C3N4) have been largely performed at/below room temperature on devices commonly fabricated by vacuum techniques, leaving the gap to further explore its behaviors at high-temperature. We reported herein the temperature dependence (400 → 35 °C) of alternating current (AC) electrical properties in bulk- and nanosheet-g-C3N4 compacts simply prepared by pelletizing the powder. The bulk sample was synthesized via the direct heating of urea, and the subsequent HNO3-assisted thermal exfoliation yielded the nanosheet counterpart. Their thermal stability was confirmed by variable-temperature X-ray diffraction, demonstrating reversible interlayer expansion/contraction upon heating/cooling with the thermal expansion coefficient of 2.2 × 10−5–3.1 × 10−5 K−1. It is found that bulk- and nanosheet-g-C3N4 were highly insulating (resistivity ρ ∼ 108 Ω cm unchanged with temperature), resembling layered van der Waals materials such as graphite fluoride but unlike electronically insulating oxides. Likewise, the dielectric permittivity ε′, loss tangent tan
δ, refractive index n, dielectric heating coefficient J, and attenuation coefficient α, were weakly temperature- and frequency-dependent (103–105 Hz). The experimentally determined ε′ of bulk-g-C3N4 was reasonably close to the in-plane static dielectric permittivity (8 vs. 5.1) deduced from first-principles calculation, consistent with the anisotropic structure. The nanosheet-g-C3N4 exhibited a higher ε′ ∼ 15 while keeping similar tan
δ (∼0.09) compared to the bulk counterpart, demonstrating its potential as a highly insulating, stable dielectrics at elevated temperatures.
Introduction
Layered materials have received increasing attention due to their vast potential applications especially at elevated temperatures. For example, the thermal properties of graphite dictate its use as an electrical conductor, a thermal insulator, an inert coating/crucible material, and as a component of nuclear reactors.1–3 Hexagonal boron nitride (hBN) possesses high thermal conductivity significantly larger than that currently used in thermal management.4 Graphite fluoride is a hydrophobic, electrically insulating, solid lubricant which starts to decompose at a temperature as high as 420 °C.5 Accordingly, it is important to uncover chemical/physical properties of these layered materials, including the nanosheets derived therefrom, to serve as guidelines for materials selection.
Graphitic carbon nitride (g-C3N4) are defect-rich, polymeric aromatic layered materials consisting mainly of sp2-bonded C and N atoms into neutral layers of tri-s-triazine units.6 The layers are linked by van der Waals interactions, adopting the A–B stacking and a misalignment to decrease the repulsive π–π interactions. g-C3N4 can be exfoliated into nanosheets by a facile HNO3-assisted thermal exfoliation,7 and further constructed into a composite with metal oxide8 or metal.9 The electrical properties of g-C3N4-based devices10–16 (typically prepared by vacuum techniques) or composites8,17–20 (where the tested specimens can be easily prepared by pelletizing the powder) have been widely investigated at/below room temperature. It is acknowledged that g-C3N4 is highly insulating (resistivity ρ larger than 108 Ω cm),11,14,17,21 with the in-plane electrical conductivity two orders of magnitude smaller than that out-of-plane through the stacked layers.22 The dielectric permittivity ε′ of g-C3N4 typically varies from 2–7 in thin films14,23,24 up to ∼60 in the bulk.18,19 However, unusually large ε′ (∼103) has also been reported9 especially at low frequency (<102 Hz) where electrode polarization could occur.
Interestingly, the investigations on the charge/dipole conduction of g-C3N4 at elevated temperatures are rather limited, or the temperature range is narrow and skewed towards the low-temperature side (i.e., below RT). For example, Patra and Mohapatra15 investigated the dielectric properties of the g-C3N4/Al2O3 heterostructure at 105 Hz, showing that the capacitance (which is proportional to ε′) increased as T increased from −93 to 25 °C. Meanwhile, ε′ of g-C3N4 was practically T-independent from −73 to 23 °C,14 but it decreased from 16 to 10 as the frequency f increased (102 → 106 Hz). The electric field-dependence of conductance25,26 provided similar results. In the metal/amorphous carbon nitride/metal devices,27,28 the conductance varied with T−1/4 from −183 to 27 °C. (A similar T−1/4 dependence was reported in a four-point probe measurement,29 revealing that the charge transport was dominated by variable-hopping conduction). In addition, the conductance increased with f (101–106 Hz) at −173 °C, but it was f-independent at 23 °C. In these works, impedance spectroscopy30,31 is an important tool to elucidate the nature of charge transport, since different presentations highlight distinct parts of the responses and provide complementary information. In addition, impedance spectroscopy enables the simple derivation of some other parameters such as refractive index,32 dielectric heating coefficient,32 and attenuation coefficient,33 but these have not been performed on g-C3N4.
Herein, we reported the impedance measurements and deduced several AC properties of polycrystalline g-C3N4 in bulk and nanosheet forms from 400 → 35 °C and at f = 105, 104, and 103 Hz. The results are compared to graphite fluoride5 which is selected because it is a readily available, highly insulating, carbon-based, heteroatom-substituted layered material, where the layers are linked by van der Waals forces as with g-C3N4. In addition, we have theoretically investigated the complex dielectric function via first-principles calculation, supplementing the recent computational works.34–40 Due to the anisotropic nature, values from the in-plane and out-of-plane direction (i.e. along the stacks) were typically reported, although the direct comparison with experimental values were not straightforward because of the difference in temperature and in frequency. Our work suggested that g-C3N4 could be a promising candidate as a thermally stable, temperature-independent insulator and dielectric (ε′ ∼8 and 15 for bulk and nanosheet forms, respectively, with similar tan
δ ∼0.09).
