DOI:
10.1039/D3RA03817C
(Paper)
RSC Adv., 2023,
13, 26196-26202
Lanthanide-MOFs as multi-responsive photoluminescence sensor for sensitively detecting Fe3+, Cr2O72− and nitrofuran antibiotics†
Received
7th June 2023
, Accepted 16th August 2023
First published on 4th September 2023
Abstract
Fast and selective detection of contaminants plays a key role in meeting human health and environmental concerns. Herein, two groups of isostructural lanthanide MOFs, [Ln(Hpta)(oxalic acid)]·H2O (1-Eu, 2-Gd) and [Ln(pta)(oxalic acid)0.5(H2O)2]·2H2O (3-Eu, 4-Gd) (H2pta = 2-(4-pyridyl)-terephthalic acid, C2O4− = oxalic acid), were synthesized by solvothermal method. Single crystal X-ray diffraction reveals that 1 and 2 are 3D neutral frameworks, while 3 and 4 consist of 2D layers with parallelogram holes and stack into 3D networks through O–H⋯N and O–H⋯O hydrogen bonding interactions. All complexes remain crystalline and stable below 400 °C, suggesting preeminent thermostability. Noteworthily, only 3 shows excellent chemical stability in water and organic solvent. Therefore, the solid-state fluorescence spectrum was used to characterize 3 which exhibited intense red luminescence. The N active sites in the pore channels of 3 are conducive to displaying a distinct quenching effect for Fe3+ cations in aqueous solutions, Cr2O72− anions in DMF and DMA solutions, and nitrofuran antibiotics in the DMF solvent. Overall, 3 is a prospective luminescent sensor for detecting Fe3+, Cr2O72− and nitrofuran antibiotics.
Introduction
In recent years, the effective detection of heavy metal ions, inorganic ions and antibiotics has become paramount to regions spanning the globe to protect human health and homeland security.1–3 Now, among the various detection methods, fluorescence-based chemical sensor systems are gaining increasing attention for the detection of nitrofuran antibiotics, inorganic anions and heavy metal ionic substances.4–7
In recent years, luminescent functional metal–organic framework (MOF) materials acting as fluorescent probes have attracted extensive attention.8–10 In comparison to transition metal ions, lanthanide ions possess higher coordination numbers, flexible coordination geometry and narrow emissions, which affect the structural diversity and luminescent properties of Ln-MOFs.11,12 Ln-MOFs impart tunable functionality and designable topology and exhibit unique luminescence properties, including large Stokes shifts, narrow bandwidth, high colour purity and relatively long luminescence lifetimes.13 Furthermore, because the 4f transitions of lanthanide ions are inner-shell transitions and the emission spectra display narrow bandwidths (Δλ < 10 nm), they have been extensively explored for applications in lighting, displays, sensing, and optical devices. These intrinsic luminescence features of lanthanides together with the unique advantages of MOFs offer hopeful prospects for designing novel luminescent materials.14 However, the majority of Ln-MOFs are still unstable in water and organic solvents, as observed with other MOFs, which is mainly attributed to the weak coordinated bond in the coordinated structure.15,16 Accordingly, it is of great significance to design and prepare ultrastable Ln-MOFs for highly selective and sensitive luminescence sensing for antibiotics and heavy metal ion substances in solutions.
Organic ligands, usually acting as building blocks in the assembly of MOFs, help to determine the backbones as well as the functions of these materials. What is noteworthy is that most MOFs in luminescence sensing are synthesized using aromatic carboxylate or pyridyl–carboxylate linkers.17 Pyridyl ligands containing a nitrogen donor are incorporated in MOFs, beneficially increasing the interactive water stability of MOFs.18 Obviously, the versatile pyridyl-decorated dicarboxylic 2-(4-pyridyl)-terephthalic acid (H2pta) will be a promising candidate to afford multifunctional MOFs due to the following points: (1) H2pta contains a pyridyl ring and a phenyl ring with structural flexibility and conformational freedom. Rotation of the C–C single bond between the pyridyl and phenyl rings could form a number of coordination geometries of metal ions. (2) It has two carboxyl groups that may be completely or partially deprotonated, inducing rich coordination modes and allowing interesting structures with higher dimensionalities. (3) The potential uncoordinated pyridyl N atoms as functional sites could recognize Lewis acidic metal ions. These characteristics of the H2pta ligand may lead to cavities, interpenetration, helical structures, and other novel motifs with unique topologies.19–21 Besides, the advantages of the mixed ligand strategy inspire us to introduce other versatile bridging linkers into the reaction system, to act in structural modulation, bridging pillar, or charge balance roles,22 whose particular behaviors allow them to be potential components for designing frameworks with diverse networks and functionalities.
