DOI:
10.1039/D3RA04088G
(Paper)
RSC Adv., 2023,
13, 21781-21792
Controlled synthesis and computational analysis of gold nanostars for the treatment of Fusarium oxysporum†
Received
17th June 2023
, Accepted 6th July 2023
First published on 19th July 2023
1 Introduction
Fusarium oxysporum is responsible for the infectious wilt diseases that affect plants. Fusarium wilt is a soil-borne pathogen that produces mycotoxins that attack sweet potato, tomato, and eggplant products.1,2 The impact of wilt diseases on food crops introduces economic burdens and devastating crop losses to agricultural production. Some chemical pesticides3 are commonly applied to manage wilt diseases.2,4,5 However, the excessive use of chemical pesticides against wilt diseases possibly gives rise to residual toxicity, fungal resistance, environmental pollution, and health hazards. To avoid this predicament, the application of safe biocontrol agents holds promise for treating wilt diseases as compared to toxic pesticides.4,6 Nanomaterials such as silver, gold, and copper nanoparticles (NPs) offer an ideal treatment against F. oxysporum. Nevertheless, the toxicity of the nanomaterials has been a concern in designing antifungal nano agents.7 In this study, gold nanostar synthesized from greener quercetin derivative (QPABA – (4,4′,4′′-((2-(3,4-bis((4-carboxyphenyl)amino)phenyl)-4-oxo-4H-chromene-3,5,7-triyl)tris(azanediyl))tribenzoic acid)) is applied as an antifungal owing to its morphology, small surface-to-volume ratio, and biocompatibility with low toxicity.
Nanomaterials are attractive materials due to their promising application in various fields, such as optics, electronics, catalysis, and biomedicine.7–9 Physical and chemical methods are widely used in the production of nanomaterials.10–12 The development of greener reducing and capping agents for synthesizing gold NPs is the focus of modern nano synthesis.13,14 Gold nanoparticles' unique and tunable properties are promising tools for diverse biomedical applications such as drug treatment, gene therapy, and diagnostic methods.15 To date, enormous efforts have been dedicated to controlling the size and shape of gold nanomaterials using the greener methodology. In this study, para-aminobenzoic acid was applied as a bioactive molecule to tune the shape-directing properties of gold nanoparticles10,13,15
The focus on gold nanostructures is attributed to their unique features such as biocompatibility, inertness, and low toxicity as compared to zinc oxide nanoparticles with high toxicity.16 In general, nanoparticles possess a high surface area-to-volume ratio.17 It is worth stating that the branching/spike morphology of the gold nanostar contributes to the high surface area-to-volume ratio17 necessary for synthesizing an effective nano-fungicide. Furthermore, the branching/spike in gold nanostar possesses high-index facets that exhibit superior catalytic activity and can enhance the signals associated with surface-enhanced Raman spectroscopy (SERS).18
Natural amino acids such as para-aminobenzoic acid play a role as reducing and capping agents for the synthesis of anisotropic gold nanoparticles with biological applications.19,20 However, the biosynthesis of nanomaterials is highly favorable due to the use of phytochemicals such as flavonoids.21 Flavonoids are polyphenols with rich antioxidant properties. The antioxidant property of flavonoids is responsible for reducing metal ions during the synthesis of nanoparticles. Notably, Quercetin (Flavonoid) is partially insoluble in polar solvents with limited aqueous applications.22 Quercetin is functionalized with para-aminobenzoic acid to improve its polar solubility. The resultant product, QPABA, is utilized as a capping and reducing agent for synthesizing gold nanostars.
Herein, we demonstrate the aqueous synthesis of gold nanostars using QPABA at room temperature without additional surfactants. The influence of QPABA on the size, morphology, and monodispersed gold nanostars was initiated by the 2
:
1 ratio of QPABA and gold metal precursor, respectively. Furthermore, spherical nanoparticles were obtained by the 1
:
1 ratio of QPABA and Au3+ ions. The growth process of gold nanostars was monitored using UV-vis spectroscopy and characterized with TEM, XRD, and EDX. The AuNSs showed excellent antifungal activity against F. oxysporum using the agar well-diffusion assay. The stability of the QPABA-Au3+ complex is proposed via DFT calculations for the interactions between Au3+ ions and possible binding sites within the QPABA ligand. The stability of the gold nanostars and their antifungal potency against F. oxysporum shows that the synthesized AuNSs could be adapted as a greener regimen for the treatment of agricultural fungal infections. The cost-effective synthesis and use of QPABA derived from the facile functionalization of quercetin promotes the sustainability of nanomaterial production.
2 Experimental section
2.1 Reagents
Anhydrous quercetin, hydrogen tetrachloroaurate salt (HAuCl4·3H2O), and para-aminobenzoic acid were purchased from Sigma-Aldrich, Milwaukee, WI. Methanol, sodium hydroxide pellets, and hydrochloric acid were purchased from Fisher Scientific, Pittsburg, PA. Ultrapure water with a resistance of >18.2 MΩ cm was utilized for all the synthesis. Fusarium oxysporum was purchased from the American Type Culture Collection (ATCC 48112) (Manassas, VA). 100 mm Corning agar plates were purchased from Fisher Scientific, Pittsburg, PA. Potato dextrose broth was purchased from Oxoid Microbiology Products (Hampshire, UK).
2.2 Experimental characterizations performed on gold nanostars
The synthesized gold nanoparticles were characterized using an Agilent Cary 3500 Multicell UV-vis spectrophotometer. Transmission electron microscopy (TEM), and Selected Area Electron Diffraction (SAED) (JOEL 7100F) were applied for the analysis of the size, shape, and crystallinity of the anisotropic gold nanostars respectively. The TEM samples were prepared by drop-casting the gold nanoparticles on a carbon-coated copper grid and dried overnight. The XRD characterization was performed using Bruker D8 Advance 800234-X-ray operating at 40 kV within the scan range of 20–80°. The effects of anisotropic gold nanoparticles against Fusarium oxysporum were visualized using a Field Emission-Scanning Electron Microscope (FE-SEM) at an accelerating voltage of 10 kV. The EDX equipped with the TEM instrument was utilized for the determination of the atomic compositions of the anisotropic gold nanoparticles.