Experimental
Synthesis
Bulk graphitic carbon nitride was prepared by a direct heating of urea powder (125 g) in an alumina crucible with a cover at 600 °C (heating rate 10 °C min−1) for 4 h.7,8 After cooling down to room temperature (RT), the pale-yellow product was collected and ground into powder. The nanosheet analog was produced by immersing the bulk powder (2.5 g) in 100 mL of HNO3 (65%) for 12 h. The obtained sample was filtered and washed with deionized water, and heated at 500 °C for 4 h. Graphite fluoride (>61 wt% F) was a product from Sigma Aldrich, and its basic characterization results can be found in Fig. S1 in ESI.†
Characterization
X-Ray diffraction (XRD) was performed using CuKα radiation at 2θ = 10–60° (Bruker model D2 PHASER). The morphology of the samples was characterized by transmission electron microscopy at 200 kV (JEOL, JEM 2010). FTIR spectra in the attenuated total reflectance (ATR) mode were collected using a PerkinElmer spectrometer (Spectrum Two) at the resolution of 4 cm−1. 1H solid state NMR spectra were acquired using a JEOL 400YH operating at 400 MHz. TGA was performed under the flow of N2 gas (20 mL min−1) from RT to 800 °C (10 °C min−1) using a PerkinElmer, Pyris-1 instrument.
The variable temperature X-ray diffraction was performed using a Rigaku SmartLab diffractometer operated from 25–350 °C under static air with the Bragg–Brentano geometry. The powder was directly placed onto an aluminum pan (7 × 7 mm2) placed on the heating stage, which was subsequently covered with stage cap chamber for heating (5 °C min−1). The XRD patterns were collected at 2θ = 10–50° at a scan speed of 40° (2θ)·min−1 with a 0.02° step-size.
Electrical properties measurements
The bulk or nanosheet-g-C3N4 powder, or graphite fluoride, was pressed without a binder into a pellet with the diameter of ∼0.5 cm and the thickness of ∼0.2 cm. All pellets were gold-sputtered on both sides to form electrical contacts. The impedance measurements were conducted using a precision LCR meter (HP-4284A, Hewlett-Packard, Palo Alto, CA) covering the frequency f = 20 to 106 Hz. To avoid complication due to water from the atmosphere,8 the specimens were heated to 400 °C prior to cool down to 35 °C employing the heating/cooling rates of 2 °C min−1. All electrical properties shown in this work were acquired from the cooling process 400 → 35 °C.
Computational method
The structural and electronic properties of g-C3N4 were performed based on first-principles calculation using the HSE06 hybrid functional41,42 as implemented in the Vienna ab initio simulation package (VASP).43,44 To accurately account for the van der Waals interaction, Grimme's empirical correction approach (GGA-D3)44 was also applied. The lattice parameters, electronic band structure, and the band gap are consistent with those in the literature, see Fig. S2.† The optical properties of g-C3N4 were calculated using the Random Phase Approximation (RPA) method based on the HSE06 functional with the assistance of the VASPKIT tool,45,46 employing an 18 × 18 × 1 gamma-centered k-point mesh.
Results and discussion
Samples characteristics
Fig. 1a shows the XRD patterns of bulk- and nanosheet-g-C3N4 samples which are similar to JCPDS 87-1526. They show two prominent peaks at ∼13.0° and 27.5° due to the in-plane motif (100 reflection) and the stacking of the graphitic carbon nitride sheet (002 reflection) respectively.6–8,47 TEM images in Fig. 1b and c display thin sheets typical of layered materials with the lateral dimension of ∼200 nm. The sheets are buckled, reflecting their flexibility and confirming a successful HNO3-assisted thermal exfoliation. The FTIR spectra in Fig. 1d show many characteristic peaks of g-C3N4.47 These include the aromatic C–N stretching (1231, 1314, 1473 cm−1), the C
N stretching (1536 and 1631 cm−1), and the N–H and O–H stretching (from absorbed H2O) at 3087, 3159 and 3254 cm−1. Interestingly, the breathing mode of s-triazine units shifts from 813 cm−1 in bulk-g-C3N4 to 803 cm−1 in nanosheet-g-C3N4, suggesting a slight modification to the tri-s-triazine building block.
