DOI:
10.1039/D3RA03531J
(Review Article)
RSC Adv., 2023,
13, 18262-18305
A fruitful century for the scalable synthesis and reactions of biphenyl derivatives: applications and biological aspects
Received
26th May 2023
, Accepted 10th June 2023
First published on 16th June 2023
Abstract
This review provides recent developments in the current status and latest synthetic methodologies of biphenyl derivatives. Furthermore, this review investigates detailed discussions of several metalated chemical reactions related to biphenyl scaffolds such as Wurtz–Fittig, Ullmann, Bennett–Turner, Negishi, Kumada, Stille, Suzuki–Miyaura, Friedel–Crafts, cyanation, amination, and various electrophilic substitution reactions supported by their mechanistic pathways. Furthermore, the preconditions required for the existence of axial chirality in biaryl compounds are discussed. Furthermore, atropisomerism as a type of axial chirality in biphenyl molecules is discussed. Additionally, this review covers a wide range of biological and medicinal applications of the synthesized compounds involving patented approaches in the last decade corresponding to investigating the crucial role of the biphenyl structures in APIs.
1. Introduction and scope
For many decades, biphenyl compounds and their isosteres have been considered a fundamental backbone in synthetic organic chemistry and natural products due to their omnipresence in medicinally active compounds, marketed drugs and natural products. For example, there are various biologically active natural products that contain biaryl scaffolds (vancomycin,1,2 WS 43708A3 and Arylomycin A4) (Fig. 1).
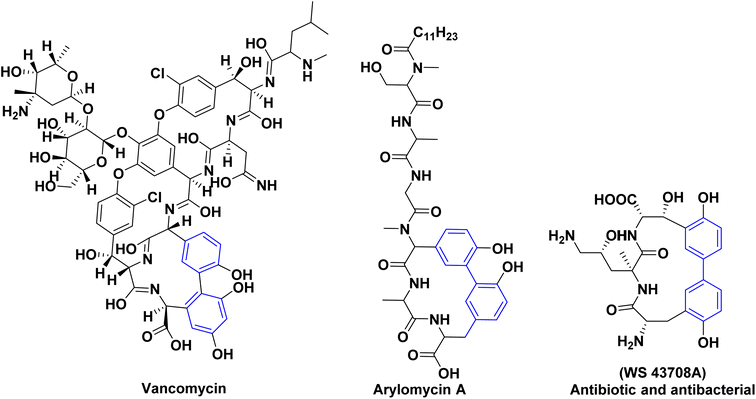 |
| Fig. 1 Exemplary skeletons for natural products containing biphenyl moieties. | |
Since biphenyls are neutral molecules without a functional group, functionalization is required for them to react. Biphenyls consist of two benzene rings linked at the [1,1′] position. The reactions of biphenyls are similar to benzene as they both undergo electrophilic substitution reaction. Biphenyl derivatives which are used to produce an extensive range of drugs, products for agriculture, fluorescent layers in organic light-emitting diodes (OLEDs)5,6 and building blocks for basic liquid crystals are significant intermediates in organic chemistry besides being the structural moiety of an extensive range of compounds with pharmacological activities.7–10 Whereas several biphenyl derivatives serve as versatile and multifaceted platforms in medicinal chemistry, as large number of biphenyl derivatives are patented and broadly used in medicine as the antiandrogenic,11 immunosuppressant, antifungal, antibacterial, antimicrobial, anti-inflammatory, anti-proliferative, osteoporosis, antihypertensive, antitumor, β-glucuronidase inhibition activity, anti-leukemia agent hypotensive, anti-cholinesterase, anti-diabetic and antimalaria drugs.12 In addition, 6-(3-(adamantan-1-yl)-4-methoxyphenyl)-2-naphthoic acid which trademark drug (adapalene) as a third-generation topical retinoid primarily used for treating acne vulgaris, anti-inflammatory, antibacterial.13 Another example is sonidegib which acts as a drug for basal cell carcinoma.14 Additionally, other manufactured naturally occurring chemicals, including the biphenyl nucleus, have shown noteworthy biological activities, such as the antipyretic properties of fenbufen15 and flurbiprofen and their role as non-steroidal anti-inflammatory drugs (NSAIDs) (Fig. 2).16
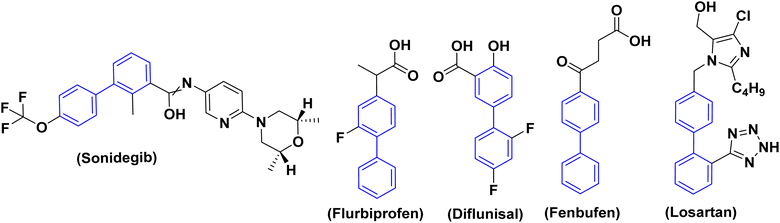 |
| Fig. 2 Representative examples for biphenyl containing marketed drugs. | |
2. Synthesis of biphenyl systems