2.3 Synthesis of quercetin para-aminobenzoic acid (QPABA)
To enhance the polar solubility of quercetin, para-aminobenzoic acid (PABA) was used to functionalize quercetin via a two-step reductive amination. Briefly, 1 mmol of quercetin (QCR) (300.23 mg) was dissolved in 200 mL of methanol in a 500 mL reaction flask. A separate solution of 5 mmol (137.14 mg) para-aminobenzoic acid was made using a 70% acetic acid and 30% water mixture.19 The resultant PABA mixture was added to the QCR mixture. 2 mL of HCl was added to facilitate the reaction and the excess acid was further neutralized using NaOH pellets. After 2 hours, 300 mg of dimethylamine borane was added, and the reaction then proceeded for 24 hours. The resultant QPABA product in solution was purified and thoroughly characterized using analytical techniques. Detailed characterization results have been reported in our previous work.19
2.4 Synthesis of AuNSs using QPABA at room temperature
To achieve the aqueous reduction of Au3+ to Au0, QPABA was utilized as a bifunctional reducing and stabilizing agent. A standard 1 mM aureate solution (HauCl4·3H2O) was prepared in a cleaned 50 mL vial. 5 mM of QPABA was also prepared in another 50 mL vial. In a one-pot chamber, 200 μl aliquots of gold and 200 μl of QPABA solution were reacted with the addition of 1000 μl of distilled water at room temperature (25 °C). Table 1 shows the series of reaction setups for the synthesis of AuNSs. The spectrophotometric measurements of the samples were performed using Agilent Cary 3500 Multicell UV-Vis Spectrophotometer. AuNS were purified via centrifugation at 3500 rpm for 10 minutes and dried for further characterization.19 Scheme 2 demonstrates the synthesis of anisotropic gold nanoparticles.
Table 1 Concentration-dependent synthesis of AuNS at 25 °C
Vials |
QPABA |
Au3+ ions |
Distilled water |
A |
200 |
200 |
1000 |
B |
400 |
200 |
1000 |
2.5 Computational analysis of AuNSs-derived QPABA
Computational studies were performed to determine the optimized geometry for QPABA. Before geometry optimization, a conformational analysis was performed on QPABA using VConf23 and Gaussian 09 (ref. 24) software packages to obtain the most stable conformer. Furthermore, geometry optimization was performed using the DFT B3LYP method, 6-31+G(d) basis set, PCM solvation model, and water was selected as solvent. In this calculation, the GAMESS25 software package was used. QPABA has five hydroxyls (–OH) groups that are binding sites in the structure. Since nanoparticles contain many metal atoms and are impossible to mimic in silico, only one Au atom was selected to determine its interaction with QPABA. Five nanocomposite structures were constructed by replacing the hydrogen atom of each hydroxyl group in QPABA at a time. After obtaining the structures, energy minimizations were performed on the structures with the use of the DFT B3LYP method, LANL2DZ basis set for Au, 6-31G(d,p) basis set for elements other than gold, IEFPCM solvation model was used. In these calculations, the Gaussian 09 (ref. 24) software package was used. GaussView20 and Discovery Studio Visualizer21 software packages were used to visualize the results. Furthermore, the angles around the Au3+ subsequently were preserved, and the interaction energy (stability) between Au3+ and QPABA was determined.
2.6 Preparation of cells
F. oxysporum spores (ATCC 48112) were cultured in the Peptone Dextrose Broth (PDB), and incubated at 28 °C overnight for 48 h. The cells were harvested by centrifugation and adjusted to 105 cells per mL. The antifungal activity of AuNSs was investigated in a dose-dependent manner. The concentration gradients of AuNSs (75, 100, 125 μg mL−1) were transferred to the agar plates seeded with 105 cells per mL fusarium conidia. The plates treated with the AuNSs were then incubated at 28 °C overnight.
2.7 Agar well diffusion assay
To determine the antifungal potency of AuNSs against F. oxysporum, the agar well-diffusion method was adapted for this study.26 Briefly, Molten PDA was obtained by dissolving 3.9 g of the PDA into 100 mL of ultrapure water in a 200 mL beaker. The PDA agar solution was autoclaved at 121 °C for 30 min. The PDA molten agar was then poured into a 100 mm Petri dish plates (Fisher Scientific), and the agar plates solidified in a biosafety hood. The yeast cells (100 μl mL−1) were seeded onto the agar plates, followed by creating three equidistant 5 mm wells on each agar plate using a Cork borer.26,27 Each agar plate was loaded with AuNSs (75 μg mL−1, 100 μg mL−1, and 125 μg mL−1) in one experimental setup. QPABA in solution was used as the control. The antifungal potency of AuNS nanoparticles against F. oxysporum was determined from the zone of inhibition using the control as a reference. The plates were incubated at 28 ± 2 °C for 7 days. The zone of inhibition was calculated as a percentage28 using the equation below:
Antifungal inhibition (%) = (Mb − Ma)/Mb |
where Ma is the diameter of the inhibition zone around the wells (mm) and Mb is the diameter of the inhibition zone in the control well (mm).
2.8 Determination of minimum inhibitory concentration (MIC)
Micro-broth dilution assay was adapted to determine the minimum inhibitory concentration (MIC) of the AuNSs against the F. oxysporum. MIC was defined at the lowest concentration of the AuNS to inhibit any growth completely. AuNSs treatment against F. oxysporum was dose-dependent (50, 60, 70, 80, 90, 100, 110, and 120 μg mL−1). The lowest concentration of AuNSs against F. oxysporum that did not show any growth in the broth media after 48 h was referred to as the MIC. The turbidity at 595 nm of the yeast was determined by using an Agilent Cary 3500 Multicell UV-vis spectrophotometer.
2.9 SEM characterization of F. Oxysporum
To determine the effect of AuNSs against Fusarium oxysporum, the spores and hyphae cells were treated with 100 μg μl−1 of AuNS except for the control and incubated at 28 °C for 24 h. The yeast cells treated with AuNSs were harvested for SEM analysis following a standard procedure. Briefly, the yeast cells were centrifugated at 5000 rpm for 5 min. The supernatant was discarded, the precipitates were further washed with PBS (3×), and the cells were then fixed with 2.5% glutaraldehyde for 6 h at 4 °C. The glutaraldehyde solution was discarded, and cells were isolated using a different ethanol mixture of 0, 50, 70, 90, 95, and 100%. Finally, the yeast cells were freeze-dried and mounted for the SEM visualization.29
2.10 Statistical analysis
All experiments were performed in triplicates and the statistical results are reported as mean ± standard deviation (SD). The results were subjected to one-way analysis of variance (ANOVA) using origin 8 with a p < 0.05 considered statistically significant.
3 Results and discussion
3.1 Optical characterization of anisotropic gold nanoparticles
The gold nanostars were synthesized at room temperature by reducing gold ions using QPABA without any surfactant. The one-pot technique for synthesizing AuNSs in this study possesses advantages that include eco-friendly, rapid, facile, and controlled morphology and sizes without using non-toxic materials.30 The effect of QPABA on the morphological characteristics of AuNSs was investigated by varying the concentration of QPABA. QPABA acts as a capping agent and reducing agent for synthesizing AuNSs. Aqueous synthesis of star-shaped and spherical gold nanoparticles using QPABA was monitored using Agilent Cary 3500 Multicell UV-vis spectrophotometer. It is worth noting that the formation of spherical gold nanoparticles and gold nanostars were achieved by maintaining a molar ratio of 1
:
1 and 2
:
1 for QPABA and the gold precursor, respectively.19 The solution gradually changed from light brown to deep wine, indicating the formation of AuNSs as seen in Fig. 1 (inset). The color change is attributed to the excitation of the surface plasmon vibrations, indicating nano-stars formation. The reaction was completed in 1 h.
