DOI:
10.1039/D3RA04249A
(Paper)
RSC Adv., 2023,
13, 24777-24788
Construction of an optical sensor for copper determination in environmental, food, and biological samples based on the covalently immobilized 2-(2-benzothiazolylazo)-3-hydroxyphenol in agarose
Received
25th June 2023
, Accepted 6th August 2023
First published on 18th August 2023
Abstract
An optical chemical sensor has been developed for the quantitative spectrophotometric analysis of copper. The optode is dependent on covalent immobilization of 2-(2-benzothiazolylazo)-3-hydroxyphenol (BTAHP) in a transparent agarose membrane. The absorbance variation of immobilized BTAHP on agarose as a film upon the addition of 5 × 10−3 M aqueous solutions of Mn2+, Zn2+, Hg2+, Cd2+, Pb2+, Co2+, Ni2+, Fe2+, La3+, Fe3+, Cr3+, Zr4+, Se4+, Th4+, and UO22+ revealed substantially higher changes in the Cu2+ ion content compared to other ions investigated here. The effects of various experimental parameters, such as the solution pH, the reaction time, and the concentration of reagents, on the quality of Cu2+ sensing were examined. Under ideal experimental circumstances, a linear response was achieved for Cu2+ concentrations ranging from 1.0 × 10−9 to 7.5 × 10−6 M with an R2 value of 0.9988. The detection (3σ) and quantification (10σ) limits of the procedure for Cu2+ analyses were 3.0 × 10−10 and 9.8 × 10−10 M, respectively. No observable interference was recorded in the detection of Cu2+ due to other inorganic cations. With no indication of BTAHP leaching, the membrane demonstrated good durability and quick response times. The optode was effectively used to determine the presence of Cu2+ in environmental water, food, and biological samples.
Introduction
Heavy metal pollution is a severe global environmental problem due to its impacts, such as genetic alteration in all living things. Currently, efforts to eliminate this hazardous material have accelerated. Technology advancement and rapid industrialization have enhanced the need for high-purity materials. Trace element measurements are becoming much more crucial because of how such elements affect human metabolism.1
The most important trace element in the human body, i.e., copper, comes in third place in terms of abundance.2 In actuality, the liver comes in second place to the brain in terms of copper content.3 This abundance is attributable to the important functions that copper ions play in a wide range of biological activities, including neuromodulation, antioxidative defense, and response to hypoxia.4
Due to its redox-active nature, copper is a well-known important trace mineral for organisms and is crucial for many key physiological processes in the human body.5–9 Cu2+ is frequently utilized as an auxiliary ion in reactions that are catalyzed by enzymes because of its powerful coordination ability. The unsuitable concentration of Cu2+ in the body, however, is the cause of several diseases, including Wilson's, Alzheimer's, Menkes, Prion, and Huntington's diseases, etc. Cu2+ is second only to mercury in drinking water in terms of toxicity.10–17
The accumulation of copper ions occurs when people and animals are exposed to excessive amounts of Cu2+-contaminated water, and thus, it is hazardous to the environment and ecosystem and can also affect the progression of diseases, such as Alzheimer's and Parkinson's.10,18,19 Additionally, Cu2+, which has a very harmful effect on aquatic creatures, is a frequent environmental pollutant in aquaculture systems.20 A tolerable amount of Cu2+ in drinking water has been determined to be between 25 and 31.5 μM by the World Health Organization (WHO) and the U.S. Environmental Protection Agency (EPA), respectively.21 Consequently, simple, practical, and affordable Cu2+ sensor technologies must be developed to successfully detect and monitor its content in drinking water, food, industrial processes, and biological systems. This will help overcome the problem of Cu2+ playing a crucial role in both the environment and the human body. Developing colorimetric sensors for the detection of Cu2+ is particularly important.
To detect Cu2+ ions at a trace level, several instrumental analytical chemistry methods can be utilized in the lab, such as spectrophotometry,22 fluorometry,23,24 atomic absorption spectroscopy (AAS),25–28 inductively coupled plasma-atomic emission spectroscopy (ICP-AES),29–32 inductively coupled plasma-mass spectrometry (ICP-MS),33,34 laser-induced breakdown spectroscopy (LIBS),35 and anodic stripping voltammetry.36–38 These instrumental methods have some limitations despite having a good limit of detection (LOD) and a wider working concentration range, such as a long and tedious analysis time, the need for expensive and sophisticated equipment, a difficult sample pre-treatment procedure, and unreliable capabilities for in situ analysis.39–42 Therefore, the need to develop portable, user-friendly tools for efficient and precise screening of Cu2+ ions has gained increasing interest. To determine the presence of the Cu2+ ion using electrochemical methods, such as potentiometric and amperometric methods, selective electrodes are required to eliminate the possibility of interference from other ions.43,44 On the other hand, chemical sensors based on optical transducers, for example, UV-Vis spectrophotometers have shown the potential to achieve miniaturized devices for various analytical applications, while featuring low manufacturing costs.45,46 However, to prevent background interferences, UV-Vis spectrophotometric techniques necessitate complex sample pre-treatment and call for the employment of various standard chemicals for color transformations.43,44 In contrast, an optical chemical sensor promises cheap, reagent-less, and quick experimentation and suffers from less interference during analysis.45
Due to the fact that heavy metal ions are harmful to the environment and vital biological processes, there has been a lot of interest in the development of optical sensors for their detection during the past few decades.47–53
Optical sensors are a crucial class of chemical sensors that can track a physical parameter related to a compound's concentration over time. They offer the benefits of straightforward construction, excellent sensitivity and selectivity, tightly regulated experimental settings, and simple sample processing.54,55 Sensors are employed in conjunction with a low-cost spectrophotometric technique to give quick and easy measurement methods with outstanding selectivity and low detection limits.23,56
Reagent immobilization is a crucial step in the fabrication of optical sensors. The immobilization of the sensors' indicators can be accomplished using a variety of chemical and physical techniques. Reagent immobilization is accomplished by physical entrapment,57 sol–gel,58,59 multilayered films,60 or chemical (covalent) bonding methods.61 Ionophores are crucial components in the design of sensors. As they can form stable complexes with transition metal ions, compounds with different donor atoms are commonly utilized as ionophores in the construction of sensors. For a particular ion, they produce observable sensitivity, selectivity, and stability.54
A brief summary of data, including reagents, linear dynamic range, and detection limit of various copper sensors is provided in Table 1. The majority of these copper sensors clearly have one or more of the following drawbacks: a narrow working concentration range, high detection limits, significant cation interference, and irreversible response.