Based on the above considerations, difunctional 2-(4-pyridyl)-terephthalic acid (H2pta) is employed as an organic linker with lanthanide ions to construct Ln(III)-MOFs with magnificent architectures and fascinating functions. Two groups of new isostructural Ln(III)-MOFs, [Ln(Hpta)(C2O4)]·H2O (1-Eu, 2-Gd) and [Ln(pta)(C2O4)0.5(H2O)2]·2H2O (3-Eu, 4-Gd), were obtained with the help of the oxalic acid coligand via hydrothermal reaction (Scheme 1). The crystallographic structures, thermostabilities, and chemical stabilities of these MOFs were characterized. Interestingly, complex 3 shows a highly selective luminescence quenching action for Fe3+ cations in aqueous media, Cr2O72− anions in DMF and DMA solutions and nitrofuran antibiotics in DMF solvent. The good reproducibility and remarkable selectivity of 3 makes it a promising candidate for chemical sensor.
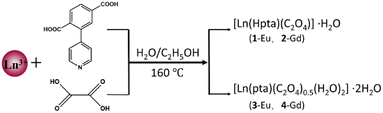 |
| Scheme 1 Synthetic route of complexes 1–4. | |
Experimental
Materials and physical measurements
All commercially available chemicals were used without further purification. Elemental analysis (C, H, and N) was performed using a PerkinElmer 2400 CHN elemental analyzer. An EQUINOX55 FT/IR spectrometer was used to record Fourier-transform infrared spectroscopy (FT-IR) spectra in the range of 400–4000 cm−1 using KBr pellets.23 The phase purity of the bulk or polycrystalline samples was examined by powder X-ray diffraction (PXRD) measurements performed on a Rigaku RU20 diffractometer at 60 kV and 300 mA using Cu Kα radiation (λ = 1.5406 Å) with a scanning speed of 5° min−1 and a step size of 0.02° in 2θ. Thermal gravimetric analysis (TGA) was performed using a NETZSCH STA 449F3 instrument at a heating rate of 10 °C min−1 from 25 to 900 °C under a N2 stream using an empty Al2O3 crucible as the standard. The fluorescence spectra were obtained using a Hitachi F-7000 fluorescence spectrophotometer at room temperature. UV-Vis spectroscopic studies were collected on a Shimadzu WFH-203B spectrophotometer.
Synthesis procedures of complexes
Complexes 1 and 2 were synthesized by the following methods. A mixture of H2pta (0.025 mmol), Ln (NO3)3·6H2O (Ln = Eu, Gd) (0.05 mmol) and oxalic acid (0.05 mmol) was dissolved in 8 mL of CH3CH2OH/H2O (1
:
1) in a 15 mL vial, heated in a Teflon-lined stainless-steel vessel at 160 °C for 3 days and then cooled at a rate of 3 °C h−1 to room temperature. Colorless needle-shaped crystals were collected by filtration and dried in air. The procedures for complexes 3 and 4 were the same as those for 1 and 2 except that the CH3CH2OH/H2O (1
:
1) was replaced by 1
:
7. Colorless block crystals were obtained and collected by filtration.
[Eu(Hpta)(C2O4)]·H2O (1). Yield of 55.0% (based on Eu3+). Anal. (%) calcd for C15H10NO9Eu: C, 36.02; H, 2.02; N, 2.80. Found: C, 35.98; H, 1.96; N, 2.75. IR (KBr pellet, cm−1): 3410(s), 2976(w), 1636(m), 1580(s), 1534(s), 1508(s), 1421(s), 1374(s), 1342(s), 1094(w), 840(m), 808(m), 570(m).
[Gd(Hpta)(C2O4)]·H2O (2). Yield of 45.0% (based on Gd3+). Anal. (%) calcd for C15H10NO9Gd: C, 35.64; H, 1.99; N, 2.77. Found: C, 34.88; H, 1.76; N, 2.45. IR (KBr pellet, cm−1): 3426(s), 2976(m), 1630(m), 1580(m), 1536(m), 1510(m), 1420(m), 1380(m), 1318(m), 1094(w), 804(m), 720(m), 578(m).
[Eu(pta)(C2O4)0.5(H2O)2]·2H2O (3). Yield of 49.0% (based on Eu3+). Anal. (%) calcd for C14H15NO10Eu: C, 33.02; H, 2.97; N, 2.75. Found: C, 32.89; H, 2.83; N, 2.56. IR (KBr pellet, cm−1): 3340(s), 1670(m), 1552(s), 1430(s), 1381(m), 1322(m), 860(m), 779(m), 597(w).
[Gd(pta)(C2O4)0.5(H2O)2]·2H2O (4). Yield of 53.0% (based on Gd3+). Anal. (%) calcd for C14H15NO10Gd: C, 32.68; H, 2.94; N, 2.72. Found: C, 32.48; H, 2.78; N, 2.65. IR (KBr pellet, cm−1): 3342(s), 1667(m), 1550(s), 1429(s), 1379(m), 1320(m), 857(m), 777(m), 599(w).