Wurtz reported the first trial for carbon–carbon bond formation reactions between two alkyl halides in the presence of sodium metal. Then, Fitting expanded this work to include the C(sp2)–C(sp2) homodimerization of aryl halides. Whereby, Ullmann described the Cu-catalyzed homocoupling reaction involving halo-arenes in 1901. In 1914, Bennett and Turner demonstrated the homodimerization of Grignard reagent and phenyl magnesium bromide which promoted by chromium(III) chloride (CrCl3) or anhydrous cupric chloride (CuCl2).17 Kumada depicted the reaction of aryl halides with arylmagnesium halide or organozinc in the presence of transition metal catalysts.18–20 Whereas, Hiyama produced biaryls via coupling the readily available organosilanes with organohalides.21,22 In the same context, Negishi et al.23 reported a regio- and chemoselective method for biaryl synthesis.24,25 In 1986, a new methodology for cross coupling reaction of organic electrophiles with organostannanes was discovered by Stille.26,27 After that, Suzuki–Miyaura cross coupling became one of the most effective and widely used ways for forming carbon–carbon bonds.15,28,29 As biphenyl scaffolds are a key step to produce bioactive molecules used for medicine. In addition, biphenyl compounds were isolated by distillation from natural sources such as crude oil, natural gas, and coal tar.6 Several methods are available in the literature for the synthesis of substituted biphenyl scaffolds through using various chemical building blocks according to the following reaction routes:
2.1 Wurtz–Fittig reaction
The history of the chemistry of biphenyl derivatives can be traced back almost 160 years ago. As the synthesis trials of biphenyl scaffolds started from 1855 till the end of the 19th century by many researchers as in 1855. Wurtz reported the first trial for C(sp3)–C(sp3) bond formation through using the sodium-mediate coupling reaction of two alkyl halides.17 In 1862, the reaction was extended by Fittig to include coupling between aryl anion and an electrophile such as aryl halide in the presence of various metal surface such as Na, Li, Au, Cu and Ag.30 Wurtz–Fittig reaction could be achieved via the free radical mechanism31 in three steps; dehalogenation, followed by diffusion of the dehalogenated molecules, and finally, coupling process between two aryl halides 1 to afford biphenyl derivative 2.32 It is worthy to mention that, bulky substitution group leads to kinetically prevent Wurtz–Fittig coupling as one of its drawbacks.33 Also, Wurtz–Fittig reaction plays an important role in the synthesis of thermoplastics polymer synthesis (polyphenylenes and oligophenylenes) (Scheme 1).34
 |
| Scheme 1 Free radical mechanism for Wurtz–Fittig reaction. | |
2.2 Ullmann reaction
The Ullmann reaction was known since 1901,35 as it has attracted the attention of many chemists trying to synthesize chiral substituted biphenyl. Moreover, the Ullmann reaction usually is achieved at lower temperatures and shorter time frame through coupling the aryl halide 1 in the presence of powdered copper or nickel catalysts, but it has changed in recent years.36,37 It is worth mentioning that, the degree of stability of substituted chiral biphenyl depends on the nature of the ortho substituents when this asymmetric Ullman coupling.38 Whereas, enantioselective biphenyls 2 were obtained via the reaction of ortho-chlorinated benzaldehyde derivatives 1 in the presence of Ni catalyst (Scheme 2).39
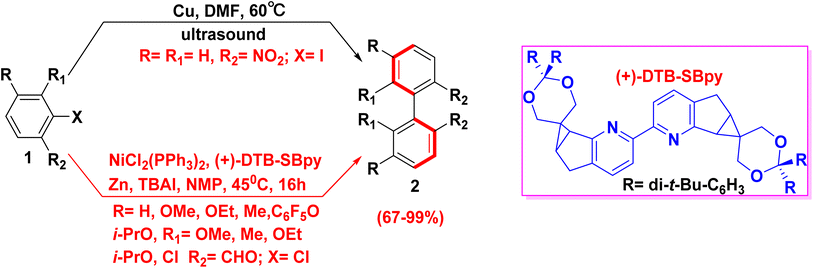 |
| Scheme 2 General Ullman cross-coupling reaction for the synthesis of biphenyl derivatives. | |
2.3 Bennett–Turner
In 1914, the coupling reaction was investigated by Bennett–Turner40 who reported the synthesis of biphenyl compound 2 via homocoupling reaction of phenylmagnesium bromide (3) and CrCl3 in diethyl ether (Et2O), incidental to an attempt to prepare organochromium compound.41 Bennett–Turner reaction can be achieved by using CuCl2.42 In this context, noteworthy is the contribution of Krizewsky with Turner using a CuCl2 promoted homocoupling reaction instead of CrCl3 (Scheme 3).17,42,43
 |
| Scheme 3 Preparation of biphenyls via Bennett–Turner. | |
Ultimately, treatment of the tetrafluoro-3-methoxybenzene (4) with CuCl2 and lithium tert-butoxide (t-BuOLi) in the presence of O2 gas produced a mixture of biphenyl coupling product 5 (56%) and the corresponding phenol 6 (38%). The proposed mechanism involves in situ deprotonation with oxidative dimerization has been studied by Do et al.44 The production of corresponding phenol was reasonable through trapping of the formed aryl lithium with oxygen gave rise to an aryl anion species which was less prone to oxygenation and more likely to form a copper adduct that would undergo oxidative coupling reaction (Scheme 4).