Table 1 Some optical sensors reported for the determination of Cu2+ ions
Reagent |
Measured signal |
Linear dynamic range (M) |
Detection limit (M) |
Ref. |
Fast sulphon black F |
Reflectance |
7.9 × 10−5 to 1.6 × 10−3 |
2.2 × 10−5 |
62 |
Lucifer yellow |
Fluorescence |
5.0 × 10−8 to 1.0 × 10−4 |
2.6 × 10−8 |
63 |
1-(2-Pyridylazo)-2-naphthol |
Absorbance |
6.3 × 10−7 to 1.0 × 10−4 |
3.3 × 10−7 |
64 |
(E)-N′-(Pyridin-2-ylmethyl-ene)isonicotin-ohydrazide |
|
1.3 × 10−5 to 8.3 × 10−7 |
1.9 × 10−7 |
65 |
2,2′-Dipyridylamine |
Absorbance |
7.5 × 10−6 to 2.0 × 10−4 |
8.0 × 10−7 |
66 |
1-(2-Pyridylazo)-2-naphthol |
Absorbance |
8.0 × 10−6 to 1.3 × 10−4 |
4.0 × 10−7 |
67 |
Zincon |
Absorbance |
1.6 × 10−6 to 4.7 × 10−5 |
1.6 × 10−6 |
68 |
3-(2-Methyl-2,3-dihydro-1,3-benzothiezol-2-yl)-2H-chromen-2-one |
Absorbance |
7.0 × 10−7 to 1.0 × 10−4 |
3.0 × 10−7 |
69 |
L-Cysteine-capped ZnS quantum dots |
Fluorescence |
0 to 260 × 10−6 |
7.1 × 10−6 |
70 |
DBzDA18C6/PAN |
Absorbance |
5.0 × 10−7 to 5.0 × 10−5 |
3.2 × 10−5 |
71 |
Pyrocatechol violet |
Absorbance |
1.0 × 10−5 to 1.0 × 10−4 |
— |
72 |
6-Bromo-3-(2-methyl-2,3-dihydrobenzo[d]thiazol-2-yl)-2H-chromen-2 one |
Absorbance |
7.0 × 10−7 to 1.0 × 10−4 |
2.5 × 10−7 |
23 |
Pyridine-thiourea/PVC |
Absorbance |
2.0 × 10−4 to 10 × 10−4 |
1.3 × 10−5 |
73 |
Coumarine/triacetylcellulose |
Absorbance |
8.0 × 10−7 to 1.0 × 10−4 |
2.5 × 10−7 |
23 |
Thiosemicarbazone/triacetylcellulose |
Absorbance |
7.5 × 10−6 to 2.0 × 10−4 |
8.0 × 10−8 |
74 |
2-(2-Benzothiazolylazo)-3-hydroxyphenol |
Absorbance |
1.0 × 10−9 to 7.5 × 10−6 |
3.0 × 10−10 |
This work |
Sensors for the selective detection of Cu2+ generally have structures of chelating metal ions with heteroatoms (nitrogen and oxygen/sulfur), such as naphthalimide,75,76 naphthalene,77 anthracene,78 anthraquinone,79 quinoline,80,81 and macrocyclic82,83 derivatives. However, many of them are obtained via complex synthesis procedures or require expensive materials. Some Cu2+ sensors based on liquid or solid-state membranes have been reported in literature,23,65,69,84,85 but they generally have insufficient detection limits, lack sufficient selectivity for environmental sensing, or suffer from reagent leaching problems.
The present work demonstrates the preparation and response of a new highly sensitive copper-optode based on covalent immobilization of 2-(2-benzothiazolylazo)-3-hydroxyphenol (BTAHP), which is a Cu2+ sensing molecule, on agarose membrane. The low detection limit of the proposed optode allows direct determination of Cu2+ in environmental and biological samples without any preconcentration.
Experimental
Reagents
All of the chemicals used were of analytical grade (Merck). Bi-distilled water was used throughout. The stock/standard Cu2+ ion solution was prepared by dissolving 0.1278 g of CuCl2·2H2O in bi-distilled water and diluting it to 250 mL. The solution was standardized via titration by a known method.86 The method basically depends on the titration of evolving iodine in the presence of starch by the reduction of Cu2+ to Cu+ in the mixtures containing iodide and copper(II) salts. The working standard solutions were prepared by suitable dilution of the stock solution.
Synthesis and purification of the reagent (chemically named 2-(2-benzothiazolylazo)-3-hydroxyphenol) was performed as reported previously.87 A solution of 5 × 10−3 M was prepared by dissolving the required weight in ethanol (Merck, Darmstadt, Germany). To hold the agarose membranes within the spectrophotometer quartz cells for absorbance assessments, a homemade polyacrylamide holder was applied. Test samples were buffered in a 0.02 M solution of phosphoric acid/sodium phosphate88 and pH was adjusted with dropwise addition of 1.0 M solutions of HCl or NaOH.
Apparatus
The spectroscopic assessments were achieved using a JASCO 530V UV-Vis spectrophotometer. The Cu2+ ion detection was evaluated by ICP-AES (PerkinElmer, Germany, 8300). A pH-meter of type Jenway 3505 pH meters 9 V-AC power was employed to record the pH values. The FT-IR spectrum was recorded in KBr pellets on a Shimadzu FT-IR 8101 PC infrared spectrometer in the range of 4000 to 500 cm−1. The thin films were positioned in a quartz cuvette and all absorption investigations were accomplished at 25 ± 2 °C in the batch mode. A homemade polyacrylamide holder was used to hold the agarose membranes inside the quartz cells of the spectrophotometer. A total glass Fisons (UK) double distiller was used to prepare the doubly distilled water.
Procedures
Immobilization procedure. For the preparation of transparent agarose membranes, a method that has been reported elsewhere9 was used with some modifications. A weighed amount, i.e., 0.4 g, of agarose powder was mixed with 20 mL of bi-distilled water. To achieve a viscose solution, the mixture was stirred at 90 °C until its volume decreased to 15 mL. The resulting solution was heated at 75 °C until a bubble-free solution was achieved. The final transparent and viscose solution was dispersed between two 20 cm × 20 cm dust-free glass plates with gentle pressure. The borders of one of the glass plates were already lined with a 0.25 mm thickness tape to adjust the thickness of the membrane. After cooling, the solidified membrane was cut into appropriate pieces and stored at 4 °C with 50% ethanol solution. For the epoxy activation, an epichlorohydrine method89 was used.For the immobilization of BTAHP on the activated agarose membranes, the membranes were treated with a 5 × 10−3 M BTAHP solution in a sodium phosphate buffer medium with a method reported elsewhere.90 The resulting red-colored membranes were thoroughly washed with water on a glass filter, soaked in water overnight, and washed again with a large volume of water to displace any non-bond BTAHP.
Measurement procedure. For spectrophotometric titrations, 2.0 mL of the BTAHP solution (5 × 10−3 M) in water was transferred into a quartz cell of the spectrometer. Microliter volumes of 10−4 M solutions of each metal ion were transferred to the BTAHP solution, using a 10 μL Hamilton microsyringe, followed by absorbance readings at 463 nm after vigorous shaking of the cell. All the absorbance data were corrected for the dilution.A 1 cm × 2 cm piece of the prepared agarose optode membrane was mounted in a polyacrylamide holder and placed inside the quartz cell of the spectrometer. The absorbance readings were performed against a blank cell containing a non-activated agarose membrane. For the study of the effects of different parameters on the response of the optical membrane, absorbance measurements at 474 nm were performed in an aqueous medium. The calibration curve was accomplished by plotting the absorbance of a series of Cu2+ standard solutions at various concentrations. The Cu2+ concentration that existed in the sample can be assessed by applying a calibration curve. The optode was regenerated for 5.0 min in a 0.05 M EDTA solution and was then ready to use.
Applications
Analysis of water samples. The proposed sensor was found to work well under laboratory conditions. The Cu2+ sensor was used for the determination of Cu2+ in some natural water samples. Water samples were collected from a local pipe, river water, and well water (Benha, Egypt). All water samples were filtered through a packed filter to remove particulate impurities, and the pH was adjusted to pH 8.75 by using a buffer solution. This analysis was carried out on 50 mL samples of water following the spiking of the samples with Cu2+ ion to make solutions ranging from 65–200 ng mL−1 CuCl2. The results were compared with those obtained using the ICP-AES method.
Preparation of real foodstuff samples. In order to demonstrate the applicability and reliability of the proposed copper sensor for some real foodstuff samples, black tea, coffee, milk powder, black currant, and sour cherry juice were prepared and analyzed by the suggested method.Tea and coffee were prepared as follows: the tea or coffee sample (about 1.0 g) was placed in a beaker followed by the addition of 10 mL of HCl and 4.0 mL of HClO4. The sample was then digested until the organic residue was completely oxidized. The solution was finally diluted to 100 mL in a standard flask after filtering it through a Whatman filter paper.
The preparation of the black currant and sour cherry juice was performed as follows: the samples were air-dried in porcelain crucibles using an oven at 105 °C for 24 h. Known amounts of dried samples were completely burned in an electric furnace at 500 ± 20 °C. The ashes thus obtained were treated with diluted HCl dissolution.