Crystallographic data collection and refinement
Suitable single crystals of all complexes were selected for X-ray measurements. Crystal structures were collected with a Bruker D8 VENTURE X-ray CMOS diffractometer using graphite monochromated Cu-Kα radiation (λ = 154
178 Å) at 150 K for 1 and 2 and Mo-Kα X-ray radiation (λ = 0.71073 Å) at 100 K for 3 and 4. The structures were determined by direct methods and refined against F2 by full-matrix least squares with Olex 2.3. All non-hydrogen atoms were refined with anisotropic thermal parameters. All hydrogen atoms were placed in calculated positions and refined isotropically. For both 1 and 2, the diffraction contribution of the highly disordered solvent molecules located in the structure was eliminated by applying the program SQUEEZE implemented in PLATON. Crystallographic data and structural refinement details for 1–4 are summarized in Table S1.† Selected bond lengths and bond angles are listed in Table S2.† Crystallographic data from the structural analysis have been deposited with the Cambridge Crystallographic Data Centre. The CCDC numbers are 2180904 (1), 2180910 (2), 2180911 (3) and 2180912 (4).
Results and discussion
Crystal structures of 1–4
Since the crystallographic analysis reveals that complexes 1 and 2 and complexes 3 and 4 are isomorphous, we chose complexes 1 and 3 as representatives to describe the detailed structures. Complex 1 crystallizes in the monoclinic P21/c space group and exhibits a 3D skeleton. As shown in Fig. S1a,† the asymmetric unit is composed of one independent Eu3+ ion, one Hpta− ligand, one coordinated C2O42− and free H2O molecules. The Eu3+ ion is eight-coordinated by four oxygen atoms (O5, O6A, O7A and O8) from C2O42− and four carboxylate oxygen atoms (O1B, O2, O3A and O4A) from three different pta2− ligands, indicating a distorted trigonal prismatic geometry (Fig. 1a). Two carboxylic groups in the Hpta− ligand link with three Eu3+ centers in bridging and chelating modes, respectively (Fig. 1b). Herein, two neighboring Eu3+ ions are connected by two μ2-COO− groups to produce a binuclear secondary building unit (SBU) (Fig. 1c). The SBUs are joined by Hpta− ligands to form an infinite one-dimensional (1D) chain as displayed in Fig. 1d. The C2O42− ligand links the adjacent chains to obtain a two-dimensional (2D) layer which is further enlarged by the C2O42− ligands to engender a 3D porous framework (Fig. 2a and b). The structure of complex 2 is shown in Fig. S1b, S2 and S3.† Complex 3 possesses a 2D framework in the triclinic space group P
. The asymmetric unit of 3 consists of one Eu3+ ion, one pta2− ligand with the protonation of oxygen atom, a half coordinated C2O42− ligand, two coordinated H2O molecules and free H2O molecules (Fig. 3a). As shown in Fig. S1c,† the nine-coordinated Eu3+ ions are surrounded by nine O atoms to generate a muffin geometry, in which five O atoms (O1, O1A, O2A, O3B, O4B) stem from three carboxyl groups of three different pta2− ligands, two O atoms (O5, O6) come from C2O42− ligands and two oxygen atoms (O7W, O8W) come from coordinated water molecules. Two carboxylate groups of each pta2− ligand link with three Eu3+ centers as bridging/chelating tridentate and chelating bidentate (Fig. 3b). Two Eu3+ centers are connected by a pair of μ3-O atoms to form an edge-sharing Eu2(COO)2 unit and the adjacent Eu2(COO)2 units are linked by the pta2− backbones, leading to a 1D double chain (Fig. 3c). The coordination bonds between the Eu3+ ions and pta2− ligands cause the generation of 2D layers (Fig. 3d). Each layer is stacked through the hydrogen bonds of coordinated water molecules (Fig. 4a) and the stacking of layers forms a 3D supramolecular structure (Fig. 4b). The structure of complex 4 is shown in Fig. S1d, S4 and S5.†
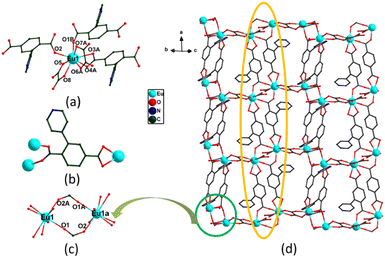 |
| Fig. 1 (a) The coordination environment of Eu3+ in 1; (b) the coordination modes of pta2− in 1; (c) the dinuclear [Eu2(COO)2] cluster; (d) a view of the 2D structure of 1 (Eu cyan, O red, N blue, C grey, hydrogen atoms omitted for clarity). | |
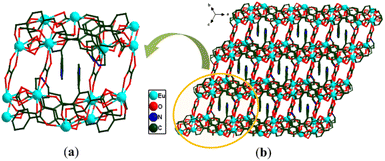 |
| Fig. 2 (a) Layer structure and (b) 3D structure of 1. | |
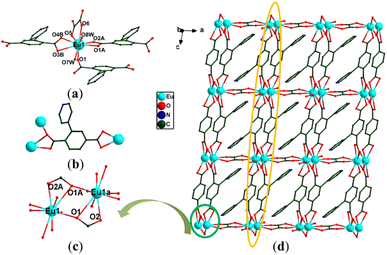 |
| Fig. 3 (a) The coordination environment of Eu3+ in 3; (b) the coordination modes of pta2− in 3; (c) the dinuclear [Eu2(COO)2] cluster; (d) a view of the 2D structure of 3 (Eu cyan, O red, N blue, C grey, hydrogen atoms omitted for clarity). | |
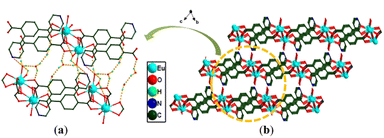 |
| Fig. 4 (a) Layer structure and (b) 3D structure of 3. | |
Powder X-ray diffraction (PXRD) and thermogravimetry (TG)
To confirm the phase purity of the obtained products, powder X-ray diffraction (PXRD) experiments were carried out for complexes 1–4. The measured PXRD results match the simulated patterns based on the single crystal data, suggesting the high phase purity and crystallinity of 1–4 (Fig. S6†).