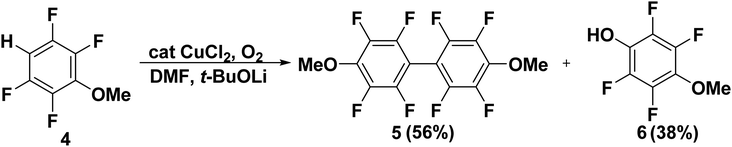 |
| Scheme 4 Copper catalyzed reaction of aryl lithium with oxygen. | |
In 2003, Demir and coworkers reported using of low-cost copper salts (Cu(I) and Cu(II)) as they are able to mediate the dimerization of arylboronic acids 7 to afford the corresponding symmetrical substituted biaryl 2 in good yields. The reaction can be possibly catalyzed under an oxygen atmosphere without a significant loss of yields.45 In 2009, Kirai et al.46 improved the previous protocol for oxidative dimerization which relying on catalysts that would facilitate transmetalation. As they reported a new effective methodology for homocoupling of aryl boronic acid derivatives 7 under stirring conditions through using the catalytic amount of [{(phen)Cu(μ-OH)}2Cl2]·3H2O. It is worthy to mention that the homocoupling reaction proceeds without any additives such as oxidant or base. Besides, this method tolerates different substituents on the arylboronic acids such as nitro group, halogens and carbonyls (Scheme 5).
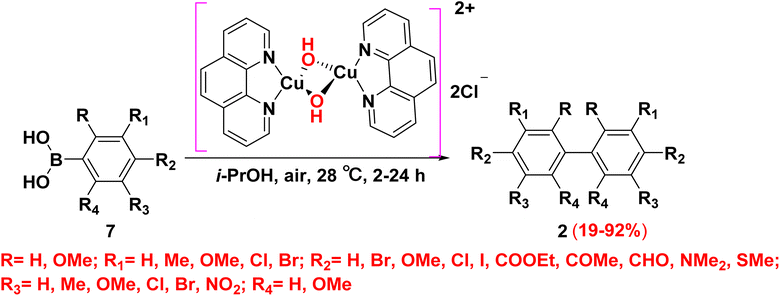 |
| Scheme 5 A reasonable mechanism for the Cu-catalyzed homocoupling of aryl boronic acids. | |
The formation of symmetrical substituted biaryl 2 is explained according to the following postulated mechanism: the reaction proceeds by the hydroxido ligand attacks the oxophilic boron center, followed by transmetalation of arylboronic acids 7 with (μ-hydroxido)copper(II) complex 2A to yield bimetallic arylcopper(II) intermediate 2B without using any base. Biaryl products 2 afford through the concomitant one-electron reduction of each copper center [2LCuII–Ar → 2LCuI + Ar–Ar]. As the binuclear copper models efficiently activate molecular oxygen, in the same time the molecular oxygen smoothly bind to the resulting bimetallic intermediate [{(phen)CuI(μ-Cl)}2] 2C, which is reoxidized to afford (μ-hydroxido)copper(II) complex 2A (Scheme 6). The present binuclear O2 activation and binuclear reductive elimination mechanism is quite different from that proposed for palladium-catalyzed aerobic homocoupling.46