For analysis of milk samples, about 2.0 g of milk powder was converted to ash at 400 °C in a crucible for 3.0 h. Afterward, 2.0 mL of concentrated HCl was added, and the mixture was heated to dissolve the residue. The pH of the resulting solution was adjusted at optimal value and diluted with bi-distilled water in a 25.0 mL volumetric flask.91
Analysis of soil sample. The homogenized soil sample (20 g) was weighed accurately; in a 200 mL beaker, it was digested in the presence of an oxidizing agent with the addition of 10 mL of concentrated HNO3 and 2.0 mL of 70% HClO4 with heating for 1.0 h. The content of the beaker was filtered through a Whatman no. 40 filter paper into a 250 mL measuring flask; pH was adjusted to the desired value and diluted to mark with bi-distilled water. The general procedure was also applied. Copper levels in the final solutions were determined by ICP-AES using the aforementioned general procedures.
Analysis of vegetable sample. The spinach sample was purchased from Benha, Egypt. Afterward, it was taken in a small mesh. For the digestion of the sample, the procedure described in a previous study92 was applied. Forty grams of spinach sample was heated in a silica crucible for 3.0 h on a hot plate, and the charred material was transferred to a furnace for overnight heating at 650 °C. The residue was cooled, and treated with 10 mL of concentrated nitric acid and 3.0 mL 30% H2O2; it was again kept in a furnace for 2.0 h at the same temperature so that no organic compound traces were left. The final residue was treated with 3.0 mL of concentrated hydrochloric acid and 2.0–4.0 mL of 70% perchloric acid and evaporated to fumes; the goal was to ensure that all the metals changed to their respective ions. The solid residue was dissolved in water, filtered, and while maintaining the pH at 8.75, the total volume was made up to 25 mL by the addition of buffer solution. The dissolved solution was suitably diluted and the presented procedure was applied.
Analysis of biological samples. Fifty grams of liver or meat were taken and dried for 48 h in an oven at 120 °C to remove the water content and to obtain a constant weight (about 68% of the sample was water). The dried liver or meat sample was transferred into a glass flask. For the digestion of the sample, the procedure given by Ghaedi et al.92 was applied, and the samples were treated.93,94 A concentrated acid mixture of 3.0 mL H2SO4, 15 mL of HClO4, and 15 mL of HNO3 was added, and the solution was left to stand overnight. The solution was kept in an oil bath at 50 °C until the foaming stopped. The temperature was then increased to 150 °C, and heating was continued until the evolution of the brown fumes of nitrogen oxides ceased. When a dark brown tinge appeared in the mixture, the flask was cooled for about 2.0 min; five milliliters of nitric acid was then added. Heating was continued until the nitrogen oxide fumes were no longer given off. The appearance of white fumes of perchloric acid in 1.0 mL of the solution is an indication of complete digestion. The solid residue was dissolved in water and filtered; while maintaining the pH at 8.75, the volume was made up to 25 mL by the addition of buffer. The sensor procedure was applied, and the copper levels in the final solutions were determined by ICP-AES and the abovementioned general procedures.
Accuracy and precision
Six solutions of various concentrations of Cu2+ were analyzed to assess the precision and accuracy of the proposed method. Determination of the repeatability (intra-assay) and evaluation of the intermediate precision (inter-assay) were determined over five non-consecutive days; it depended on the relative standard deviation (RSD) percentage that was assessed within the same day, and the six replicates which were evaluated by the assay procedure.
Results and discussion
Preliminary studies
Fig. 1 displays the absorption spectra of BTAHP and the Cu–BTAHP complex solutions, providing valuable insights into their respective maximum absorption wavelengths. Notably, BTAHP exhibits peak absorption at 458 nm, while the Cu–BTAHP complex demonstrates a distinct absorption band at 468 nm. For Cu–BTAHP, the sensor presents an absorbance maximum of ∼474 nm. By increasing the addition of Cu2+, a decrease in the absorbance is observed at this wavelength. For most of the other metal ions studied, i.e., Mn2+, Zn2+, Hg2+, Cd2+, Pb2+, Co2+, Ni2+, Fe2+, La3+, Fe3+, Cr3+, Zr4+, Se4+, Th4+, and UO22+, only negligible or small variations in the absorbance maximum were obtained by altering the metal ion/BTAHP mole ratio. Based on the high selectivity of BTAHP for Cu2+ and a distinct color change (decrease) that was observed for the formation of its complex with Cu2+, BTAHP was expected to act as a suitable reagent for the preparation of the optode for Cu2+ ion (Scheme 1).
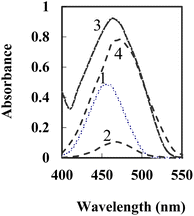 |
| Fig. 1 Absorption spectra of 1-BTAHP in solution; 2-BTAHP–Cu (5 × 10−4 M) in solution and 3-BTAHP optode 4-BTAHP–Cu optode at 5 × 10−7 M Cu2+ on the sensor under optimum conditions. | |
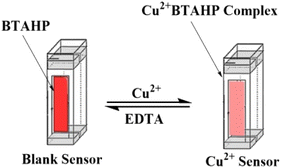 |
| Scheme 1 Representative diagram of the preparation of the Cu2+-sensing optode. | |
Preparation of the optode
For the preparation of the Cu2+-sensitive optode, BTAHP was loaded on the transparent agarose membrane. Immobilization of BTAHP on agarose membranes changed its optical properties to some extent. As depicted in Fig. 1, the absorbance maximum of the BTAHP membrane showed a red shift from 463 to 474 nm, which was probably due to the flatter structure of the immobilized reagent that may have caused an easier electron resonance. Similar shifts have been reported for other agarose optical membranes.95–97
Effect of pH on the test solution
In order to obtain an appropriate BTAHP loading on the agarose membrane, experimental conditions were optimized for immobilization. The effect of pH was studied, which showed that a maximum immobilization of BTAHP was achieved at the pH of ∼8.75 as recorded in Fig. 2.
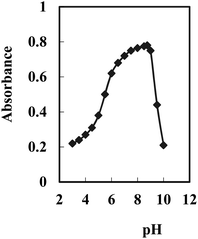 |
| Fig. 2 Effect of pH on the optode of 5 × 10−7 M Cu2+ complexed with BTAHP under optimum conditions. | |
A study of the effects of BTAHP concentration on its immobilization on the membrane indicated a continuous increase in the membrane absorbance by an increase in the BTAHP concentration from 2.5 × 10−6 to 5 × 10−2 M. High loadings, however, could unacceptably decrease the transmittance of the membrane. Therefore, a BTAHP concentration of 5 × 10−3 M, resulting in a maximum absorbance of about 0.73, was used in subsequent experiments.
BTAHP contains an azo group, and it shows color changes with pH variation as reported elsewhere.87 Therefore, the response characteristics of the membrane prepared for Cu2+ determination were studied as a function of pH. It seemed more appropriate to use ΔA (the difference in absorbance before and after the addition of Cu2+) rather than the absolute value of A in this investigation. The difference in absorbance increased rapidly from 4.5 to 7.5, then slightly increased till it became pH 8.75; it started to decrease from ∼pH 9.0 and decreased rapidly at higher pH values. Therefore, a pH of 8.75 was considered to be optimum and was used for the determination of Cu2+ in test solutions.
Effect of temperature on the sensor reaction
The effect of temperature on the performance of the sensor was studied. The absorption spectra were recorded at various temperatures 25 to 70 °C at 474 nm. As the temperature of the Cu2+ sample increased, the difference in absorbance at 474 nm decreased due to thermal quenching, which was related to the increase in ions lattice vibrations98 and decreasing formation of the complex with the membrane. Increasing the temperature to ≥75 °C eliminated the variation in absorbance indicating no complex formation between Cu2+ and BTAHP. The best temperature to obtain highly sensitive and selective results was 25 ± 2.0 °C.
Response time of the optode
The response time of the Cu2+ optode membrane was evaluated by immersing the optode in a 5 × 10−7 M Cu2+ solution, buffering it at pH 8.75, and following the absorbance change at 474 nm with time. As shown in Fig. 3, the absorbance reached 95% of the steady-state signal in ∼3.0 min.