The TG curves (Fig. S7†) of 1–4 were obtained by thermogravimetric tests under N2 atmosphere. On account of the isomorphism of 1 and 2, as well as 3 and 4, the TG curves of 1 and 3 are described as respective representatives. For 1, the weight loss of 3.32% at 25–97 °C (theoretical value is 3.60%) is due to the loss of free water molecules. The weight loss of 17.55% from 97 to 470 °C is consistent with the removal of the oxalic acids (theoretical value is 17.99%). Above 474 °C, the framework of complex 1 collapses. In the case of 3, the weight loss of 7.99% below 124 °C relates to the removal of free water molecules (calcd 7.07%). The weight loss of 3.62% in the temperature range of 181–218 °C is attributed to the disappearance of coordinated water molecules (calcd 3.53%). Above 418 °C, the main framework of complex 3 decomposes rapidly.
Chemical stability
Further exploring the chemical stability of 1–4 is significant to practical applications. Unfortunately, the chemical stabilities of complexes 1, 2 and 4 were unqualified. In contrast, the peak positions of the PXRD patterns for 3 remain basically unchanged after immersion in water for seven days (Fig. 5a), in both acidic and basic solutions (pH = 4–11) (Fig. 5b), and even in common organic solvents (Fig. 5c) for 24 h at room temperature, indicating that 3 could remain stable in water, acidic/basic solutions and organic solvents.24
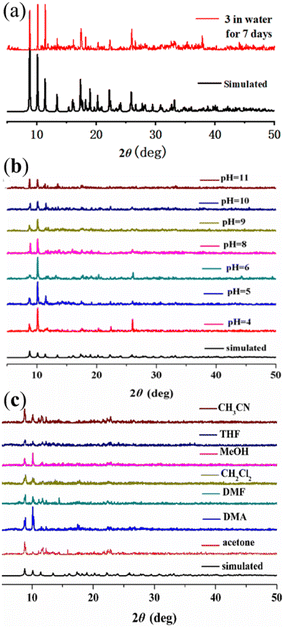 |
| Fig. 5 PXRD profiles of 3 after being soaked in (a) water for seven days, (b) acidic and basic solutions for 24 h, and (c) different organic solvents for 24 h. | |
Fluorescence properties
In this case, the luminescent properties of H2pta and 3 were also investigated. As shown in Fig. S8 and S9,† the luminescence spectrum of 3 reveals characteristic photoluminescence properties with fluorescence peaks at 550, 598, and 623 nm which are attributed to the 5D0 → 7F1, 5D0 → 7F2, and 5D0 → 7F4 transitions of Eu3+ ions, respectively (excitation wavelength 315 nm). From the Eu3+ transition rules, if Eu3+ ions take up an inversion center, orange light is emitted from the magnetic dipole transitions (5D0 → 7F1) and if Eu3+ ions take up a noninversion center, red light is emitted because of the electric dipole transitions (5D0 → 7F2). However, the luminescence intensity of 3 at 623 nm (5D0 → 7F2 transitions) is stronger than that at 598 nm (5D0 → 7F1 transitions), which indicates that Eu3+ ions take up a non-inversion center. This transition is comparable to earlier reported luminescence studies of Eu-based MOFs.25
Detection of metal ions
Based on the excellent luminescence properties and structural stability of complex 3, subsequent fluorescence sensing experiments were performed. The powder samples were immersed in 0.01 mol L−1 of various M(NO3)x (M = K+, Na+, Ni2+, Co2+, Pb2+, Zn2+, Cu2+, Cd2+, Ag+, Ca2+ and Fe3+) aqueous, DMF, DMA, EtOH, MeOH, and CH3CN solutions. The results are shown in Fig. 6a and b. Only Fe3+ ions show a distinct quenching effect on the luminescence of complex 3 in aqueous solutions. In order to confirm the selectivity, more experiments were performed. When Fe3+ and other metal ions (Zn2+, Pb2+, Ni2+, Co2+, Na+, Cu2+, Cd2+) with mixed ratios of 1
:
3 were added to the solution, the fluorescence was completely quenched as well. The results further demonstrate the excellent anti-interference ability of 3 in the detection of Fe3+ (Fig. S10†). Furthermore, a series of titration experiments was carried out in aqueous solutions with various concentrations of Fe3+. The fluorescence intensity of 3 (Fig. 6c) is almost entirely quenched when the Fe3+ concentration is 500 mM. Quantitatively, the quenching efficiency is calculated using the Stern–Volmer (S–V) equation: (I0/I) = 1 + Ksv[Q], where I0 and I are the luminescence intensities before and after adding metal ions, respectively, [Q] refers to the molar concentration of metal ions and Ksv is the quenching constant, which is an important indicator of the sensing ability of a fluorescent sensor. The Ksv value is found to be 1.96 × 104 M−1 for 3 (Fig. 6d), signifying that Fe3+ has a high-efficiency quenching effect on the luminescence emission of 3. According to the ratio of 3δ/Ksv (δ: standard error), the detection limit is 3.25 × 10−5 M for 3. In fact, complex 3 possesses selectivity for both Fe3+ and Cu2+ in organic solvents. The titration experiments and Ksv results are shown in Fig. S11–S14.† Furthermore, 3 (Fig. 7a) was washed with water several times and the fluorescence intensities were well-retained over five runs. Meanwhile, the PXRD patterns prove that the crystallinity and structural integrity of 3 (Fig. 8a) exhibit no change after the detection tests, supporting its good recyclability. Overall, the high sensitivity and recyclability for Fe3+, together with its stability in acidic/basic aqueous solutions, make 3 competitive compared to other MOF fluorescent probes.