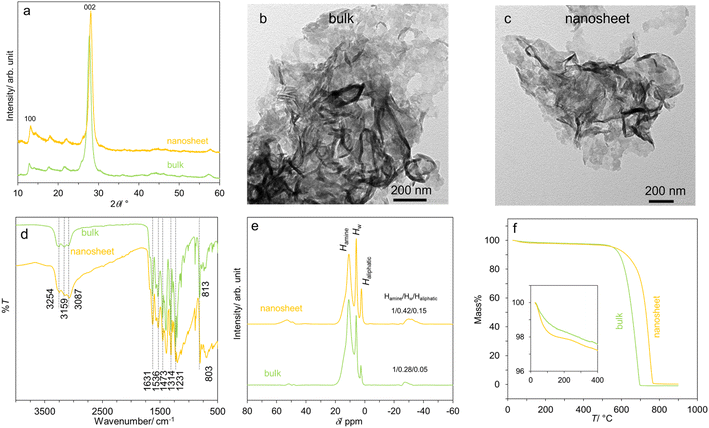 |
| Fig. 1 Some characteristics of the bulk- and nanosheet-g-C3N4 samples: (a) XRD patterns, (b and c) TEM images, (d) FTIR spectra, (e) 1H NMR spectra, and (f) mass loss curves. | |
The modification is further supported by the 1H NMR spectra in Fig. 1e, which comprise of three signals at ∼10.8, 5.8 and 2.6 ppm. There signals are ascribed respectively to the H atoms attached to N atoms from the triazine unit (Hamine), residual H2O (HW), and H attached to C atom (Haliphatic) presumably at the edge or defect.8,48,49 The peak area ratio Hamine/HW/Haliphatic for bulk-g-C3N4 equals 1/0.28/0.05, but it is 1/0.42/0.15 for nanosheet-g-C3N4. Accordingly, it can be deduced that there are more water molecules in the nanosheets, and that there is an increasing relative proportion of aliphatic region/edges in the nanosheets, consistent with the exfoliation. Lastly, Fig. 1f shows the mass loss curves. The two samples show the first mass loss due the removal of loosely bound, physisorbed water at T < 200 °C: 1.68 wt% for bulk-g-C3N4, and 2.09 wt% for nanosheet-g-C3N4. At T > 400 °C, a significant mass loss is observed possibly due to structural degradation. The hydrophilic nature of the g-C3N4 samples is consistent with earlier works on their protonic conduction8 and humidity detection.10,11,17
To further conform the thermal stability of the samples, we conducted the variable-temperature XRD measurement employing the temperature the sequence 25 → 350 → 25 °C. Fig. 2a and b focus on the interlayer distance (d002) of bulk- and nanosheet-g-C3N4 samples respectively, showing that the peak left-shifts with increasing T, reflecting the typical thermal expansion. Upon cooling down, d002 right-shifts close to the original position indicating the reversible expansion/contraction and ensuring the thermal stability within this range. This finding indicates no phase change or structural degradation at least up 350 °C. Accordingly, water (cc. Fig. 1e and f) is most likely on the surface but not intercalated into the interlayer space. The T-dependence of d002 is shown in Fig. 2c, varying from 3.193–3.202 Å, where there is no significant difference between bulk- and nanosheet-g-C3N4 samples.
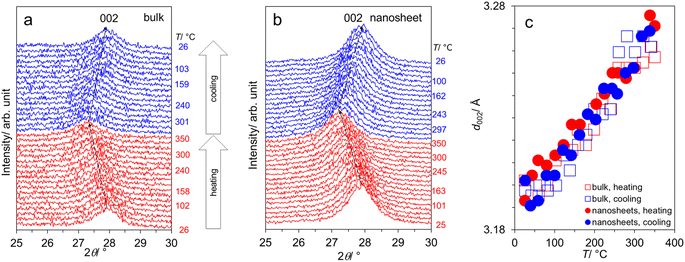 |
| Fig. 2 (a) Variable-temperature XRD patterns from the sequence 25 → 350 → 25 °C: (a) bulk-g-C3N4, (b) nanosheet-g-C3N4; and (c) the T-dependence of the interlayer spacing d002. | |
The coefficient of thermal expansion (CTE) is calculated as:
|
CTE = [((d002(350 °C) − d002(25 °C))/d002(25 °C)]/ΔT
| (1) |
where
d002(350 °C) and
d002(25 °C) are the interlayer spacing at 350 °C and 25 °C, respectively; and Δ
T is the temperature range of the measurement. It is found that CTE values are 2.2 × 10
−5-3.1 × 10
−5 K
−1 (Table S1
†). This is the same order of magnitude with the values previously reported from first principles investigations,
35 and with those experimentally obtained from amorphous carbon nitride,
50 natural graphite sheet,
51 hBN,
52 see Table S1.
†
Temperature dependence of AC properties
It is found that the Nyquist plots (real part Z′ vs. imaginary part −Z′′) of the two samples from 400 → 50 °C appeared almost as a straight line (Fig. S3†). This does not allow the fitting by equivalent circuit or a theoretical model. Considering the limited information from the f-dependence of the complex impedance, we investigated the T-dependence of AC properties from 400 → 35 °C at selected frequencies instead. As shown in Fig. 3a for the data at 105 Hz, log
ρ(T) is relatively constant throughout 400 → 50 °C for both bulk- and nanosheet-g-C3N4 samples alike. The variation of resistivity is not more than a factor of four (Fig. S4†) despite of the wide temperature window of investigation. This fluctuation can be explained by the simple powder pressing into pellets and the accompanying porosities (apparent densities 37–44% of the theoretical values). Meanwhile, the spike at <50 °C is most likely caused by proton conduction due to the (re)adsorption of atmospheric water.8 For comparison, the variation of log
ρ(T) in typical oxides such as the insulating ceramics BaTiO3–Bi1/2Na1/2TiO3 (BT-BNT)53 is up to four orders of magnitude. The stable log
ρ(T) might be common for layered van der Waals materials such as graphite3 or graphite fluoride (Fig. 3a and also in Fig. S5†) The flat log
ρ(T) of graphite fluoride might be due to the higher apparent density (71%) of the specimen. The absence of proton conduction at <50 °C can be well explained by the well-known water repellent nature of graphite fluoride.