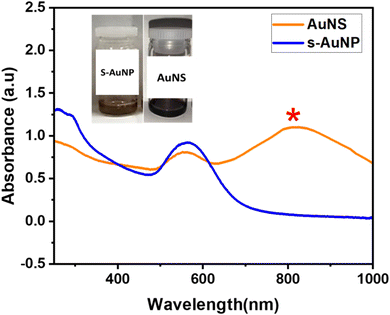 |
| Fig. 1 UV-vis spectra of gold nanostars and spherical gold nanoparticles synthesized with QPABA and gold precursor at a molar ratio of 2 : 1 and 1 : 1, respectively. | |
The characteristic SPR band observed at 540 nm increased with an increase in the concentration of QPABA. This effect eventually led to the formation of star-shaped gold nanoparticles with an SPR peak at 800 nm. The peaks formed by the QPABA-Au3+ observed in the UV-visible spectroscopic analyses confirmed the uniform size and shape of the nanoparticles. The corresponding UV-vis absorption spectrum shows two characteristic bands centered at 550 and 800 nm which corresponds to the localized surface plasmon resonances (LSPR) of the cores and the tip of the gold nanostars, respectively.31 Notably, the size and shape of AuNPs have influences on the surface plasmon resonance (SPR) band, stability, color, and optical absorption intensity.
3.2 Kinetic growth process of anisotropic gold nanostars
To understand the growth process of gold nanostars, time-course measurements of the UV-vis spectroscopy was adapted to monitor the formation of nanoparticles and the evolution of shapes that lead to star formation. The color change from the initial reaction solution was time-dependent.19 The color transitioned from a pale wine solution to a dark wine color. The color transitions corresponded to the formation of gold nanostars. The resultant plasmon resonance peak for the appearance of the nanostars was located at 800 nm. The experimental setup for synthesizing gold nanostar was sampled at 10 min intervals for UV-vis analysis. Given the duration of synthesis, the reaction mixture after 1 h growth duration led to a constant appearance of SPR peak at 800 nm, hence the formation of gold nanostars. The mechanism of AuNSs initially entails the formation of primary particles via the reduction of HAuCl4 by QPABA, acting as the preferential reducing and capping agent. Then, these particles aggregate via surface interaction and then act as the template assisting the anisotropic growth of gold in favorable directions, with a resultant formation of AuNSs. Maintaining a 2
:
1 ratio of QPABA to the gold precursor favors the growth of gold nanostar. The initial reaction entails the rapid formation of spherical gold nanoparticles. The higher concentration of the QPABA drives the formation of the star shape. Controlling the growth mechanism of the gold nanostar was attributed to the reducing power of the QPABA. The particle growth at the (111) index plane of the nuclei further confirmed the gold nanostar. The as-prepared AuNSs anisotropic growth mechanism possessed unique surface morphology that correlates to its plasmon resonance with abundance protrusions and edges for several applications (Fig. 2).
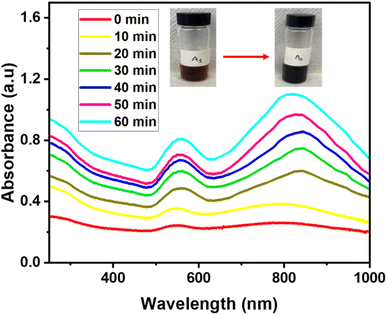 |
| Fig. 2 UV-vis spectra showing the growth process of gold nanostars derived from QPABA and gold precursor in the molar ratio of 2 : 1. The duration of growth occurred over the range of 1 hour. | |
3.3 TEM characterization of anisotropic gold nanoparticles
The morphology of the as-prepared AuNSs was analyzed by the high-resolution TEM in a normal mode. The as-prepared NPs were found to be stable for more than six months without any precipitation. The TEM images showed spherical particles and sizes dependent on the concentration of QPABA.
The average particle size of the spherical nanoparticles is 25.7 ± 2 nm, as seen in Fig. 3E and F. The spherical nanoparticles were derived from a molar ratio of 1
:
1 between QPABA and gold ions. Fig. 3A–C shows images of AuNSs of varying diameters obtained by a 2
:
1 molar ratio of QPABA to gold precursors. As seen in the TEM micrographs, the resultant particles exhibit anisotropy and are uniform with a unique morphology of a peculiar star-like structure.19 TEM images shown in Fig. 3C indicate that nanostars are 40 nm in dimension. Fig. 3A shows that the nanostars consist of a core and obtuse branches originating from the core. The synthesized AuNSs in Fig. 3A–C were found to have average crystallite sizes of 52 ± 3 nm, 40 ± 2 nm, and 58 ± 2 nm, respectively. The morphology of these gold nanostars provides a myriad of applications for the treatment of resistant microbial infections.32 The EDX was used to characterize the elemental mapping of the synthesized gold nanostars.33 The EDX profile of the as-prepared gold nanostars shows that the AuNSs are composed of Au, C, O, and Cu. In Fig. 3D, the EDX confirmed that the gold element was the predominant component of the gold nanostar. The Cu peak signal originated from copper–carbon grid. The source of oxygen and carbon originates from the QPABA ligand. The XRD patterns identified the crystal structure of the as-synthesized gold nanostars as face-centered-cubic.33
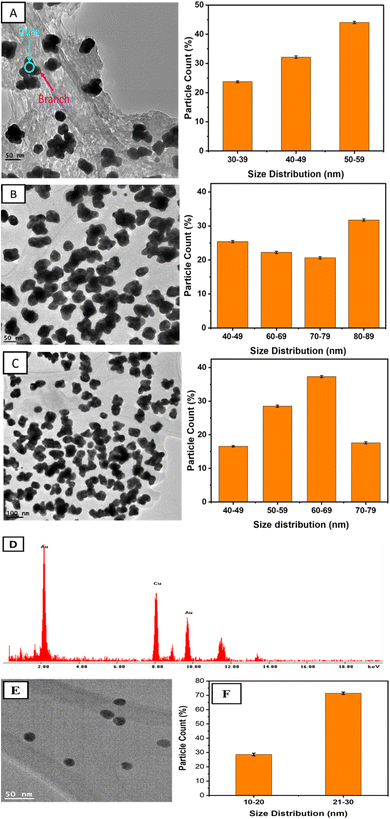 |
| Fig. 3 (A–C) High-resolution TEM images of QPABA-derived gold nanostars and their corresponding histogram of the size distribution. (D) Energy-dispersive X-ray (EDX) elemental spectrum of gold nanostars (E) spherical gold nanoparticles obtained from a 1 : 1 ratio of QPABA and Au3+ ions. (F) Size distribution histogram of spherical gold nanoparticles. | |
As seen in Fig. 4A, four distinct peaks observed at 38.2°, 44.4°, 64.6°, and 78° in the 2θ range of 20–85 °C were ascribed to (1 1 1), (2 0 0), (2 2 0), (3 1 1) indexes, respectively. Notably, the ratio intensity between the (111) and the (200) diffraction is given as 0.32. Since (111) is the highest peak, we can conclude that the preferential growth of gold nanoparticles occurs along the (111) direction.13 The face-centered cubic (fcc) structure of the gold nanostars matches the Joint Committee on Powder Diffraction Standard (JCPDS) card number 00-004-084.13 The Selected Area Electron Diffraction (SAED) pattern of a single gold nanostar in Fig. 4B shows the ring patterns which indicate the crystallinity of the as-prepared AuNSs. The rings of the SAED confirm the crystal structure of the gold nanostars, as confirmed by the XRD.