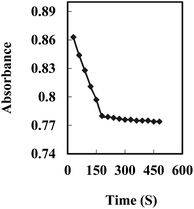 |
| Fig. 3 A typical response curve of the optode at 474 nm as a function of time for Cu2+ concentration of 5 × 10−7 M. | |
Generally, the response time decreased by increasing the analyte concentration and varying it from 2.0 to 3.0 min. This may be explained by the fact that at higher analyte concentrations, the rate of diffusion of the analyte in the membrane phase may be increased.99
Stoichiometric ratio
The binding mode of BTAHP with Cu2+ was confirmed by the absorbance of the Job plot method. To obtain the Job plot, the experiment was performed between BTAHP and different moles ratio of Cu2+ ions; the maximum difference in absorbance was observed at 0.5 mole fraction of the sensor, which indicated 1
:
1 stoichiometric complexation (Fig. 4). These findings, combined with the infrared (IR) spectra of BTAHP and the Cu–BTAHP complex, provided the basis for proposing a structural representation of the complex as depicted in Scheme 2. The Cu ion formed a covalent bond with the oxygen atom of the hydroxyl group and a coordinate bond with the nitrogen atom of the azo group. For the 1
:
1 binding mode, the conditional formation constant (log
K), was evaluated using the Harvey and Manning equation by applying the data obtained from the Job's method; the result was a value of 8.57, whereas the true constant was 8.70. The mechanism of formation of the optode membrane and complex is represented in Scheme 2.
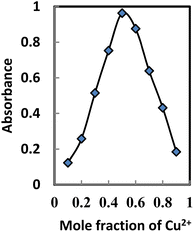 |
| Fig. 4 Job's method for the determination of the Cu2+–BTAHP complex. | |
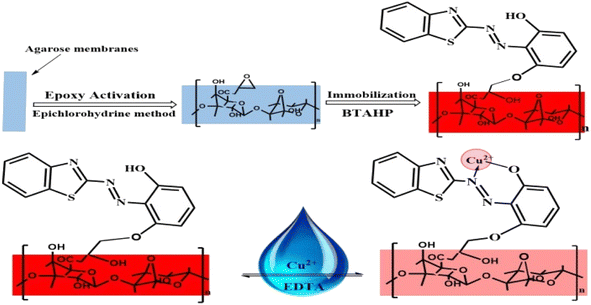 |
| Scheme 2 Representative scheme of the optode and BTAHP–Cu2+ complex formation. | |
Regeneration, reproducibility, short-term stability, and lifetime
Efficient regeneration of the sensor in a reasonable time is a requirement for its usefulness in multiple usages. HCl, NaSCN, and EDTA solutions were tested in different concentrations for the possible regeneration of the membrane after it had been loaded by Cu2+ samples to be examined. The results indicated that a 0.05 M EDTA solution can efficiently remove any adsorbed Cu2+ from the membrane and return its absorbance to the initial value in ∼5.0 min (Fig. 5).
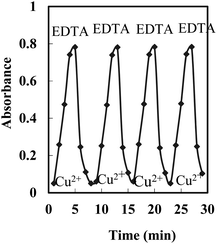 |
| Fig. 5 Repeatability of the optode exposed to 100 ng mL−1 of Cu2+ and 0.05 M EDTA. | |
The reproducibility of the membrane optode response was investigated by its multiple usages for Cu2+ monitoring in test solutions of 5 × 10−7 M. After each absorbance reading, the membrane was regenerated by a 0.05 M EDTA solution, rinsed with some deionized water, and soaked in a phosphate buffer solution (pH 8.75) for a few minutes. Good reproducibility was obtained for the signal at a Cu2+ concentration of 5 × 10−7 M. The corresponding RSD value was found to be ±1.9%.
The results of the intra-day and inter-day precision and accuracy assessments indicated that the approach applied here was highly repeatable and reproducible; the coefficients of variation ranged between 1.65 and 1.90%.
The short-term stability of the proposed sensor was studied by variation in the absorbance measurements, when the sensor was in contact with a 5 × 10−7 M Cu2+ solution at pH 8.75 over a period of 5.0 h. From the absorbance value that was taken every 10 min (n = 30), it was found that the response was almost complete with only a 2.7% increase in absorbance after 5.0 h of monitoring.
The lifetime of the membrane was examined over a period of two months during which four prepared membranes were stored in 20% ethanol at 4 °C. The mean absorbance differences of the sensors at 474 nm were found to be 0.057 (±0.0015) and 0.058 (±0.0019) before and after this period, respectively. Hence, the sensor is stable within this period with a minimum lifetime of two months. In addition, no evidence of dye leaching or signal drift was observed during multiple usages of the membrane.
Effect of interfering ions
For the selectivity investigation, the interference of different inorganic cations on the response of the proposed sensor was tested using solutions of 5 × 10−7 M of Cu2+ and variable concentrations of interfering cations in phosphate buffer (pH 8.75). The tolerance limit was considered to be the concentration that caused an error of more than ±5.0% in the absorbance difference corresponding to a fixed concentration of Cu2+ ions. Based on the data shown in Table 2, it can be seen that BTAHP-based optode is highly selective towards the Cu2+ ion, and it tolerates 400 times or higher concentrations of monovalent, divalent, trivalent, and tetravalent diverse ions. The results confirmed that the optode exhibited excellent selectivity toward Cu2+ ions with respect to the other coexisting interference ions at λmax = 474 nm. Although zirconium formed a complex with BTAHP at a pH of 5.5 on a dextran-type lipophilic gel and was measured at 569 and 800 nm by applying solid-phase spectrophotometry, it does not react under conditions that are optimized for the proposed optode for Cu ion.
Table 2 Effect of the possible interferences on the absorbance of the optode
Interferent |
Concentration (M) |
Absorbance change (ΔA = A2 − A1) |
Relative error (ΔA/A1) × 100 |
K+, Na+ |
2 × 10−4 |
0.015 |
1.92 |
Ag+, Li+ |
2 × 10−4 |
0.023 |
2.94 |
Ca2+, Mg2+ |
2 × 10−4 |
0.18 |
2.30 |
Mn2+, Zn2+ |
2 × 10−4 |
0.027 |
3.95 |
Pb2+, Hg2+ |
2 × 10−4 |
0.031 |
3.96 |
Co2+, Ni2+ |
2 × 10−4 |
0.033 |
4.22 |
Cd2+, Fe2+ |
2 × 10−4 |
0.35 |
4.48 |
Fe3+, Al3+ |
2 × 10−4 |
0.034 |
4.35 |
La3+, Cr3+ |
2 × 10−4 |
0.32 |
4.09 |
Y3+, Sm3+ |
2 × 10−4 |
0.30 |
3.84 |
Zr4+, Se4+ |
2 × 10−4 |
0.39 |
4.84 |
Th4+, UO22+ |
2 × 10−4 |
0.36 |
4.69 |
Calibration curve
The membrane response curve was studied by the stepwise addition of Cu2+ to a series of test solutions, followed by monitoring the differences in absorbance at 474 nm. It was found that the absorbance decreased continuously by increasing the analyte concentration, and the membrane was saturated when the Cu2+ concentration exceeded 7.5 × 10−7 M. The response curve was linear in a logarithmic scale for a Cu2+ concentration range of 7.5 × 10−6 to 1.0 × 10−9 M (Fig. 6). A line was fitted to the experimental points, and the following equation was achieved for the calibration curve with an R2 value of 0.9988: |
ΔA = −0.0206pCu + 0.2184
| (1) |
Here, pCu stands for −log[Cu2+]. The detection and quantification limits were evaluated by ordinary methods from 3σ and 10σ to be 3.0 × 10−10 and 9.8 × 10−10 M, respectively, which is sufficiently low for the detection of Cu2+ in environmental samples.