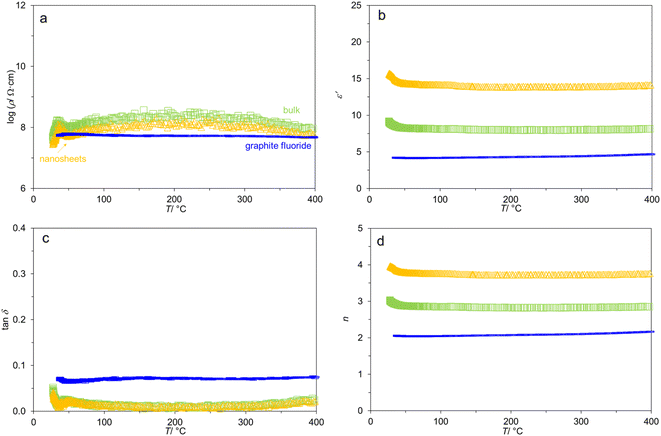 |
| Fig. 3 Temperature dependence of (a) log ρ, (b) ε′, (c) tan δ, and (d) n for bulk- and nanosheet-g-C3N4 samples, in comparison to graphite fluoride. Data shown here were at 105 Hz and were obtained in the cooling process. | |
It is observed (Fig. 3a) that log
ρ at 105 Hz is in the order: bulk-g-C3N4 (up to 8.5 Ω cm) > nanosheet-g-C3N4 (8.1 Ω cm) > graphite fluoride (7.6 Ω cm). It is known that g-C3N4 shows high in-plane resistivity but low out-of-plane resistivity.12,13,22 This is because the carriers are localized at the N atoms for the in-plane direction, but they can hop between the layers (out-of-plane), leading to the anisotropic transport characteristics.13 Accordingly, the nanosheet-g-C3N4 with smaller number of stacks should possess a facilitated charge/dipoles transport, as indeed experimentally detected by the smaller log
ρ. The different behaviors of bulk-vs. nanosheet-g-C3N4 have been observed by other techniques such as ab initio calculations37 and time resolved photoluminescence.54 This is also consistent with the modifications to the graphitic structure as shown by IR and 1H NMR spectroscopies, warranting further investigations. The different AC behavior suggests that the nanosheets would not reassemble back to the bulk form by a simple pressing during specimen preparation.
Despite of the small variation in log
ρ(T), Fig. 3b shows that ε′ is practically T-independent from 400 → 50 °C, and equals to 14 (nanosheets), 8 (bulk), and 4 (graphite fluoride). While the specimens contain porosities, the ε′ values of g-C3N4 samples are comparable to those in the literature.8,14,15,23,55 For example, Patra and Mohapatra14 reported ε′ = 12 (also at 105 Hz) in the low-temperature measurement (−3 to 23 °C). Moreover, Fig. 3c shows that the loss tangents tan
δ of the two g-C3N4 samples are similar (0.009 at 200 °C) and comparable to that in carbon nitride films on Si,23 which are seven times smaller than that of graphite fluoride. The dielectric loss is typically due to dipole relaxation (energy dissipation) and resistive loss (due to mobile charge carriers). Apparently, the latter is insignificant in nanosheet-g-C3N4. Also, the larger ε′ of the nanosheet-compared to the bulk-g-C3N4 is consistent with the larger σ (i.e., smaller ρ, Fig. 3a) and the same loss tangent (Fig. 3c), because σ = ωε′ε0
tan
δ.
The T-dependence impedance measurements allowed the calculation of some other parameters, see ESI.† Fig. 3d shows that the refractive indices n [eqn (S8)†] at 105 Hz are similarly T-independent at 3.7 (nanosheet-g-C3N4), 2.8 (bulk-g-C3N4), and 1.6 (graphite fluoride) (vs. 1.85 for amorphous carbon nitride).55 Notably, n of nanosheet-g-C3N4 is larger than that of the bulk, presumably reflecting the complex scatterings which require in-depth investigations. Nevertheless, similar finding is available56 where n of graphite (2.56) is smaller than that of graphene (2.65–2.71), although the difference is small in this case. The T-dependence of the dielectric heating coefficient32 J, and the attenuation coefficient33 α has been calculated as shown in Fig. S6.†
First-principles calculation
We also investigated the complex dielectric function (real part ε′ and the imaginary part ε'') of bulk-g-C3N4 at absolute zero temperature by first-principles calculation. Because the polarization along the x- and y-axes (i.e., in-plane) is isotropic, it is represented as a single line Exx(yy) shown by the blue line in Fig. 4a and b. The polarization along the z-axis (i.e., out of plane) is represented by the red line Ezz. The average polarization was calculated by assuming that the off-diagonal elements contribute less to the average compared to the diagonal ones: |
Eavg = 2/3(Exx + Eyy) + 1/3(Ezz)
| (2) |
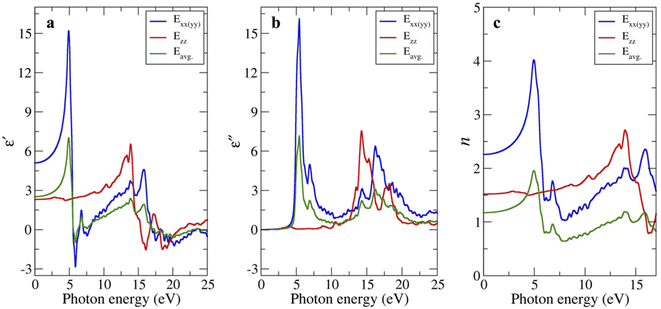 |
| Fig. 4 (a) Real and (b) imaginary parts of the complex dielectric function, and (c) refractive index of bulk-g-C3N4 as a function of photon energy. | |
The strong peak is observed for Exx(yy) at 4.9 eV, while another peak with medium intensity is observed for Ezz at 15.8 eV. The peaks positions are broadly consistent with the reports in g-C3N4 (ref. 39 and 40) or amorphous carbon.34
The static dielectric permittivity (i.e., ε′ close to zero energy) were calculated to be 5.10, 2.31, and 2.52 for the in-plane, out-of-plane, and the average value, respectively, which are comparable to 6.15, 2.84, and 5.05 reported by Rignanese et al.36 Notably, the value along the in-plane direction (which was derived theoretically at absolute zero temperature) better matched the experimentally observed value (ε′ ∼8), the latter being weakly T-dependent from 400 → 50 °C. Meanwhile, the imaginary part near zero energy is on the order of 10−2 (not clearly visible in Fig. 4b). Accordingly, the ratio of imaginary part over real part (i.e., tan
δ) will be small, qualitatively consistent with the impedance measurements.