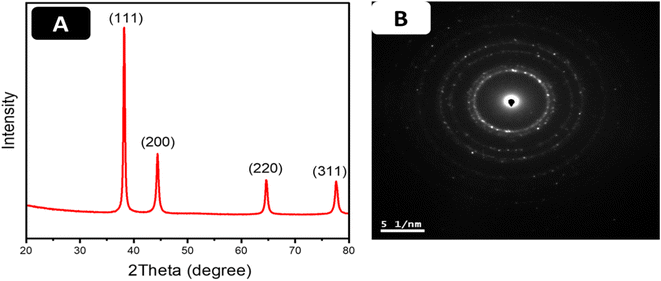 |
| Fig. 4 (A) XRD pattern of AuNS controlled synthesized with 5 mM QPABA. (B) Selected area electron diffraction (SAED) pattern of gold nanostars. | |
3.4 Mechanism of gold nanostars formation using QPABA
Synthetic methods to control the formation of Au nanostars are limited. Nanostars are characterized by an uncertain number of protrusions from their core. In this study, the even formation of monodispersed gold nanostars is achieved by varying concentrations of QPABA. The QPABA plays a shape-determining role in particle morphology owing to its strong reducing and stabilizing capability. The mechanistic growth and formation of gold nanostars are shown in Scheme 3. QPABA reacts with HAuCl4 in a 1
:
1 ratio and forms the Au seeds instantaneously. The reducing capacity of QPABA is slower at low concentrations and the gold seeds are more prominent. The nucleation rate of the Au (0) is rapid when the concentration of QPABA is high; as a result, Au seeds of smaller sizes are synthesized. The TEM results suggest that during the first stage of the reaction, the nuclei growth of gold ions occurs in the presence of the weak binding of low-concentrated QPABA. The 1
:
1 ratio of QPABA and HAuCl4 yields a spherical gold SPR band at 540 nm. Furthermore, the 2
:
1 ratio of QPABA and HAuCl4 leads to an LSPR at 800 nm. The increased amount of QPABA leads to strong interaction and reduction of the gold ions.31 The excess QPABA facilitates the growth of gold seeds from the nucleation phase into star-shaped nanoparticles. Notably, as the protrusions from the core of the nanostar increase in length, the degree of hybridization between the core and branch plasmons increases. A redshift confirms this strong interaction and hybridization at 800 nm. The even formation of the nanostars shows the stabilizing capacity and control of QPABA, orchestrating the formation of the resultant star-shaped particle with myriad applications.
3.5 Comparative analysis of QPABA to different reducing agents
Shape-directing agents for the formation of anisotropic gold nanoparticles and their corresponding applications have been identified in Table 2. Some of these shape-directing agents act as either reducing or capping agents. It has been reported that several factors, such as synthetic method, choice of solvent, and reducing and capping agents play a role in the fabrication of anisotropic nanomaterial.34,35 We highlight some of the reducing agents and the synthetic approach for formulating gold nanostars. Cetyltrimethylammonium bromide (CTAB) is not eco-friendly, and its application in synthesizing anisotropic nanomaterials may render them toxic.36 We seek to promote the greener synthesis of gold nanostars by using plant-based phytochemicals as reducing agents at room temperature.
Table 2 Comparative analysis of shape-directing reducing agent/capping agent for the synthesis of gold nanostars and their applications
Shape-directing reducing agent |
Synthetic method |
Sizes (diameter) |
Application |
References |
Gelatin |
Au-salt (3 mM) reacts with (0.5%) of gelatin at acidic pH (3–6) |
∼60 nm |
Human lung cancer cells (A549) |
Das et al.37 |
Hydroxylamine |
A solution of HAuCl4 solution was added to hydroxylamine was a solution at pH 12 |
42 nm |
Sensing and biomedical application |
Minati et al.38 |
Cetyltrimethylammonium bromide (CTAB) |
Gold seeds stabilized using CTAB solution |
6 nm |
Inhibition of melanoma B16–F10 cells |
Navarro et al.39 |
2-[4-(2-Hydroxyethyl)-1-piperazinyl] propane sulfonic acid (EPPS) |
AuCl4 reacts with EPPS buffer |
6–8 nm |
Detection of uranyl |
Harder et al.40 |
QPABA |
5 mM QPABA reacts with 0.1 mM HAuCl4 |
44 nm |
Inhibition of Fusarium oxysporum |
This study |
QPABA is a quercetin derivative and was synthesized to improve its polar solubility. It was produced via facile two-step reductive amination. Applying QPABA in the anisotropic synthesis of gold nanostars exhibits a greener approach for utilizing safe reducing agents. QPABA is compared to other reducing agents to determine its reducing and stabilizing capacity as natural derivatized quercetin. QPABA has several binding sites for interacting with gold ions. The strength of the interaction determines the ability to both reduce and stabilize the growth of the star-shaped nanoparticles. As shown in Scheme 1, there are several potential sites of binding: carboxyl groups in proximity to the gold ions in solution, there are amide groups in Ring B, that are easily oxidized. The functional groups in Ring A could be farther and unavailable for binding. The orientation and steric hindrance of the functional groups in QPABA could impact their binding to gold ions. Depending on the molecular geometry, the carboxyl groups in proximity may interact directly with the gold atoms leading to low-energy stabilized structures. Such possibilities are needed to explain the unusual stability observed in the resulting AuNSs. Hence computational studies were employed to give a better insight into the interactions.