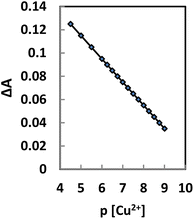 |
| Fig. 6 Logarithmic scale calibration curve for the Cu2+ optode at 474 nm under optimum conditions. | |
The performance of the proposed sensor was assessed by evaluation of the t-value (for accuracy) and F-test (for precision) as compared with the ICP-AES method. The mean values were obtained in a Student's t- and F-tests at 95% confidence limits for five degrees of freedom.100 The results showed that the calculated values did not exceed the theoretical values. The wider range of determination, higher accuracy, increased stability, and lower time consumption shows the advantages of the proposed method over the compared method.
Accuracy and precision
To check the validity, accuracy, and applicability of the proposed method and the reproducibility of the results presented, six replicate experiments at six concentrations of Cu2+ ions were carried out. The relative standard deviations of the various Cu2+ concentrations that were obtained from the experiments were carried out. It was found that the within-day relative standard deviations were <1.7%, indicating that the proposed method was highly reproducible and that BTAHP was successfully applied to determine Cu2+ in the complex formation.
Performance comparison of the Cu2+ ion optode with other works
There are several Cu2+ ion detection methods reported in the literature based on reflectance,62 fluorescence,63,70 transducers, optical absorption,23,64–69,71–74 and the use of different immobilization substrates. A comparison of the sensing performance of the Cu2+ ion optode developed in this work with similar methods from other literature reports is summarized in Table 1 with regard to dynamic linear response range and limit of detection. The developed Cu2+ ion optode was noted to exhibit improved dynamic linear range with shorter response time, i.e., within 3.0 min as compared to other reported optical Cu2+ ion optodes. This was largely due to the large surface area of BTAHP that provided multiple binding sites for immobilization of the chemical sensing reagent, which considerably increased the diffusion rate of the target analyte and the loading capacity of both the reactant and the target. Moreover, the utilization of a BTAHP multi-dentate derivative as the sensing reagent also promoted higher binding capacity due to the availability of multi-banding sites of the BTAHP reagent for Cu2+ ions. The use of agarose as the immobilization matrix, on the other hand, was reported to be beneficial for a wider working linear range in the quantification of Cu2+ ions.44 The proposed optode, which was based on immobilized BTAHP as an ionophore, for Cu2+ ion detection demonstrated no leaching of the sensing materials from the micro-sized immobilization substrate due to the excellent compatibility between the lipophilic BTAHP ligand compound.
Analytical performance
Determination of copper ions in drinking water samples. Copper is an essential trace element required to maintain good health as it plays a vital role in several enzymes. A normal adult requires 2–4 mg of copper per day most of which is provided by food and ∼10% comes from drinking water. However, consumption of excessive levels of copper can cause nausea, vomiting, diarrhea, and headaches. The USEPA (United States Environmental Protection Agency) and European Council Directive proposed the Maximum Contaminant Level for Cu in drinking water to be 1.3 μg mL−1.101 It is necessary to make an accurate, fast, and cheap optode for the determination of Cu(II) in water.Analysis of the spiked copper samples further proved the validity of the proposed procedure. For this reason, the solutions of concentrations between 1.0 and 3.0 μg mL−1 were spiked in the sample by using 50 mL of a water sample. Copper was assessed using the proposed optode after the samples were homogenized, and the proposed methodology was implemented. Analytical parameters derived from standard studies as provided for the proposed method were used to derive the results. Table 3 showed the experimental results of spiked copper samples. The RSDs were below 2.15%. Calculated recoveries for waters were found between 98.33 and 102.67%.
Table 3 Recovery studies of copper in real samples
Sample |
Added ng mL−1 |
Founda ng mL−1 |
RSD (%) |
Recovery (%) |
t-Testb |
F-Valueb |
Sensor |
ICP-AES |
Average of six replicate measurements. Theoretical values of t and F at 95% confidence limit were 2.57 and 5.05, respectively. |
Tap water |
0.0 |
6.6 |
6.3 |
— |
— |
— |
— |
100 |
106.0 |
106.5 |
1.7 |
99.44 |
|
3.84 |
200 |
209.5 |
203.1 |
1.5 |
101.40 |
1.59 |
|
Well water |
0.0 |
77.0 |
75.5 |
— |
— |
— |
— |
70 |
149.0 |
142.3 |
1.2 |
101.36 |
|
3.32 |
140 |
213.2 |
218.7 |
1.4 |
98.25 |
1.42 |
|
River water |
0.0 |
61.5 |
63.0 |
— |
— |
— |
— |
65 |
123.8 |
125.0 |
1.6 |
97.87 |
|
3.57 |
130 |
195.8 |
197.8 |
1.4 |
102.25 |
1.08 |
|
Vegetable |
0.0 |
28.5 |
28.8 |
— |
— |
— |
— |
50 |
79.0 |
76.8 |
1.3 |
100.64 |
|
3.41 |
100 |
127.0 |
131.5 |
1.6 |
98.83 |
1.72 |
|
Lotus (tree) |
0.0 |
18.0 |
17.8 |
|
— |
— |
— |
90 |
107.0 |
110.6 |
1.1 |
99.07 |
|
3.68 |
180 |
200.0 |
194.3 |
1.5 |
99.13 |
1.25 |
|
Liver |
0.0 |
29.5 |
29.0 |
— |
— |
— |
— |
75 |
106.0 |
111.7 |
1.1 |
101.44 |
1.81 |
|
150 |
178.0 |
185.3 |
1.4 |
99.16 |
|
3.52 |
Meat |
0.0 |
31.0 |
30.5 |
— |
— |
— |
— |
60 |
90.4 |
88.8 |
1.7 |
99.34 |
1.66 |
|
120 |
152.5 |
154.7 |
1.5 |
100.99 |
|
3.28 |
Soil |
0.0 |
17.6 |
18.1 |
— |
— |
— |
— |
80 |
99.0 |
95.6 |
1.4 |
101.43 |
1.53 |
|
160 |
178.4 |
180.9 |
1.2 |
100.45 |
|
2.92 |
Determination of copper ions in environmental samples. In order to confirm the applicability of the proposed sensor procedure, it has been used to determine nanogram amounts of Cu2+ in environmental (vegetable biological and soil) samples. The reliability of the presented procedure was checked by spiking experiments and independent analysis. The results are presented in Table 3. An ICP-AES methodology was applied as the reference method, and the results are shown in Table 3. The recovery of spiked samples is satisfactorily reasonable and was confirmed using the standard addition method, which indicates the capability of the system to determine the ions. A good agreement was achieved between the added and measured analyte amounts. The recovery values evaluated for the added standards were always higher than 95%, thus confirming the accuracy of the method and its independence from the matrix effects.
Determination of copper ions in foodstuff samples. The proposed sensor was considered to be an ideal choice for the target analyte determination in a wide variety of real foodstuff samples as they possess numerous advantages, such as simplicity, speed, low cost, possibility of rapid sampling, and selectivity.To assess the applicability of the proposed Cu2+ sensor, an attempt was made to determine the Cu2+ ion concentration in some real foodstuff samples. The samples were treated by the reported procedures as described above and analyzed by the proposed sensor. The analysis was performed by the standard addition technique. The results are presented in Table 4. According to this Table 4, the obtained results are comparable with those obtained by ICP-AES. Thus, the optode is a good alternative method for the determination of Cu2+ in different real samples.