It is natural to assume that thin sheets of layered materials would approximately (but not neatly) stack on top of each other upon pelletization. Because the in-plane resistivity is higher than the out-of-plane resistivity,12,13,22 charges/dipoles will be mostly confined to the in-plane direction, explaining to the reasonable agreement between the measurement and the calculated polarization along the xx(yy) direction. Likewise, the calculated (static) n values for the xx(yy) direction of 2.26 (vs. 2.0 by Reshak et al.)39 are closer to n = 2.8 experimentally determined from bulk-g-C3N4 (Fig. 3d). The n values of 1.52 (zz) and 1.17 (averaged) are smaller.
Frequency dependence of AC properties
Table 1 compares log
ρ, ε′, tan
δ, and n at f = 105, 104 and 103 Hz, all acquired upon cooling down (see also Fig. S7 and S8†). The values shown were averaged from 400 → 50 °C, with the standard deviation of the last digit in parenthesis. In all cases, log
ρ increases with decreasing f (i.e., the samples become more insulating). This finding agrees with Jonscher's universal power law due to interacting charges/dipoles33,57,58 (σ = Afn; ρ = 1/σ). However, ε′ increases with decreasing f because dipoles have more time to reorient in response to the alternating field.8,32,33 The increased ε′ leads to an increasing tan
δ. Interestingly, tan
δ remains similar between bulk- and nanosheet-g-C3N4 (0.01 at 105 Hz, and 0.08 at 103 Hz) while the ε′ values of the nanosheets are almost two times larger, 15 vs. 8. The results suggest that nanosheet-g-C3N4 could be a promising candidate for a highly insulating, stable dielectrics at elevated temperature.
Table 1 Comparison of the log
ρ, ε′, tan
δ, and n of the samples averaged from 400 → 50 °C (with the standard deviation of the last digit in parenthesis) at selected frequencies (in Hz), obtained during the cooling process
Sample |
f/Hz |
log(ρ/Ω cm) |
ε′ |
tan δ |
n |
Bulk-g-C3N4 |
105 |
8(1) |
8.07(9) |
0.014(5) |
2.84(2) |
104 |
8.8(1) |
8.3(2) |
0.04(1) |
2.88(3) |
103 |
9.5(2) |
8.8(5) |
0.08(3) |
2.97(9) |
Nanosheet-g-C3N4 |
105 |
8.0(1) |
14.0(2) |
0.014(4) |
3.75(2) |
104 |
8.6(1) |
14.5(3) |
0.04(1) |
3.81(4) |
103 |
9.2(2) |
15(1) |
0.08(3) |
3.9(1) |
Graphite fluoride |
105 |
7.73(3) |
4.3(1) |
0.070(3) |
2.08(3) |
104 |
8.8(1) |
4.8(1) |
0.065(7) |
2.19(3) |
103 |
9.8(2) |
5.2(3) |
0.05(2) |
2.29(6) |
Lastly, n is practically T-invariant in both g-C3N4 samples. The insignificant change of n(T) was long known in other layered materials such as graphite59 from 25 to 600 °C. When f decreased from 105 to 103 Hz, the variation with T is more pronounced with the standard deviations 0.5–0.7%, 1%, and 3% of n, comparable to that of graphite fluoride also shown in Table 1.
Conclusions
Bulk- and nanosheet-g-C3N4 were thermally stable at least up to 350 °C with reversible interlayer expansion/contraction upon heating/cooling. They exhibited relatively stable resistivity, ε′, tan
δ, and n invariant with temperature from 400 → 50 °C which were also weakly f-dependent from 105–103 Hz.
The experimental values (ε′ and n) of bulk-g-C3N4 are in reasonable agreement with the values along the in-plane direction as extracted from first-principles calculation, further showcasing the anisotropic charge/dipole transport.
Notably, nanosheet-g-C3N4 is more conducting than the bulk counterpart, in addition to exhibiting almost two time larger ε′ (15 vs. 8) but similar tan
δ (0.09). This is contributed to the facilitated charge transfer in nanosheets with decreased stack numbers, ascribed to structural modifications suggested by IR and 1H NMR spectroscopies. The nanosheet-g-C3N4 could be a promising candidate for a highly insulating, stable dielectrics at elevated temperature. The weak temperature-dependence of electrical properties, and the demonstrated structural/thermal stabilities, would minimize the impact of varying temperature on the device performances.