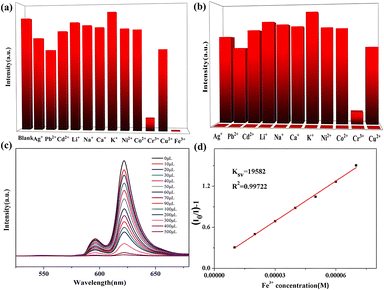 |
| Fig. 6 (a) Luminescence intensity (315 nm excitation wavelength) of 3 treated with 0.01 M of various different metal ions in water; (b) luminescence intensity at 315 nm of 3 dispersed in water with the addition of different ions (0.01 M) (Cu2+, Ag+, Zn2+, Na+, Pb2+, Co2+, K+, Cd2+, Ca2+, Ni2+, Li+) and Fe3+ incorporated systems (0.01 M); (c) luminescence spectra of 3 in aqueous solutions with Fe3+ at different concentrations; (d) a plot of relative luminescence intensity vs. Fe3+ concentration. | |
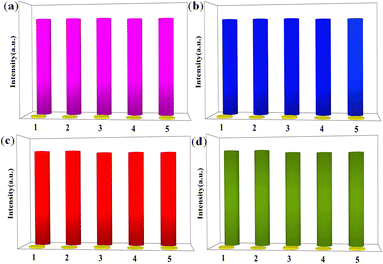 |
| Fig. 7 (a) Recyclability of the quenching ability of 3 immersed in water and in the presence of 0.01 M aqueous solution of Fe3+ ions; (b) recyclability of the quenching ability of 3 immersed in DMF and in the presence of 0.01 M DMF solution of NFT; (c) recyclability of the quenching ability of 3 immersed in DMF and in the presence of 0.01 M DMF solution of Cr2O72− anions; (d) recyclability of the quenching ability of 3 immersed in DMA and in the presence of 0.01 M DMA solution of Cr2O72− anions (the purple/blue/red/green columns represent the initial fluorescence intensity and the yellow columns represent the intensity upon the addition of an aqueous solution of 0.01 M Fe3+/NFT/Cr2O72−). | |
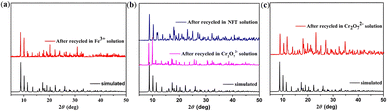 |
| Fig. 8 (a) PXRD patterns of 3 treated by Fe3+ aqueous solution; (b) PXRD patterns of 3 treated by NFT/Cr2O72− in DMF solution; (c) PXRD patterns of 3 treated by Cr2O72− in DMA solution. | |
Detection of antibiotics
To explore the ability of 3 to sense trace quantities of antibiotics, fluorescence-quenching titrations were performed with the addition of antibiotics to DMF in which 3 was dispersed. A total of eight frequently used antibiotics, nitrofurantoin (NFT), nitrofurazone (NFZ), sulfachloropyridazine (SCP), sulfamethoxazole (SMZ), trimethoprim (TMP), penicillin sodium (PCL), and morpholine guanidine hydrochloride (MH), were added. As shown in Fig. 9a, NFT and NFZ, both nitrofuran antibiotics, have the highest quenching efficiency for 3. The quenching efficiencies for 3 of these antibiotics follow the order NFT > NFZ > SCP > SMZ > TMP > MH > PCL. Thus, 3 is a promising luminescent probe for the selective detection of nitrofuran antibiotics. Subsequently, the fluorescence sensing performance of 3 towards NFT inspired us to check the anti-interference ability for NFT detection. Fluorescence titration experiments were performed (Fig. 9b). From the linear fitting of the S–V plot, the calculated Ksv value for NFT is 4.01 × 104 M−1 (Fig. 9c). According to 3δ/Ksv, the calculated detection limit for NFT is 2.69 × 10−5 M. To evaluate the recyclability of 3, cyclic luminescence tests were performed and the fluorescence intensity of 3 was observed to have little variation after the sample was washed with fresh DMF several times (Fig. 7b and 8b). The results demonstrate that 3 is an ideal fluorescence sensor for detecting nitrofuran antibiotics with high sensitivity, good recyclability and superior anti-interference capability.