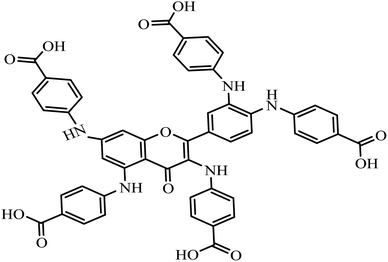 |
| Scheme 1 Structure of quercetin-para-aminobenzoic acid (QPABA). | |
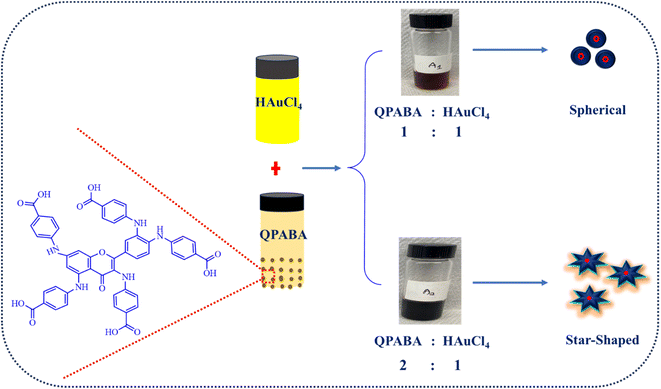 |
| Scheme 2 Synthesis of anisotropic gold nanoparticles using QPABA. | |
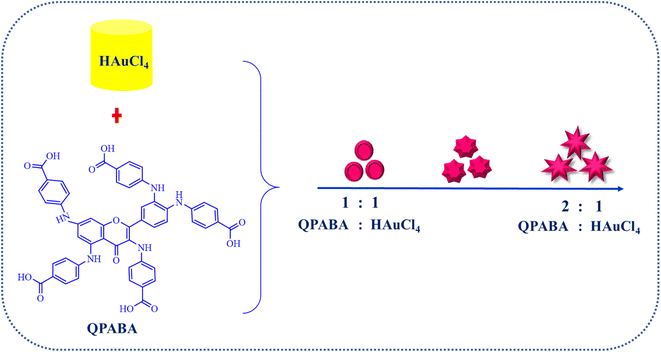 |
| Scheme 3 Mechanistic formation of gold nanostars in increasing QPABA. | |
3.6 Computational analysis of QPABA-derived AuNS
In this study, optimized structures of QPABA ligand and QPABA – Au3+ structures (MS1-5) and corresponding energies were determined. The different conformational optimized structures are shown in Fig. 5. To understand the effects of the energy in the formation of a specific configuration, we performed a geometrical optimization of the QPABA and gold complex to determine their stability. Fig. 6 shows the binding energies for the different calculated models. It is worth stating that the morphology of AuNSs depends on the concentration of the reducing agent used in the reduction process. The stability of the nanoparticles is evaluated using density functional theory based on quantum mechanical calculations.20,41 The minimum energy for each of the structures was obtained utilizing a geometry optimization task based on the DFT method. The geometry optimization was followed by a single-point energy calculation to obtain the energies of the various QPABA-gold conformations. The energies corresponding to possible QPABA–Au nanocomposite structures (MS1-5) were calculated to obtain the most stable structure among all possible structures. The results showed that the energy corresponding to the most stable nanocomposite model structure (MS2) was found to be −1.29222 × 107 kJ mol−1 among all possible structures. To understand the stabilizing role of QPABA in the synthesis of gold nanostars, the three-dimensional structure and binding mode of carboxylate-containing ligand (QPABA) to gold ions have been determined using computational modeling.42
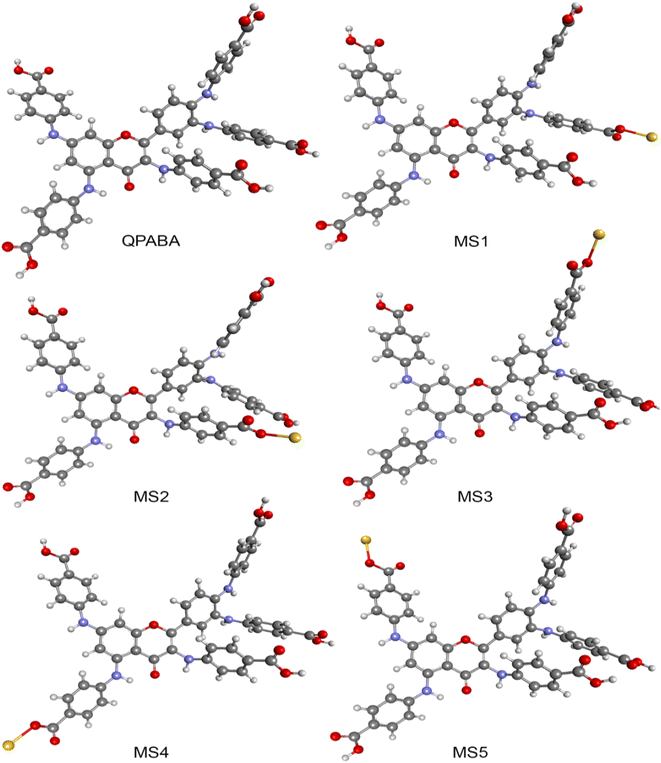 |
| Fig. 5 Optimized structures of QPABA and QPABA–Au nanocomposite model structures (MS1-5). | |
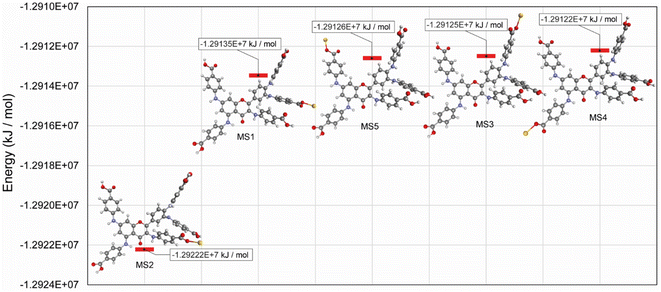 |
| Fig. 6 Possible QPABA–Au3+ nanocomposite model structures and corresponding energies. | |
The binding effect between the QPABA and the gold ions occurs at positions 3 and 4 OH-rich regions of QPABA. The OH site for gold reduction is favored at high QPABA to gold ratios. The interactions between gold and QPABA occur through coordination bonds. Since QPABA has different OH sites for reducing the gold ions, the DFT calculation showed that the stable conformer (MS2) is the favorable configuration of QPABA for reducing gold ions.
The different interaction energy (Eint) between gold ions and the different OH sites on the QPABA is displayed in Fig. 6. In the present work, Eint for the QPABA–gold complex was modeled after a mono-substitution of the hydrogen atoms of each hydroxyl group before interaction with gold ions.20 A comparison of Eint values shows that the interaction energies of the most favorable QPABA–Au complex bonds are −1.29222 × 107 kJ mol−1. The highest stability of the resultant QPABA-Au3+ complex was attributed to the intramolecular bonding between the OH groups and the gold ions. Several reports have identified the poor stability of different metallic nanostars.43,44 Wu et al. reported that the fabrication of anisotropic gold nanoflowers transformed into isotropic spherical gold nanoparticles within 1 h owing to the thermodynamically unstable character of nanoflowers.45 The formation and stability of the nanostars are determined by the reducing and capping agent and the reaction conditions. The QPABA acting as both reducing and capping agents shows a high stability for the fabrication of gold nanostars due to intra-H bonding and using water as a solvent at room temperature.