Author contributions
Tosapol Maluangnont: conceptualization; writing, review, and editing; funding acquisition; project administration, and supervision. Phieraya Pulphol, Kanokwan Chaithaweep, Klichchupong Dabsamut, and Thawanrat Kobkeatthawin: investigation. Siwaporn Meejoo Smith, Adisak Boonchun and Naratip Vittayakorn: supervision. All authors have reviewed and approved of the manuscript.
Conflicts of interest
There are no conflicts of interest to declare.
Acknowledgements
This work is supported by King Mongkut's Institute of Technology Ladkrabang (KREF 046403) (T. Maluangnont). The work of N. Vittayakorn is funded by KMITL under grant No. KREF 116501. We also acknowledge the facilities and technical assistance from Nanotechnology and Materials Analytical Instrument Service Unit (NMIS) of College of Materials Innovation and Technology, KMITL.
References
- D. K. L. Tsang, B. J. Marsden, S. L. Fok and G. Hall, Graphite thermal expansion relationship for different temperature ranges, Carbon, 2005, 43, 2902–2906 CrossRef CAS.
- J. B. Nelson and D. P. Riley, The thermal expansion of graphite from 15 °C to 800 °C: part I. experimental, Proc. Phys. Soc., 1945, 57, 477–486 CrossRef CAS.
- M. Okada, N. Ohta, O. Yoshimoto, M. Tatsumi and M. Inagaki, Review on the high-temperature resistance of graphite in inert atmospheres, Carbon, 2017, 116, 737–743 CrossRef CAS.
- J.-C. Zheng, L. Zhang, A. V. Kretinin, S. V. Morozov, Y. B. Wang, T. Wang, X. Li, F. Ren, J. Zhang and C.-Y. Lu, et al., High thermal conductivity of hexagonal boron nitride laminates, 2D Mater., 2016, 3, 011004 CrossRef.
- R. L. Fusaro and H. E. Sliney, Graphite fluoride (CFx)n—a new solid lubricant, ASLE Trans., 1970, 13, 56–65 CrossRef CAS.
- F. Fina, S. K. Callear, G. M. Carins and J. T. S. Irvine, Structural investigation of graphitic carbon nitride via XRD and neutron diffraction, Chem. Mater., 2015, 27, 2612–2618 CrossRef CAS.
- T. Kobkeatthawin, J. Trakulmututa, T. Amornsakchai, P. Kajitvichyanukul and S. M. Smith, Identification of active species in photodegradation of aqueous imidacloprid over g-C3N4/TiO2 Nanocomposites, Catalysts, 2022, 12, 120 CrossRef CAS.
- T. Maluangnont, P. Pulphol, S. Pongampai, T. Kobkeatthawin, S. M. Smith and N. Vittayakorn, TiO2/graphitic carbon nitride nanosheet composite with enhanced sensitivity to atmospheric water, RSC Adv., 2023, 13, 6143–6152 RSC.
- S. Khasim, A. Pasha, M. Lakshmi, C. Panneerselvam, A. A. A. Darwish, T. A. Hamdalla, S. Alfadhli and S. A. Al-Ghamdi, Conductivity and dielectric properties of heterostructures based on novel graphitic carbon nitride and silver nanoparticle composite film for electronic applications, Dig. J. Nanomater. Biostruct., 2022, 17, 1089–1098 CrossRef.
- L. M. Zambov, C. Popov, M. F. Plass, A. Bock, M. Jelinek, J. Lancok, K. Masseli and W. Kulisch, Capacitance humidity sensor with carbon nitride detecting element, Appl. Phys. A, 2000, 70, 603–606 CrossRef CAS.
- Z. Zhang, J. Huang, Q. Yuan and B. Dong, Intercalated graphitic carbon nitride: a fascinating two-dimensional nanomaterial for an ultra-sensitive humidity nanosensor, Nanoscale, 2014, 6, 9250–9256 RSC.
- N. Urakami, K. Ogihara, H. Futamura, K. Takashima and Y. Hashimoto, Demonstration of electronic devices in graphitic carbon nitride crystalline film, AIP Adv., 2021, 11, 075204 CrossRef CAS.
- K. Takashima, N. Urakami and Y. Hashimoto, Electronic transport and device application of crystalline graphitic carbon nitride film, Mater. Lett., 2020, 281, 128600 CrossRef CAS.
- P. C. Patra and Y. N. Mohapatra, Dielectric constant of thin film graphitic carbon nitride (g-C3N4) and double dielectric Al2O3/g-C3N4, Appl. Phys. Lett., 2021, 118, 103501 CrossRef CAS.
- P. C. Patra and Y. N. Mohapatra, Graphitic carbon nitride (g-C3N4)/Al2O3 heterostructure as double dielectric: a comparative study in MIS based on a-IGZO, IEEE J. Electron Devices Soc., 2021, 9, 618–622 CAS.
- M. Aono and S. Nitta, High resistivity and low dielectric constant amorphous carbon nitride films: application to low-k materials for ULSI, Diamond Relat. Mater., 2002, 11, 1219–1222 CrossRef CAS.
- X. Guo, D. Kuang, Z. Zhu, Y. Ding, L. Ge, Z. Wu, B. Du, C. Liang, G. Meng and Y. He, Humidity sensing
by graphitic carbon nitride nanosheet/TiO2 nanoparticle/Ti3C2Tx nanosheet composites for monitoring respiration and evaluating the waxing of fruits, ACS Appl. Nano Mater., 2021, 4, 11159–11167 CrossRef CAS.