Table 4 Results obtained by the application of the proposed Cu2+ sensor to some foodstuff products
Sample |
Added μg g−1 |
Founda μg g−1 |
Recovery (%) |
t-Testb |
F-Valueb |
Proposed |
ICP-AES |
Average of six replicate measurements ± standard deviation. Theoretical values of t and F at 95% confidence limit were 2.57 and 5.05, respectively. |
Coffee |
— |
1.86 ± 0.31 |
1.91 ± 0.56 |
— |
|
|
2.5 |
4.45 ± 0.38 |
4.20 ± 0.82 |
102.06 |
1.09 |
|
5.0 |
6.75 ± 0.24 |
7.00 ± 0.63 |
98.40 |
|
3.77 |
7.5 |
9.55 ± 0.17 |
9.15 ± 0.90 |
102.27 |
1.37 |
|
Black tea |
— |
4.39 ± 0.32 |
4.47 ± 0.77 |
— |
|
|
5.0 |
9.20 ± 0.42 |
9.70 ± 0.95 |
97.98 |
|
3.57 |
10 |
14.55 ± 0.23 |
14.15 ± 0.86 |
101.11 |
1.43 |
|
15 |
20.00 ± 0.37 |
19.00 ± 0.67 |
103.15 |
|
3.87 |
Black currant |
— |
4.97 ± 0.21 |
5.11 ± 0.84 |
— |
|
|
7.5 |
12.55 ± 0.24 |
12.80 ± 0.97 |
100.64 |
1.47 |
|
15 |
19.70 ± 0.19 |
20.40 ± 0.81 |
98.65 |
|
3.16 |
22.5 |
27.25 ± 0.45 |
27.85 ± 0.78 |
99.20 |
1.56 |
|
Sour cherry juice |
— |
0.61 ± 0.25 |
0.69 ± 1.04 |
— |
|
|
1.5 |
2.15 ± 0.29 |
2.00 ± 0.79 |
101.90 |
|
3.68 |
3.0 |
3.70 ± 0.18 |
3.40 ± 1.03 |
102.49 |
1.32 |
|
4.5 |
5.00 ± 0.39 |
5.35 ± 0.88 |
97.85 |
|
3.17 |
Milk powder |
— |
4.20 ± 0.33 |
4.15 ± 0.69 |
— |
|
|
3.0 |
7.15 ± 0.28 |
7.35 ± 0.85 |
99.31 |
1.17 |
|
6.0 |
10.45 ± 0.41 |
9.90 ± 1.01 |
102.45 |
|
3.68 |
9.0 |
13.00 ± 0.34 |
13.45 ± 1.07 |
98.48 |
1.46 |
|
The performance of the optode was assessed by calculation of the t-value (for accuracy) and F-test (for precision) as compared with the ICP-AES method. The mean values were obtained in a Student's t- and F-tests at 95% confidence limits for five degrees of freedom.100 The results showed that the calculated values did not exceed the theoretical values indicating no significant difference with the compared procedure.
The data (Table 5) reveal a reasonable consistency within the assessed values through the proposed technique for six edible oil samples and that conventionally depend on ICP-AES for Cu quantitation. Accuracy was assessed by comparing results with those from the ICP-AES. Applying the paired t-test and F-value shows no significant differences at a 95% confidence level.
Table 5 Results obtained for Cu(II) determination in six edible oil samples
Sample |
Spiked ng L−1 |
Founda (ng L−1) ± SD |
Recovery, % |
t-Testb |
F-Valueb |
Proposed |
FAAS |
Average of six replicate measurements ± standard deviation. Theoretical values of t and F at 95% confidence limit are 2.57 and 5.05, respectively. |
Extra virgin olive oil |
— |
75.0 ± 0.44 |
75.5 ± 1.21 |
— |
— |
|
250 |
281.5 ± 0.27 |
267.5 ± 1.43 |
102.36 |
1.65 |
|
500 |
564.5 ± 0.18 |
588.0 ± 1.73 |
98.17 |
|
3.28 |
750 |
842.0 ± 0.29 |
811.5 ± 1.50 |
102.60 |
1.23 |
|
Soybean oil |
— |
76.0 ± 0.16 |
75.35 ± 1.27 |
— |
|
|
300 |
368.5 ± 0.21 |
392.0 ± 1.62 |
98.01 |
1.72 |
|
600 |
694.0 ± 0.26 |
662.0 ± 1.38 |
102.66 |
|
3.18 |
900 |
1000 ± 0.19 |
954.5 ± 1.15 |
102.46 |
1.34 |
|
Chilli oil |
— |
109.0 ± 0.13 |
108.50 ± 1.52 |
— |
|
|
200 |
303.5 ± 0.31 |
315.0 ± 1.37 |
98.22 |
1.17 |
|
400 |
515.5 ± 0.25 |
500.0 ± 1.53 |
102.38 |
|
3.44 |
600 |
720.0 ± 0.21 |
695.5 ± 1.31 |
101.55 |
1.86 |
|
Palm oil |
— |
99.0 ± 0.15 |
100.50 ± 0.88 |
— |
|
|
350 |
458.5 ± 0.27 |
466.5 ± 0.96 |
102.12 |
|
2.84 |
700 |
792.0 ± 0.33 |
715.0 ± 1.56 |
99.12 |
1.55 |
|
1050 |
1135.5 ± 0.37 |
1125.0 ± 1.44 |
98.78 |
|
3.63 |
Rice bran oil |
— |
91.0 ± 0.18 |
91.5 ± 1.76 |
— |
|
|
400 |
482.5 ± 0.31 |
500.5 ± 1.21 |
98.27 |
1.79 |
|
800 |
909.0 ± 0.34 |
873.5 ± 1.17 |
102.02 |
|
3.52 |
1200 |
1307 ± 0.22 |
1266.0 ± 0.95 |
101.24 |
1.48 |
|
Corn oil |
— |
77.5 ± 0.29 |
77.0 ± 1.32 |
— |
|
|
500 |
565.0 ± 0.12 |
593.5 ± 1.04 |
97.84 |
|
3.83 |
1000 |
1100 ± 0.51 |
1105.0 ± 1.72 |
102.09 |
1.81 |
|
1500 |
1559.5 ± 0.38 |
1600.0 ± 1.47 |
98.86 |
|
3.69 |
Conclusion
The BTAHP-based Cu2+ optode membrane developed in this study exhibits excellent Cu2+ selectivity as compared to other common metal ions. For direct evaluation of the analyte in samples of polluted natural water, the optode exhibits great sensitivity and low detection and quantification limits. Simple membrane preparation and the development of a covalent bond between BTAHP and the agarose membrane help form an optode with a long lifetime and a rapid reaction time that does not show any signs of dye leaching. The optode membrane may be easily regenerated by an EDTA solution; it also offers the possibility of multiple usages for environmental monitoring of Cu2+. Due to the advantages of the proposed optode method with respect to previously reported ones (Table 1), it may be used as a promising alternative for copper determination in water, food, and environmental samples.
Data availability
All data and materials will be made available upon request.
Author contributions
Mai Aish: conceptualization, data curation, investigation, methodology, validation, visualization, writing – original draft, writing – review & editing. Validation, writing – original draft, writing–review & editing. Reem Alshehri: conceptualization, investigation, methodology, validation, writing – original draft, writing – review & editing. Alaa Amin: conceptualization, methodology, data curation, investigation, supervision, writing–original draft, writing – review & editing.
Conflicts of interest
The authors declare that they have no known competing financial interests or personal relationships that could have appeared to influence the work reported.
Acknowledgements
The authors offer their sincere thanks to Port Said, Taibah, and Benha Universities for providing the necessary facilities.