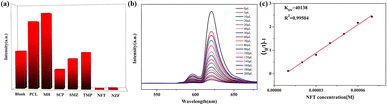 |
| Fig. 9 (a) Luminescence intensity (315 nm excitation wavelength) of 3 treated with 0.01 M of various different antibiotics in DMF; (b) luminescence spectrum of 3 in DMF with NFT at different concentrations; (c) a plot of relative luminescence intensity vs. NFT concentration. | |
Detection of anions
In addition, 3 was also used for the detection of anions in industrial wastewater. It was dispersed into DMF, DMA, EtOH, MeOH, and CH3CN solutions containing potassium salts of NO3−, PO43−, SO42−, Br−, S2O82−, CrO42−, HPO42− and Cr2O72− at the same concentration to study their effects on the luminescent intensity of this MOF. The luminescent measurements clearly illustrate that Fe3+, Cu2+, and Cr2O72− could cause fluorescence quenching for 3 in different organic solvents (Table S3†). Remarkably, Cr2O72− gives rise to the highest quenching effect on the emission of complex 3 in DMA and DMF solutions (Fig. 10a and S15a†). Meanwhile, the selectivity was tested and the sensing results in organic solvents are shown in Fig. 10b and S15b.†
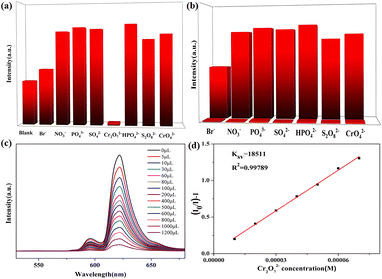 |
| Fig. 10 (a) Luminescence intensity (315 nm excitation wavelength) of 3 treated with 0.01 M of various different anions in DMA; (b) luminescence intensity (315 nm) of 3 dispersed in DMA with the addition of different anions (0.01 M) (NO3−, CrO42−, Br−, SO42−, S2O82−, PO43−, HPO42−) and Cr2O72− incorporated systems (0.01 M); (c) luminescence spectrum of 3 in DMA solutions with Cr2O72− at different concentrations; (d) a plot of relative luminescence intensity vs. Cr2O72− concentration. | |
The fluorescence emission was still remarkably quenched with the addition of Cr2O72−, clearly confirming that the other anions could not interfere with the Cr2O72− sensing. In addition, a series of titration experiments were carried out in DMA and DMF solutions with various concentrations of Cr2O72−. The fluorescence intensities for 3 (Fig. 10c and S15c†) are almost entirely quenched at Cr2O72− ion concentrations of 1200 μL in DMA solution and 700 μL in DMF solution. The Ksv values are found to be 1.85 × 104 M−1 and 8.47 × 103 M−1 for 3 (Fig. 10d and S15d†), showing that Cr2O72− has a high-efficiency quenching effect on the luminescence emission of 3, and the detection limits calculated by 3δ/Ksv are 1.3 × 10−5 M and 6.67 × 10−5 M. Recycling experiments were also recorded for 3 which was washed with acetone solution several times (Fig. 7c and d). The PXRD (Fig. 8b and c) and luminescence intensity curves of the recycled 3 are well consistent with the original results, proving that 3 as a Cr2O72− probe can be recycled with a fast and simple method. In addition, complex 3 possesses selectivity for S2O82− and Cr2O72− in aqueous solvents (Fig. S16†).
Mechanism for sensing of Fe3+, NFT, and Cr2O72−
The mechanism of the fluorescence quenching of 3 caused by metal ions, anions and nitro antibiotics is explained by analyzing the ultraviolet absorption spectra (UV-Vis) of the target products and complex 3.26 The PXRD patterns for 3 before and after the fluorescence experiments are consistent with each other, excluding the possibility of the luminescence quenching effect being derived from the collapse of the framework (Fig. 8). The experimental data indicate that strong absorption bands appear in the UV-Vis absorption spectrum (200–250 nm) of Fe3+ in aqueous solution, while the excitation wavelength of 3 is 315 nm (Fig. S17a†), suggesting that the fluorescence quenching is not caused by competition absorption of the excitation wavelength energy between Fe3+ and complex 3. According to Fig. S18,† the fluorescence lifetime of 3 reduced from 0.568 ns to 0.401 ns after adding Fe3+, indicated that the fluorescence quenching effect is dynamic quenching.27 The UV-Vis absorption spectra of Cr2O72− in DMF and DMA solutions and NFT in DMF solution show strong absorption bands for Cr2O72− in DMF (250–300 and 300–400 nm), Cr2O72− in DMA (300–315 and 315–450 nm), and NFT (250–305 and 305–450 nm) and the excitation wavelength for 3 is 315 nm (Fig. S17b–d†). From the experimental data, the UV-Vis absorption spectra of Cr2O72− and NFT overlap with the excitation spectrum of 3. These facts imply that Cr2O72− and NFT could absorb the energy of the excitation light from 3, reducing the efficiency of the energy transformation from the ligand to the lanthanide ions, which definitely induces the luminescence quenching effect. In addition, the uncoordinated pyridine groups can be used as functional groups to detect metal ions and small organic molecules,28 so the N active sites in the pore channel of 3 may be beneficial to promote the selectivity and sensitivity for detecting Fe3+ cations, Cr2O72− anions and nitrofuran antibiotics.