3.7 Antifungal activity of AuNSs against F. oxysporum
Fusarium wilt caused by F. oxysporum deteriorates the quality of plants. The pathogen can survive for a long time and inflict a significant loss of agricultural produce.46 There is a need for inexpensive management of fusarium wilts outbreaks. The agar well diffusion assay was performed to determine the mycelial growth inhibition activity of the star-shaped gold nanoparticles against F. oxysporum. The effect of AuNS treatment on the mycelial growth of F. oxysporum strains were tested for 7 days of incubation. The growth of F. oxysporum with and without exposure to AuNSs are shown in Fig. 7.
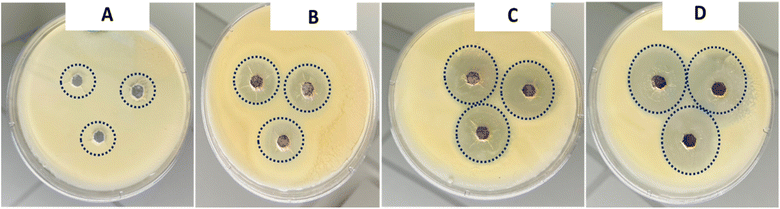 |
| Fig. 7 Antifungal dose-dependent treatment of QPABA-derived F. oxysporum. Control did not receive the treatment of AuNS, but just the reducing/capping agent, QPABA. (A) Control (B) 75 μg mL−1, (C) 100 μg mL−1, and (D) 125 μg mL−1. | |
The cells (106 cells per CFU) were treated with AuNSs in a dose-dependent manner (75 μg mL−1, 100 μg mL−1, and 125 μg mL−1). From our results, it was obvious that AuNSs significantly reduced the mycelial growth after 7 days as compared with the untreated controls. Fig. 7 shows that increasing the concentration treatments of AuNS corresponded to an increase in the zone of inhibition. The zone of inhibition represents the efficacy of the AuNSs. The zones of inhibition were used to estimate the susceptibility of AuNSs to F. oxysporum.28 From the results, treatment of AuNS at 125, 100, and 75 μg mL−1 yielded a reduction in mycelial growth that corresponded to the zone of inhibitions of 15.2 ± 0.63 mm, 12.20 ± 0.45 mm, and 8.20 ± 0.56 mm respectively. It was obvious that higher dose treatment yielded higher zones of inhibition. The zones where F. oxysporum did not grow correlated to the MIC after the minimum treatment of the AuNS. This approach aided the selection of the appropriate concentration of the AuNS treatment for F. oxysporum. The MIC of AuNS against F. oxysporum was 100 μg mL−1, and this represents the lowest concentration for treatment. Nevertheless, treatment using spherical gold nanoparticles following the treatment procedure illustrated above did not show inhibition of the mycelia growth. In this sense, we can conclude that the nanostar shape influences the antifungal activity against F. oxysporum.
The measured diameter of inhibition zones around each diffusion well after treatment of the AuNSs is shown in Table 3. Some factors that contributed to the antifungal activity included concentration, small particle size, and morphology of the nanoparticles. It is worth noting that, at constant dosage treatment and sizes for both spherical and star-shaped gold nanoparticles, the effectiveness of the nanostars was more pronounced compared to the spherical gold nanoparticles.30 In this view, it was concluded that the shape of the resultant particles played a role in the mycelia reduction of F. oxysporum (Table 2).
Table 3 Zone of inhibition (mm) after AuNS treatment against F. oxysporum in a dose-dependent manner
Fungus |
AuNS treatment (μg mL−1) |
Zone of inhibition (mm) |
Day 2 |
Day 5 |
F. oxysporum |
Control |
0.00 ± 0.00 |
0.00 ± 0.00 |
75 |
7.20 ± 0.70 |
8.20 ± 0.56 |
100 |
11.00 ± 1.20 |
12.20 ± 0.45 |
125 |
14.90 ± 0.90 |
15.2 ± 0.63 |
3.8 SEM analysis of F. oxysporum after AuNSs treatment
SEM microscopy was used to assess whether the AuNSs impacted the growth and morphological transformation of the F. oxysporum. From Fig. 8A, the control without treatment showed rapid mycelial growth of F oxysporum after 48 h of incubation at 28 °C. In contrast, the treatment of AuNS (100 μg mL−1) showed morphological changes, as seen in Fig. 8B. Thus, the results strongly suggest that AuNSs can effectively inhibit the growth of F. oxysporum.
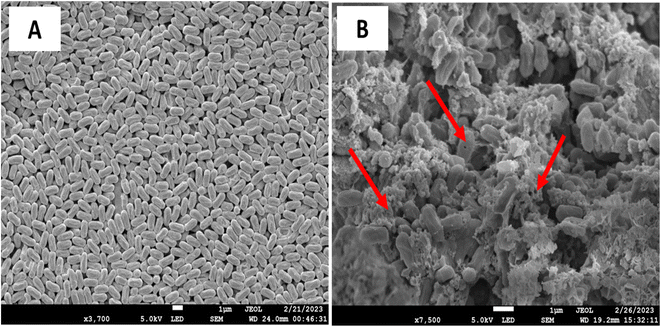 |
| Fig. 8 SEM analysis of before (A) and after treatment of 75 μg mL−1 of AuNS. Arrows show the disintegrated membrane of the F. oxysporum. | |
The mechanism of antifungal activity can be attributed to the morphology and high surface-to-volume ratio of AuNSs with an increased affinity to interact with the fungi, hence the antifungal activity of AuNSs against F. oxysporum is effective. Furthermore, the antifungal mechanism of NPs is ascribed to other factors, such as the absorption and accumulation of ions in the fungal cell, which damages the cell membrane enzymes.47 SEM showed significant morphological alterations in F. oxysporum upon exposure to AuNPs. The morphological change in structure leads to fungal cell death. These data are consistent with reported data that shows that the effects of AuNPs on F. oxysporum disrupt the cell membrane and organelles. Furthermore, another possible mechanism of action for AuNS demonstrated against fusarium has been reported by Xie et al.48,49 The AuNS treatment disintegrated the cells with their sharp edges that eventually killed the fungi. Together, these results suggest that the antifungal mechanism of gold nanostars is influenced by the concentration of gold ions and the size and morphology of particles. Our results are consistent with data reported elsewhere.50,51
4 Conclusion
The shape-controlling capacity of the quercetin derivative QPABA has been demonstrated as a greener and low-cost reducing and capping agent for synthesizing gold nanostars with high yield. The resultant AuNSs were characterized by TEM, UV-vis, XRD, and EDX to confirm the morphology, size, and crystallinity of the particles and shape evolution of gold nanostars. The computational analysis confirmed the structural conformation and stability of the QPABA and gold ions to form gold nanostars. The theoretical analysis proved that the formation of the AuNSs was ascribed to the conformational interaction between gold ions and QPABA at positions 3 and 4 in addition to factors such as the concentration of the ligand and choice of solvent. The antifungal activity of the AuNSs was effective against F. oxysporum at a MIC of 100 μg mL−1. Our study shows the site-specific interaction between gold ions and QPABA for the synthesis of anisotropic gold nanoparticles to treat agricultural-based fungal infections. The future work will focus on the influence of shape on the cytotoxicity of gold nanostars against MCF-7 human breast cancer cell lines. Since cytotoxicity is a measure of biocompatibility,52 it is expected that the morphological properties of the gold nanostars will play a role in determining the cytotoxicity effects against breast cancer cell lines. This will enlighten and contribute to the established biocompatibility of star-shaped gold nanoparticles.