References
- G. Forstner and T. Wittmann, Metal pollution in the aquatic environment, Springer Verlag, Newyork, 1981, vol. 3, pp. 271–318 Search PubMed
. - Q. Meng, R. Zhang, H. Jia, X. Gao, C. Wang and Y. Shi, et al., Talanta, 2015, 143, 294–301 CrossRef CAS PubMed
. - F. Bulcke, R. Dringen and I. F. Scheiber, Neurotoxicity of Copper. Neurotoxicity of Metals. Springer, 2017, pp. 313–343 Search PubMed
. - I. F. Scheiber, J. F. Mercer and R. Dringen, Prog. Neurobiol., 2014, 116, 33–57 CrossRef CAS PubMed
. - B. E. Kim, T. Nevitt and D. J. Thiele, Nat. Chem. Biol., 2008, 4, 176–185 CrossRef CAS PubMed
. - J. Prohaska and A. A. Gybina, J. Nutr., 2004, 134, 1003–1006 CrossRef CAS PubMed
. - E. Que, D. W. Domaille and C. J. Chang, Chem. Rev., 2008, 108, 1517–1549 CrossRef CAS PubMed
. - A. V. Davis and T. V. O'Halloran, Nat. Chem. Biol., 2008, 4, 148–151 CrossRef CAS PubMed
. - N. J. Robinson and D. R. Winge, Annu. Rev. Biochem., 2010, 79, 537–562 CrossRef CAS PubMed
. - E. Gaggelli, H. Kozlowski, D. Valensin and G. Valensin, Chem. Rev., 2006, 106, 1995–2044 CrossRef CAS PubMed
. - I. Bertini and A. Rosato, Cell. Mol. Life Sci., 2008, 65, 89–91 CrossRef CAS PubMed
. - S. G. Kaler, Nat. Rev. Neurol., 2011, 7, 15–29 CrossRef CAS PubMed
. - A. Ahuja, K. Dev, R. S. Tanwar, K. K. Selwal and P. K. Tyagi, J. Trace Elem. Med. Biol., 2015, 29, 11–23 CrossRef CAS PubMed
. - P. Dusek, P. M. Roos, T. Litwin, S. A. Schneider, T. P. Flaten and J. Aaseth, J. Trace Elem. Med. Biol., 2015, 31, 193–203 CrossRef CAS PubMed
. - Y. H. Hung, A. I. Bush and R. A. Cherny, J. Biol. Inorg. Chem., 2010, 15, 61–76 CrossRef CAS PubMed
. - H. Kozlowski, M. Luczkowski, M. Remelli and D. Valensin, J. Biol. Inorg. Chem., 2012, 256, 2129–2141 CAS
. - R. Squitti, I. Simonelli, E. Cassetta, D. Lupoi, M. Rongioletti, M. Ventriglia and M. Siotto, Curr. Alzheimer Res., 2017, 14, 1318–1326 CrossRef CAS PubMed
. - D. Strausak, J. F. Mercer, H. H. Dieter, W. Stremmel and G. Multhaup, Brain Res. Bull., 2001, 55, 175–185 CrossRef CAS PubMed
. - G. Multhaup, A. Schlicksupp, L. Hesse, D. Beher, T. Ruppert, C. L. Masters and K. Beyreuther, Science, 1996, 271, 1406–1409 CrossRef CAS PubMed
. - I. Yreula, Braz. J. Plant Physiol., 2005, 17, 145–156 CrossRef
. - M. Kumar and A. Puri, A review of permissible limits of drinking water, Indian J. Occup. Health, 2012, 16, 40–44 Search PubMed
. - J. J. Pinto, C. Moreno and M. Garcia-Vargas, Talanta, 2004, 64, 562–565 CrossRef CAS PubMed
. - E. Pourbasheer, S. Morsalia, A. Banaei, S. Aghabalazadeh, M. R. Ganjali and P. Norouzi, J. Ind. Eng. Chem., 2015, 26, 370–374 CrossRef CAS
. - C. Boonmee, T. Noipa, T. Tuntulani and W. Ngeontae, Spectrochim. Acta, Part A, 2016, 169, 161–168 CrossRef CAS PubMed
. - M. Camino, M. G. Bagur and M. Sa, J. Anal. At. Spectrom., 2001, 16, 638–642 RSC
. - R. J. Cassella, O. I. B. Magalh, M. Tadeu, E. Luiz, S. Lima, M. Ang, F. S. Neves, F. Margarida and B. Coutinho, Talanta, 2005, 67, 121–128 CrossRef CAS PubMed
. - B. F. Reis, M. Knochen, G. Pignalosa, N. Cabrera and J. Giglio, Talanta, 2004, 64, 1220–1225 CrossRef CAS PubMed
. - M. H. Mashhadizadeh, M. Pesteh, M. Talakesh, I. Sheikhshoaie, M. M. Ardakani and M. A. Karimi, Spectrochim. Acta, Part B, 2008, 63, 885–888 CrossRef
. - K. Pomazal, C. Prohaska, I. Steffan, G. Reich and F. K. Josef, Analyst, 1999, 124, 657–663 RSC
. - L. C. Ferreira, A. S. Queiroz, M. S. Fernandes and H. C. Santos, Spectrochim. Acta, Part B, 2002, 57, 1939–1950 CrossRef
. - A. Asfaw and G. Wibetoe, Microchim. Acta, 2005, 68, 61–68 CrossRef
. - A. A. Vaughan and R. Narayanaswamy, Sens. Actuators, B, 1998, 51, 368–376 CrossRef CAS
. - J. S. Becker, A. Matusch, C. Depboylu, J. Dobrowolska and M. V. Zoriy, Anal. Chem., 2007, 79, 6047–6080 CrossRef PubMed
. - D. Walaszek, M. Senn, A. Wichser, M. Faller, B. Wagner, E. Bulska and A. Ulrich, Spectrochim. Acta, Part B, 2014, 99, 115–120 CrossRef CAS
. - B. Yin, Z. Liu, M. Yi and J. Zhang, Tetrahedron Lett., 2008, 49, 3687–3690 CrossRef CAS
. - A. P. S. Gonzales, M. A. Firmino, C. S. Nomura, F. R. P. Rocha, P. V. Oliveira and I. Gaubeur, Anal. Chim. Acta, 2009, 636, 198–204 CrossRef CAS PubMed
. - A. Mohadesi and M. A. Taher, Talanta, 2007, 72, 95–100 CrossRef CAS PubMed
. - A. A. Ensafi, T. Khayamian, A. Benvidi and E. Mirmomtaz, Anal. Chim. Acta, 2006, 561, 225–232 CrossRef CAS
. - C. Topcu, G. Lacin, V. Yilmaz, F. Coldur, B. Caglar and I. Isildak, Anal. Lett., 2018, 51, 1890–1910 CrossRef CAS
. - M. A. Chamjangali, S. Soltanpanah and N. Goudarzi, Sens. Actuators, B, 2009, 138, 251–256 CrossRef CAS
. - X. Zhang, J. Peng, C. He, G. Shen and R. Yu, Anal. Chim. Acta, 2006, 567, 189–195 CrossRef CAS
. - T. Mayr and T. Werner, Analyst, 2002, 127, 248–252 RSC
. - L. L. Tan, A. Musa and Y. H. Lee, Sens. Actuators, B, 2012, 173, 614–619 CrossRef CAS
. - S. S. M. Hassan, S. A. Marei, I. H. Badr and H. A. Arida, Anal. Chim. Acta, 2001, 427, 21–28 CrossRef CAS
. - H. Filik and D. Aksu, Sens. Actuators, B, 2009, 136, 105–112 CrossRef CAS
. - P. L. Yew and Y. H. Lee, Sens. Actuators, B, 2014, 191, 719–726 CrossRef CAS
. - A. Tigrerosa and J. Portilla, RSC Adv., 2020, 10, 19693–19712 RSC
. - P. Grandjean, P. Weihe, R. F. White and F. Debes, Environ. Res., 1998, 77, 165–172 CrossRef CAS PubMed
. - B. High, D. Bruce and M. M. Richter, Anal. Chim. Acta, 2001, 449, 17–22 CrossRef CAS
. - L. Tapia, M. Suazo, C. Hodar, V. Cambiazo and M. Gonzalez, BioMetals, 2003, 16, 169–174 CrossRef CAS PubMed