Conclusion
In conclusion, four new paired MOFs assembled from 2-(4-pyridyl)-terephthalic acid have been successfully isolated and structurally characterized. Particularly, complex 3, which contains Eu3+ ions nine-coordinated in muffin geometry, shows excellent stability and luminescence properties. This Eu(III) based MOF presents the potential for highly sensitive and selective detection of Fe3+ in water and NZF in DMF solution, as well as Cr2O72− in DMF and DMA solutions. The possible mechanism is fluorescence quenching dependent on competitive absorption between complex 3 and the analytes. The successful assembly of the present case may provide a good guideline to construct new Eu(III)-MOF functional materials.
Conflicts of interest
There are no conflicts of interest to declare.
Acknowledgements
This work was supported by the NSFC (21863009 and 22063008), the Natural Science Foundation of Ningxia Province (2023AAC03227, 2022AAC05002, 2021AAC03136 and 2021BEB04062), the Young Top-notch Talent Cultivation Program of Ningxia Province, the Discipline Project of Ningxia (NXYLXK2017A04), the China Postdoctoral Science Foundation (2022M723148) and the Research Project of Ningxia Medical University (XT2019001).
Notes and references
- G.-Q. Zhang, L.-J. Gao, H.-M. Chai and Y.-X. Ren, ACS Omega, 2021, 6, 6810–6816 CrossRef CAS PubMed.
- Z. Sun, J. Sun, L. Xi, J. Xie, X. Wang, Y. Ma and L. Li, Cryst. Growth Des., 2020, 20, 5225–5234 CrossRef CAS.
-
(a) L. Li, Q. Chen, Z. Niu, X. Zhou, T. Yang and W. Huang, J. Mater. Chem. C, 2016, 4, 1900–1905 RSC;
(b) Y. Shi, L. He, X. Wang, Z. Wu, N. Guo, H. Zhang, W. Wang and J. Cui, J. Rare Earths, 2020, 38, 1231–1236 CrossRef CAS;
(c) M. Liu, H. Li, L. Bai, K. Zheng, Z. Zhao, Z. Chen, S. W. Ng, L. Ding and C. Zeng, J. Hazard. Mater., 2021, 413, 125291 CrossRef CAS PubMed.
- P. Jia, Z. Wang, Y. Zhang, D. Zhang, W. Gao, Y. Su, Y. Li and C. Yang, Spectrochim. Acta, Part A, 2020, 230, 118084 CrossRef CAS PubMed.
- L. Liu, Y. Wang, R. Lin, Z. Yao, Q. Lin, L. Wang, Z. Zhang and S. Xiang, Dalton Trans., 2018, 47, 16190–16196 RSC.
- Y. Sun, N. Zhang, Q. L. Guan, C. H. Liu, B. Li, K. Y. Zhang, G. H. Li, Y. H. Xing, F. Y. Bai and L. X. Sun, Cryst. Growth Des., 2019, 19, 7217–7229 CrossRef CAS.
- H. Yu, M. Fan, Q. Liu, Z. Su, X. Li, Q. Pan and X. Hu, Inorg. Chem., 2020, 59, 2005–2010 CrossRef CAS PubMed.
- P. Kumar, A. Deep and K.-H. Kim, TrAC, Trends Anal. Chem., 2015, 73, 39–53 CrossRef CAS.
-
(a) S. Liu, M. Liu, M. Guo, Z. Wang, X. Wang, W. Cui and Z. Tian, J. Lumin., 2021, 236, 118102 CrossRef CAS;
(b) Y. Jiang, K. Zheng, Z. Liu, X. Yu, Q. Yang, T. Tang and C. Zeng, Adv. Opt. Mater., 2022, 10, 2102267 CrossRef CAS.
- B. Li, W. Wang, Z. Hong, E. S. M. El-Sayed and D. Yuan, Chem. Commun., 2019, 55, 6926–6929 RSC.
- H. Wang, X. Wang, M. Liang, G. Chen, R.-M. Kong, L. Xia and F. Qu, Anal. Chem., 2020, 92, 3366–3372 CrossRef CAS PubMed.
-
(a) R. Li, W. Wang, E. S. M. El-Sayed, K. Su, P. He and D. Yuan, Sens. Actuators, B, 2021, 330, 129314 CrossRef CAS;
(b) J. Xue, Y. Wang, G. Yang and Y. Wang, J. Rare Earths, 2023 DOI:10.1016/j.jre.2023.02.016.