Conflicts of interest
There are no conflicts of interest to declare.
Acknowledgements
The authors acknowledge the US National Science Foundation Grant #IOS-1543944, NJIT Start-ups (172803), and the Bill & Melinda Gates Foundation for funding. The computational studies reported in this paper were partially performed at TUBITAK ULAKBIM, High Performance and Grid Computing Center (TRUBA resources), and Kocaeli University.
References
- H. M. Ji, H. Y. Mao, S. J. Li, T. Feng, Z. Y. Zhang, L. Cheng, S. J. Luo, K. A. Borkovich and S. Q. Ouyang, New Phytol., 2021, 232, 705–718 CrossRef CAS PubMed.
- H. Ashraf, T. Anjum, S. Riaz, I. S. Ahmad, J. Irudayaraj, S. Javed, U. Qaiser and S. Naseem, Environ. Sci.: Nano, 2021, 8, 1729–1748 RSC.
- O. Degani and B. Kalman, J. Fungi, 2021, 7, 235 CrossRef CAS.
- X. Huang, J. Zhao, X. Zhou, Y. Han, J. Zhang and Z. Cai, Eur. J. Soil Sci., 2019, 70, 518–529 CrossRef CAS.
- M. A. Khan, S. A. Khan, U. Waheed, M. Raheel, Z. Khan, A. F. Alrefaei and H. H. Alkhamis, J. King Saud Univ., Sci., 2021, 33, 101299 CrossRef.
- H. Shang, C. Ma, C. Li, J. Zhao, W. Elmer, J. C. White and B. Xing, Environ. Sci. Technol., 2021, 55, 13432–13442 CrossRef CAS PubMed.
- K. Giannousi, A. Pantazaki and C. Dendrinou-Samara, in Nanostructures for Antimicrobial Therapy, Elsevier, 2017, pp. 515–529 Search PubMed.
- R. Yazdian-Robati, N. Hedayati, S. Dehghani, M. Ramezani, M. Alibolandi, M. Saeedi, K. Abnous and S. M. Taghdisi, Anal. Biochem., 2021, 629, 114307 CrossRef CAS PubMed.
- C. T. Campbell, J. C. Sharp, Y. Yao, E. M. Karp and T. L. Silbaugh, Faraday Discuss., 2011, 152, 227–239 RSC.
- F. J. Osonga, G. Eshun, S. Kalra, I. Yazgan, L. Sakhaee, R. Ontman, S. Jiang and O. A. Sadik, ACS Agric. Sci. Technol., 2022, 2, 42–56 CrossRef CAS.
- J. C. L. Chow, in Handbook of Functionalized Nanomaterials, Elsevier, 2021, pp. 281–308 Search PubMed.
- J. A. Moore and J. C. Chow, Nano Express, 2021, 2, 022001 CrossRef.
- F. J. Osonga, V. M. Kariuki, V. M. Wambua, S. Kalra, B. Nweke, R. M. Miller, M. Cesme and O. A. Sadik, Acs Omega, 2019, 4, 6511–6520 CrossRef CAS.
- S. Siddique and J. C. Chow, Nanomaterials, 2020, 10, 1700 CrossRef CAS PubMed.
- N.-f. Sun, Z.-a. Liu, W.-b. Huang, A.-l. Tian and S.-y. Hu, Crit. Rev. Oncol./Hematol., 2014, 89, 352–357 CrossRef PubMed.
- Y. Xiao, Y. Li, Y. Shi, Z. Li, X. Zhang, T. Liu, T. H. Farooq, Y. Pan, X. Chen and W. Yan, Sci. Total Environ., 2022, 806, 151211 CrossRef CAS.
- A. Candreva, F. Parisi, R. Bartucci, R. Guzzi, G. Di Maio, F. Scarpelli, I. Aiello, N. Godbert and M. La Deda, Chemistryselect, 2022, 7, e202201375 CrossRef CAS.
- L. Wei, T. Sheng, J.-Y. Ye, B.-A. Lu, N. Tian, Z.-Y. Zhou, X.-S. Zhao and S.-G. Sun, Langmuir, 2017, 33, 6991–6998 CrossRef CAS PubMed.
- F. J. Osonga, G. B. Eshun and O. A. Sadik, RSC Adv., 2022, 12, 31855–31868 RSC.
- A. Jakhmola, R. Vecchione, F. Gentile, M. Profeta, A. Manikas, E. Battista, M. Celentano, V. Onesto and P. Netti, Mater. Today Chem., 2019, 14, 100203 CrossRef CAS.
- F. J. Osonga, A. Akgul, R. M. Miller, G. B. Eshun, I. Yazgan, A. Akgul and O. A. Sadik, ACS Omega, 2019, 4, 12865–12871 CrossRef CAS PubMed.
- F. J. Osonga, J. O. Onyango, S. K. Mwilu, N. M. Noah, J. Schulte, M. An and O. A. Sadik, Tetrahedron Lett., 2017, 58, 1474–1479 CrossRef CAS.
- C. E. Chang and M. K. Gilson, J. Comput. Chem., 2003, 24, 1987–1998 CrossRef CAS PubMed.