- S. Pareek, M. Sharma, S. Lai and J. K. Quamara, Polymeric graphitic carbon nitride-barium titanate nanocomposites with different content ratios: a comparative investigation on dielectric and optical properties, J. Mater. Sci.: Mater. Electron., 2018, 29, 13043–13051 CrossRef CAS.
- S. Pareek and J. K. Quamara, Dielectric and optical properties of graphitic carbon nitride-titanium dioxide nanocomposite with enhanced charge separation, J. Mater. Sci., 2018, 53, 604–612 CrossRef CAS.
- S. Liu, T. Ye, Y. Liu, H. Cheng and X. Liu, Graphitic-carbon nitride nanosheets as a new inorganic filler for improving energy storage density of PVDF-based dielectric composites, J. Mater. Sci.: Mater. Electron., 2020, 31, 13063–13069 CrossRef CAS.
- X. Kang, A. Chatzitakis, T. Aarholt, X. Sun, C. Negri and T. Norby, Facet-engineered TiO2 nanomaterials reveal the role of water–oxide interactions in surface protonic conduction, J. Mater. Chem. A, 2022, 10, 218–227 RSC.
- Y. Noda, C. Merschjann, J. Tarábek, P. Amsalem, N. Koch and M. J. Bojdys, Directional charge transport in layered two-dimensional triazine-based graphitic carbon nitride, Angew. Chem., Int. Ed., 2019, 58, 9394–9398 CrossRef CAS PubMed.
- N. Konofaos, E. K. Evangelou, S. Logothetidis and M. Gioti, Electrical properties of carbon nitride films on silicon, J. Appl. Phys., 2002, 91, 9915–9918 CrossRef CAS.
- S. Pareek, S. Waheed and S. Karak, The effect of UV-ozone treatment on structural, optical, and dielectric properties of thermally evaporated graphitic carbon nitride thin film, J. Electron. Mater., 2023, 52, 1104–1112 CrossRef CAS.
- C. Godet, J. P. Kleider and A. S. Godovskikh, Electric field-controlled sign of the capacitance in metal-carbon nitride-metal devices, J. Non-Cryst. Solids, 2008, 354, 2637–2640 CrossRef CAS.
- S. Kumar, C. Godet, A. Goudovskikh, J. P. Kleider, G. Adamopoulos and V. Chu, High-field transport in amorphous carbon and carbon nitride films, J. Non-Cryst. Solids, 2004, 338–340, 349–352 CrossRef CAS.
- J. P. Kleider, A. S. Gudovskikh and C. Godet, DC and AC hopping transport in metal/amorphous carbon nitride/metal devices, J. Non-Cryst. Solids, 2006, 352, 1323–1326 CrossRef CAS.
- C. Godet, J. P. Kleider and A. S. Gudovskikh, Frequency scaling of ac hopping transport in amorphous carbon nitride, Diamond Relat. Mater., 2007, 16, 1799–1805 CrossRef CAS.
- Z. Qin, P. Wang, H. Shen, L. Mi and X. Ying, Microstructure and electronic properties of pulsed-discharge-deposited amorphous carbon-nitride films, Diamond Relat. Mater., 2015, 14, 1616–1622 CrossRef.
- J. T. S. Irvine, D. C. Sinclair and A. R. West, Electroceramics: characterization by impedance spectroscopy, Adv. Mater., 1990, 2, 132–138 CrossRef CAS.
- R. Gerhardt, Impedance and dielectric spectroscopy revisited: distinguishing localized relaxation from long-range conductivity, J. Phys. Chem. Solids, 1994, 55, 1491–1506 CrossRef CAS.
- T. Maluangnont, S. Sriphan, T. Charoonsuk and N. Vittayakorn, Dielectric spectroscopy and electric modulus analyses of Ti0.8O2 nanosheets–Ag nanoparticles–cellulose filter paper composites, Integr. Ferroelectr., 2022, 224, 214–224 CrossRef CAS.
- S. Sriphan, P. Pulphol, T. Charoonsuk, T. Maluangnont and N. Vittayakorn, Effect of adsorbed water and temperature on the universal power law behavior of lepidocrocite-type alkali titanate ceramics, J. Phys. Chem. C, 2021, 125, 12910–12920 CrossRef CAS.
- M. Gioti and S. Logothetidis, Dielectric function, electronic properties and optical constants of amorphous carbon and carbon nitride films, Diamond Relat. Mater., 2003, 12, 957–962 CrossRef CAS.
- S. P. Sun, S. Gu, J. H. Sun, F. F. Xia and G. H. Chen, First principles investigation of the electronic properties of graphitic carbon nitride with different building block and sheet staggered arrangement, J. Alloys Compd., 2018, 735, 131–139 CrossRef CAS.
- G.-M. Rignanese, J.-C. Charlier and X. Gonze, First-principles study of vibrational and dielectric properties of C3N4 polymorphs, Phys. Rev. B, 2002, 66, 205416 CrossRef.
- S. Agrawal, W. Lin, O. V. Prezhdo and D. J. Trivedi, Ab initio quantum dynamics of charge carriers in graphitic carbon nitride nanosheets, J. Chem. Phys., 2020, 153, 054701 CrossRef CAS PubMed.
- B. Zhu, B. Cheng, L. Zhang and J. Yu, Review on DFT calculation of s-triazine-based carbon nitride, Carbon Energy, 2019, 1, 32–56 CrossRef CAS.