-
(a) X. Li, J. X. Tang, H. Liu, K. Gao, X. R. Meng, J. Wu and H. W. Hou, Chem.–Asian J., 2019, 14, 3721–3727 CrossRef CAS PubMed;
(b) M.-Q. Yang, C.-P. Zhou, Y. Chen, J.-J. Li, C.-H. Zeng and S. Zhong, Sens. Actuators, B, 2017, 248, 589–596 CrossRef CAS;
(c) K. Zheng, Z.-Q. Liu, Y. Huang, F. Chen, C.-H. Zeng, S. Zhong and S. W. Ng, Sens. Actuators, B, 2018, 257, 705–713 CrossRef CAS.
- C. C. Zhang, X. F. Ma, P. P. Cen, X. Y. Jin, J. H. Yang, Y.-Q. Zhang, J. Ferrando-Soria, E. Pardo and X. Y. Liu, Dalton Trans., 2020, 49, 14123–14132 RSC.
- Y.-Q. Sun, Y. Cheng and X.-B. Yin, Anal. Chem., 2021, 93, 3559–3566 CrossRef CAS PubMed.
- X. Wang, Z. Jiang, C. Yang, S. Zhen, C. Huang and Y. Li, J. Hazard. Mater., 2022, 423, 126978 CrossRef CAS PubMed.
- F. Q. An, C. C. Zhang, L. J. Duan, X. Y. Liu, Z. Wang, X. Y. Jin and W. M. Song, New J. Chem., 2019, 43, 4800–4807 RSC.
- H. H. Yu, M. Y. Fan, Q. Liu, Z. M. Su, X. Li, Q. Q. Pan and X. L. Hu, Inorg. Chem., 2020, 59, 2005–2010 CrossRef CAS PubMed.
- J. Zhang, Y. Huang, D. Yue, Y. Cui, Y. Yang and G. Qian, J. Mater. Chem. B, 2018, 6, 5174–5180 RSC.
- W.-M. Chen, X.-L. Meng, G.-L. Zhuang, Z. Wang, M. Kurmoo, Q.-Q. Zhao, X.-P. Wang, B. Shan, C.-H. Tung and D. Sun, J. Mater. Chem. A, 2017, 5, 13079–13085 RSC.
- C. Zhang, Y. Qin, L. Duan, L. Wang, Y. Wu, Y. Guo, W. Song and X. Liu, Dalton Trans., 2022, 51, 473–477 RSC.
- L. Fan, W. Fan, B. Li, X. Liu, X. Zhao and X. Zhang, Dalton Trans., 2015, 44, 2380–2389 RSC.
- J.-Z. Li, F. Bigdeli, X.-M. Gao, R. Wang, X.-W. Wei, X.-W. Yan, M.-L. Hu, K.-G. Liu and A. Morsali, Inorg. Chem., 2019, 58, 5397–5400 CrossRef CAS PubMed.
- W. Liu, D. Li, F. Wang, X. Chen, X. Wang and Y. Tian, Opt. Mater., 2022, 123, 111895 CrossRef CAS.
- X.-L. Lv, S. Yuan, L.-H. Xie, H. F. Darke, Y. Chen, T. He, C. Dong, B. Wang, Y.-Z. Zhang, J.-R. Li and H.-C. Zhou, J. Am. Chem. Soc., 2019, 141, 10283–10293 CrossRef CAS PubMed.
-
(a) E. A. Dolgopolova, A. M. Rice, C. R. Martin and N. B. Shustova, Chem. Soc. Rev., 2018, 47, 4710–4728 RSC;
(b) L.-L. Liu, C.-X. Yu, J. Sun, P.-P. Meng, F.-J. Ma, J.-M. Du and J.-F. Ma, Dalton Trans., 2014, 43, 2915–2924 RSC;
(c) H. Li, Y. Han, X. Lv, S. Du, H. Hou and Y. Fan, CrystEngComm, 2013, 15, 3672–3677 RSC;
(d) J. Huang, H. Li, J. Zhang, L. Jiang and C. Y. Su, Inorg. Chim. Acta, 2012, 388, 16–21 CrossRef CAS;
(e) S.-J. Liu, L. Xue, T.-L. Hu and X.-H. Bu, Dalton Trans., 2012, 41, 6813–6819 RSC;
(f) J. Y. Lu, Coord. Chem. Rev., 2003, 246, 327–347 CrossRef CAS.
- J. R. Lakowicz, Principles of Fluorescence Spectroscopy, Kluwer Academic/Plenum Publishers, New York, 2nd edn, 1999 Search PubMed.
- W. Yan, C. L. Zhang, S. G. Chen, L. J. Han and H. G. Zheng, ACS Appl. Mater. Interfaces, 2019, 9, 1629–1634 CrossRef PubMed.
Footnotes |
† Electronic supplementary information (ESI) available: Fig. S1–S15, Tables S1–S3, crystal structures in CIF format. CCDC 2180904 (1), 2180910 (2), 2180911 (3) and 2180912 (4). For ESI and crystallographic data in CIF or other electronic format see DOI: https://doi.org/10.1039/d3ra03817c |
‡ These authors contributed equally to this work. |
|
This journal is © The Royal Society of Chemistry 2023 |
Click here to see how this site uses Cookies. View our privacy policy here.