- M. J. Frisch, G. W. Trucks, H. B. Schlegel, G. E. Scuseria, M. A. Robb, J. R. Cheeseman, G. Scalmani, V. Barone, G. A. Petersson, H. Nakatsuji, X. Li, M. Caricato, A. V. Marenich, J. Bloino, B. G. Janesko, R. Gomperts, B. Mennucci, H. P. Hratchian, J. V. Ortiz, A. F. Izmaylov, J. L. Sonnenberg, D. Williams-Young, F. Ding, F. Lipparini, F. Egidi, J. Goings, B. Peng, A. Petrone, T. Henderson, D. Ranasinghe, V. G. Zakrzewski, J. Gao, N. Rega, G. Zheng, W. Liang, M. Hada, M. Ehara, K. Toyota, R. Fukuda, J. Hasegawa, M. Ishida, T. Nakajima, Y. Honda, O. Kitao, H. Nakai, T. Vreven, K. Throssell, J. A. Montgomery Jr, J. E. Peralta, F. Ogliaro, M. J. Bearpark, J. J. Heyd, E. N. Brothers, K. N. Kudin, V. N. Staroverov, T. A. Keith, R. Kobayashi, J. Normand, K. Raghavachari, A. P. Rendell, J. C. Burant, S. S. Iyengar, J. Tomasi, M. Cossi, J. M. Millam, M. Klene, C. Adamo, R. Cammi, J. W. Ochterski, R. L. Martin, K. Morokuma, O. Farkas, J. B. Foresman and D. J. Fox, Gaussian 16, Revision C.01, Gaussian, Inc., Wallingford, CT, 2016 Search PubMed.
- G. M. Barca, C. Bertoni, L. Carrington, D. Datta, N. De Silva, J. E. Deustua, D. G. Fedorov, J. R. Gour, A. O. Gunina and E. Guidez, J. Chem. Phys., 2020, 152, 154102 CrossRef CAS PubMed.
- P. Nidhi, R. Rolta, V. Kumar, K. Dev and A. Sourirajan, J. Ethnopharmacol., 2020, 262, 113135 CrossRef CAS PubMed.
- S. Magaldi, S. Mata-Essayag, C. H. De Capriles, C. Pérez, M. Colella, C. Olaizola and Y. Ontiveros, Int. J. Infect. Dis., 2004, 8, 39–45 CrossRef CAS.
- S. Raj, V. Vinod, J. Jayakumar, P. Suresh, A. Kumar and R. Biswas, Lett. Appl. Microbiol., 2021, 73, 31–38 CrossRef CAS PubMed.
- C. Zhao, X. Lv, J. Fu, C. He, H. Hua and Z. Yan, J. Appl. Microbiol., 2016, 121, 254–262 CrossRef CAS.
- F. J. Osonga, I. Yazgan, V. Kariuki, D. Luther, A. Jimenez, P. Le and O. A. Sadik, RSC Adv., 2016, 6, 2302–2313 RSC.
- A. Kedia and P. S. Kumar, J. Mater. Chem. C, 2013, 1, 4540–4549 RSC.
- M. Borzenkov, G. Chirico, L. d'Alfonso, L. Sironi, M. Collini, E. Cabrini, G. Dacarro, C. Milanese, P. Pallavicini and A. Taglietti, Langmuir, 2015, 31, 8081–8091 CrossRef CAS PubMed.
- R. P. Talemi, S. M. Mousavi and H. Afruzi, Mater. Sci. Eng., C, 2017, 73, 700–708 CrossRef CAS PubMed.
- K. Vijayaraghavan and T. Ashokkumar, J. Environ. Chem. Eng., 2017, 5, 4866–4883 CrossRef CAS.
- M. L. Personick and C. A. Mirkin, J. Am. Chem. Soc., 2013, 135, 18238–18247 CrossRef CAS PubMed.
- J. S. Bozich, S. E. Lohse, M. D. Torelli, C. J. Murphy, R. J. Hamers and R. D. Klaper, Environ. Sci.: Nano, 2014, 1, 260–270 RSC.
- R. P. Das, V. V. Gandhi, B. G. Singh and A. Kunwar, New J. Chem., 2021, 45, 13271–13279 RSC.
- L. Minati, F. Benetti, A. Chiappini and G. Speranza, Colloids Surf., A, 2014, 441, 623–628 CrossRef CAS.
- J. R. Navarro, D. Manchon, F. Lerouge, N. P. Blanchard, S. Marotte, Y. Leverrier, J. Marvel, F. Chaput, G. Micouin and A.-M. Gabudean, Nanotechnology, 2012, 23, 465602 CrossRef PubMed.
- R. A. Harder, L. A. Wijenayaka, H. T. Phan and A. J. Haes, J. Raman Spectrosc., 2021, 52, 497–505 CrossRef CAS PubMed.
- H. Al-Johani, E. Abou-Hamad, A. Jedidi, C. M. Widdifield, J. Viger-Gravel, S. S. Sangaru, D. Gajan, D. H. Anjum, S. Ould-Chikh and M. N. Hedhili, Nat. Chem., 2017, 9, 890–895 CrossRef CAS PubMed.
- G. Zazeri, A. P. R. Povinelli, N. M. Pavan, D. R. de Carvalho, C. L. Cardoso and V. F. Ximenes, J. Mol. Struct., 2021, 1244, 130995 CrossRef CAS.
- W. Xi and A. J. Haes, J. Am. Chem. Soc., 2019, 141, 4034–4042 CrossRef CAS PubMed.
- S. Sasidharan, D. Bahadur and R. Srivastava, ACS Sustainable Chem. Eng., 2017, 5, 10163–10175 CrossRef CAS.
- F. Wu, Y. Liu, Y. Wu, D. Song, J. Qian and B. Zhu, J. Mater. Chem. B, 2020, 8, 2128–2138 RSC.
- W. V. Mbasa, W. A. Nene, F. A. Kapinga, S. A. Lilai and D. D. Tibuhwa, Crop Prot., 2021, 139, 105379 CrossRef CAS.
- L. Hermida-Montero, N. Pariona, A. I. Mtz-Enriquez, G. Carrión, F. Paraguay-Delgado and G. Rosas-Saito, J. Hazard. Mater., 2019, 380, 120850 CrossRef CAS.
- X. Wang, X. Liu, J. Chen, H. Han and Z. Yuan, Carbon, 2014, 68, 798–806 CrossRef CAS.
- J. Xie, Z. Ming, H. Li, H. Yang, B. Yu, R. Wu, X. Liu, Y. Bai and S.-T. Yang, Chemosphere, 2016, 151, 324–331 CrossRef CAS PubMed.
- M. Noman, T. Ahmed, U. Ijaz, M. Shahid, M. M. Nazir, J. C. White, D. Li and F. Song, Small, 2023, 19, 2205687 CrossRef CAS.
- F. J. Osonga, A. Akgul, I. Yazgan, A. Akgul, G. B. Eshun, L. Sakhaee and O. A. Sadik, Molecules, 2020, 25, 2682 CrossRef CAS.
- P. M. Favi, M. Gao, L. Johana Sepúlveda Arango, S. P. Ospina, M. Morales, J. J. Pavon and T. J. Webster, J. Biomed. Mater. Res., Part A, 2015, 103, 3449–3462 CrossRef CAS.
|
This journal is © The Royal Society of Chemistry 2023 |