. - D. J. Waggoner, T. B. Bartnikas and J. D. Gitlin, Neurobiol. Dis., 1999, 6, 221–230 CrossRef CAS PubMed
. - H. Jiang, D. Tang, Z. Li, J. Li, H. Liu, Q. Meng, Q. Han and X. Liu, Spectrochim. Acta, Part A, 2020, 243, 118784 CrossRef CAS PubMed
. - H. B. Wang, H. Y. Bai, Y. S. Wang, T. Gan and Y. M. Liu, Microchim. Acta, 2020, 187, 185–189 CrossRef CAS PubMed
. - R. Zare-Dorabei, K. Dashtian and V. Jalalat, IEEE Sens. J., 2015, 15, 6715–6718 CAS
. - R. Zare-Dorabei, P. Norouzi and M. R. Ganjali, J. Hazard. Mater., 2009, 171, 601 CrossRef CAS PubMed
. - K. Alizadeh, B. Rezaei and E. Khazaeli, Sens. Actuators, B, 2014, 193, 267–271 CrossRef CAS
. - H. Xu, J. W. Aylott, R. Kopelman, T. J. Miller and M. A. Philbert, Anal. Chem., 2001, 73, 4124 CrossRef CAS PubMed
. - S. Dong, M. Luo, G. Peng and W. Cheng, Sens. Actuators, B, 2008, 129, 94–98 CrossRef CAS
. - K. Dashtian and R. Zare-Dorabei, Sens. Actuators, B, 2017, 242, 586–590 CrossRef CAS
. - M. Swati, N. Nisha and R. Srivastava, J. Bionanosci., 2007, 1, 90–96 CrossRef
. - R. Güell, C. Fontàs, V. Salvadó and E. Anticó, Anal. Chim. Acta, 2007, 594, 162–167 CrossRef PubMed
. - W. E. F. W. Khalid, M. Ahmad and L. Y. Heng, Chiang Mai J. Sci., 2014, 41, 383–389 CAS
. - N. Aksuner, E. Henden, I. Yilmaz and A. Cukurovali, Sens. Actuators, B, 2008, 134, 510 CrossRef CAS
. - B. Rezaei, H. Hadadzadeh and A. Azimi, J. Anal. Chem., 2012, 67, 687–691 CrossRef CAS
. - M. Ghaedi, A. Shahamiri, S. Hajati and B. Mirtamizdoust, J. Mol. Liq., 2014, 199, 483–488 CrossRef CAS
. - R. Zare-Dorabei, S. M. Ferdowsi, A. Barzinand and A. Tadjarodi, Ultrason. Sonochem., 2016, 32, 265–276 CrossRef CAS PubMed
. - M. Ghaedi, J. Tashkhourian, M. Montazerozohori, M. N. Biyareh and B. Sadeghian, Arabian J. Chem., 2017, 10, S2319 CrossRef CAS
. - T. Leelasattarathkul, S. Liawruangrath, M. Rayanakorn, B. Liawruangrath, W. Oungpipat and N. Youngvises, Talanta, 2007, 72, 126–131 CrossRef CAS PubMed
. - E. Pourbasheer, S. Morsali, Z. Azari, M. A. Karimi and M. R. Ganjali, Appl. Organomet. Chem., 2018, 32, 1–8 CrossRef
. - M. Koneswaran and R. Narayanaswamy, Sens. Actuators, B, 2009, 139, 104–108 CrossRef CAS
. - N. Alizadeh, A. Moemeni and M. Shamsipur, Anal. Chim. Acta, 2002, 464, 187–191 CrossRef CAS
. - I. M. Steinberg, A. Lobnik and O. S. Wolfbeis, Sens. Actuators, B, 2003, 90, 230–235 CrossRef CAS
. - W. M. Khairul, M. F. Abu Hassan, A. I. Daud, H. M. Zuki, K. H. K. Bulat and M. A. Kadir, Malaysian J. Anal. Sci., 2016, 20, 73–84 CrossRef
. - M. A. Chamjangali, S. Soltanpanah and N. Goudarzi, Sens. Actuators, B, 2009, 138, 251–256 CrossRef CAS
. - H. Cheng and Y. Qian, Dyes Pigm., 2015, 112, 317–326 CrossRef CAS
. - M. Dodangeh, K. Gharanjig and M. Arami, Spectrochim. Acta, Part A, 2016, 154, 207–214 CrossRef CAS PubMed
. - J. Dessingiou, J. K. Khedkar and C. P. Rao, J. Chem. Sci., 2014, 126, 1135–1141 CrossRef CAS
. - H. G. Lee, K. B. Kim, G. J. Park, Y. J. Na, H. Y. Jo, S. A. Lee and C. Kim, Inorg. Chem. Commun., 2014, 39, 61–65 CrossRef CAS
. - K. Ghosh and D. Kar, J. Inclusion Phenom. Macrocyclic Chem., 2013, 77, 67–74 CrossRef CAS
. - L. Tang, P. Zhou, Z. Huang, J. Zhao and M. Cai, Bull. Korean Chem. Soc., 2013, 34, 2905–2908 CrossRef CAS
. - J. Jiang, H. Jiang, X. Tang, L. Yang, W. Dou, W. Liu, R. Fang and W. Liu, Dalton Trans., 2011, 40, 6367–6370 RSC
. - P. N. Basa and A. G. Sykes, J. Org. Chem., 2012, 77, 8428–8434 CrossRef CAS PubMed
. - P. Singh, L. S. Mittal, S. Kumar, G. Bhargava and S. Kumar, J. Fluoresc., 2014, 24, 909–915 CrossRef CAS PubMed
. - N. H. Abdul Razak, L. L. Tan, S. A. Hasbullah and L. Y. Heng, Microchem. J., 2020, 153, 104460 CrossRef CAS
. - L. K. Kumawata, N. Mergua, A. K. Singh and V. K. Gupta, Sens. Actuators, B, 2015, 212, 389–394 CrossRef
. - A. I. Vogel, A Text-Book of Quantitative Inorganic Analysis, 3rd edn, Longmans, London, 1961, p. 441 Search PubMed
. - A. S. Amin, J. Taibah Univ. Sci., 2015, 9, 227–236 CrossRef
. - J. A. Dean, Analytical Chemistry Handbook, McGraw-Hill, New York, 1995, pp. 14.30–14.34 Search PubMed
. - I. Matsumoto, Y. Mizuno and N. Seno, J. Biochem., 1979, 85, 1091–1096 CrossRef CAS PubMed
. - P. Hashemi and M. M. Abolghasemi, Sens. Actuators, B, 2006, 115, 49–53 CrossRef CAS
. - A. K. Singh, S. Mehtaband and A. K. Jain, Anal. Chim. Acta, 2006, 575, 25–31 CrossRef CAS PubMed
. - M. Ghaedi, A. Shokrollahi, A. H. Kianfar, A. S. Mirsadeghi, A. Pourfarokhi and M. Soylak, J. Hazard. Mater., 2008, 154, 128–134 CrossRef CAS PubMed
. - M. Ghaedi, A. Shokrollahi, M. R. Fathi, S. Gharaghani and M. Soylak, Quim. Nova, 2008, 31, 70–74 CrossRef
. - A. R. Rod, S. Borhani and F. Shemirani, Eur. Food Res. Technol., 2006, 223, 1438–2377 CrossRef
. - P. Hashemi, R. Afzari, M. M. Abolghasemi and A. Olin, Sens. Actuators, B, 2007, 121, 396–400 CrossRef CAS
. - P. Hashemi, M. M. Abolghasemi, K. Alizadeh and R. A. Zarjani, Sens. Actuators, B, 2008, 129, 332–338 CrossRef CAS
. - P. Hashemia, M. Hosseini, K. Zargoosh and K. Alizadeh, Sens. Actuators, B, 2011, 153, 24–28 CrossRef
. - K. Saidi, W. Chaabani and M. Dammak, RSC Adv., 2021, 11, 30926–30936 RSC
. - E. Antico, M. Lerchi, B. Rusterholz, N. Achermann, M. Badertscher, M. Valiente and E. Pretsch, Anal. Chim. Acta, 1999, 388, 327–338 CrossRef CAS
. - J. N. Miller and J. C. Miller, Statistics and Chemometrics for Analytical Chemistry, Prentice-Hall, London, 5th edn, 2005 Search PubMed
. - A. A. Maria and W. I. Neil, Open J. Chem., 2019, 5, 30–34 CrossRef
.
|
This journal is © The Royal Society of Chemistry 2023 |