- A. H. Reshak, S. A. Khan and S. Auluck, Linear and nonlinear optical properties for AA and AB stacking of carbon nitride polymorph (C3N4), RSC Adv., 2014, 4, 11967–11974 RSC.
- L.-W. Ruan, Y.-J. Zhu, L.-G. Qiu, Y.-P. Yuan and Y.-X. Lu, First principles calculations of the pressure affection to g-C3N4, Comput. Mater. Sci., 2014, 91, 258–265 CrossRef CAS.
- J. Heyd, G. E. Scuseria and M. Ernzerhof, Hybrid functionals based on a screened Coulomb potential, J. Chem. Phys., 2003, 118, 8207–8215 CrossRef CAS.
- J. Heyd, G. E. Scuseria and M. Ernzerhof, Erratum: “Hybrid functionals based on a screened Coulomb potential” [J. Chem. Phys., 118, 8207, 2003], J. Chem. Phys., 2006, 124, 219906 CrossRef.
- G. Kresse and J. Furthmüller, Efficient iterative schemes for ab initio total-energy calculations using a plane-wave basis set, Phys. Rev. B, 1996, 54, 11169–11186 CrossRef CAS PubMed.
- S. Grimme, J. Antony, S. Ehrlich and H. Krieg, A consistent and accurate ab initio parametrization of density functional dispersion correction (DFT-D) for the 94 elements H-Pu, J. Chem. Phys., 2010, 132, 154104 CrossRef PubMed.
- V. Wang, N. Xu, J.-C. Liu, G. Tang and W.-T. Geng, VASPKIT: A user-friendly interface facilitating high-throughput computing and analysis using VASP code, Comput. Phys. Commun., 2021, 267, 108033 CrossRef CAS.
- K. Dabsamut, I. Chatratin, T. Thanasarnsurapong, T. Maluangnont and A. Boonchun, Theoretically proposed stable polymorph of two-dimensional pentagonal β-PdPSe, Phys. Chem. Chem. Phys., 2023, 25, 3815–3819 RSC.
- T. Narkbuakaew and P. Sujaridworakun, Synthesis of tri-s-triazine based g-C3N4 photocatalyst for cationic rhodamine B degradation under visible light, Top. Catal., 2020, 63, 1086–1096 CrossRef CAS.
- P. Zhang, Y. Tong, Y. Liu, J. J. M. Vequizo, H. Sun, C. Yang, A. Yamakata, F. Fan, W. Lin and X. Wang, et al., Heteroatom dopants promote two-electron O2 reduction for photocatalytic production of H2O2 on polymeric carbon nitride, Angew. Chem., Int. Ed., 2020, 59, 16209–16217 CrossRef CAS PubMed.
- W. Li, Z. Guo, L. Jiang, L. Zhong, G. Li, J. Zhang, K. Fan, S. Gonzalez-Cortes, K. Jin and C. Xu, et al., Facile in situ reductive synthesis of both nitrogen deficient and protonated g-C3N4 nanosheets for the synergistic enhancement of visible-light H2 evolution, Chem. Sci., 2020, 11, 2716–2728 RSC.
- A. Champi, R. G. Lacerda and F. C. Marques, Thermal expansion coefficient of amorphous carbon nitride thin films deposited by glow discharge, Thin Solid Films, 2002, 420–421, 200–204 CrossRef CAS.
- M. Cermak, N. Perez, M. Collins and M. Bahrami, Material properties and structure of natural graphite sheet, Sci. Rep., 2020, 10, 18672 CrossRef CAS PubMed.
- W. Paszkowicz, J. B. Pelka, M. Knapp, T. Szyszko and S. Podsiadlo, Lattice parameters and anisotropic thermal expansion of
hexagonal boron nitride in the 10–297.5 K temperature range, Appl. Phys. A, 2002, 75, 431–435 CrossRef CAS.
- T. Shimada, K. Touji, Y. Katsuyama, H. Takeda and T. Shiosaki, Lead free PTCR ceramics and its electrical properties, J. Am. Ceram. Soc., 2007, 27, 3877–3882 CAS.
- P. C. Patra and Y. N. Mohapatra, Exfoliated and evaporated thin films of graphitic carbon nitride (g-C3N4): evolution of photoelectronic properties from bulk, Mater. Lett., 2021, 302, 130374 CrossRef CAS.
- M. Aono, Y. Naruse, S. Nitta and T. Katsuno, The effect of hydrogen- and oxygen-plasma treatments on dielectric properties of amorphous carbon nitride films, Diamond Relat. Mater., 2001, 10, 1147–1151 CrossRef CAS.
- S. Cheon, K. D. Kihm, H. G. Kim, G. Lim, J. S. Park and J. S. Lee, How to reliably determine the complex r efractive Index (RI) of graphene by using two independent measurement constraints, Sci. Rep., 2014, 4, 6364 CrossRef CAS PubMed.
- A. K. Jonscher, Dielectric relaxation in solids, J. Phys. D: Appl. Phys., 1999, 32, R57–R70 CrossRef CAS.
- A. K. Jonscher, The 'universal' dielectric response, Nature, 1977, 267, 673–679 CrossRef CAS.
- B. J. Stagg and T. T. Charalampopoulos, Refractive indices of pyrolytic graphite, amorphous carbon, and flame soot in the temperature range 25° to 600 °C, Combust. Flame, 1993, 94, 381–396 CrossRef CAS.
|
This journal is © The Royal Society of Chemistry 2023 |
Click here to see how this site uses Cookies. View our privacy policy here.