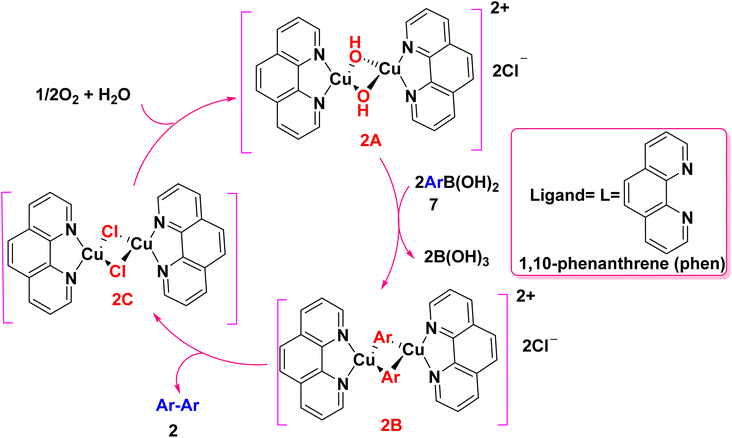 |
| Scheme 6 Possible mechanism for copper catalyzed homocoupling reaction. | |
2.4 Kumada cross coupling
Since 1972, Kumada catalyzed cross coupling reaction of organozinc or organomagnesium with arylhalide in the presence of Pd, Ni, Cu, Fe or Co catalyst.18–20 However, this reaction was limited with Pd catalyst compared to Ni catalyst due to the high reactivity of organomagnesium,47 as well as less toxicity and non-expensive Ni catalyst.48 Besides, arylchlorides were preferable than aryliodides and arylbromides because of low price. Treatment of chloromethylbenzene 8 with (methoxyphenyl)magnesium bromide 9 in the presence of Ni complex and THF afforded 4′-methoxy-2-methyl-biphenyl 10 in (87%) yield.49 Whereas, in the presence of Pd catalyst, product 10 produced in (94%) yield (Scheme 7).50
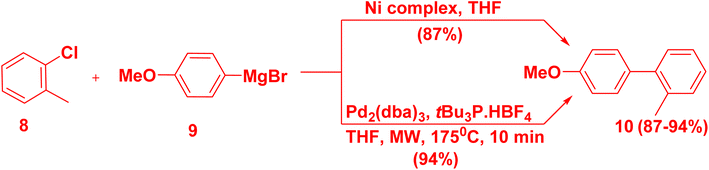 |
| Scheme 7 Kumada cross-coupling reaction for biaryl synthesis. | |
Recent research has largely been focused on directed C–H activation. Primary imines 12 produced by a Grignard reagent's nucleophilic addition to nitrile group 11 which is susceptible to attack make it easier to introduce copper(II) into a close C–H bond. Primary imine derivatives 12 underwent a closure under oxygen-mediated elimination affording the respective phenanthridine derivatives 13 (Scheme 8).51,52
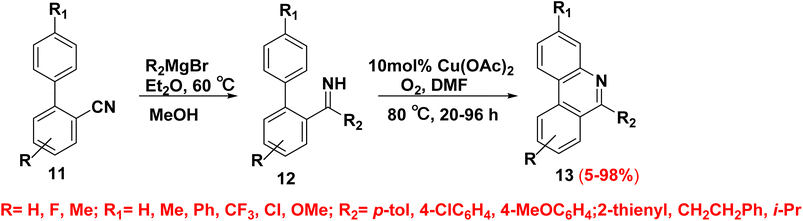 |
| Scheme 8 Copper catalyzed oxidation phenanthridine. | |
The proposed mechanism for the synthesis of the phenanthridine derivatives 13 is illustrated in Scheme 9. This involves the nucleophilic addition of biaryl-2-carbonitriles 11 and Grignard reagents to produce N–H imines 12, followed by their Cu-catalyzed C–N bond led to formation of the aromatic C–H bond, in which molecular oxygen is a necessary component to complete the catalytic process (Scheme 9).51,52
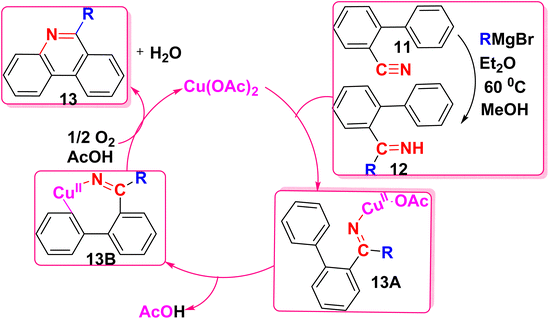 |
| Scheme 9 Mechanism for the oxidative formation of phenanthridine. | |
2.5 Hiyama cross-coupling
One of the most important corn stone for synthesizing biaryl is Hiyama cross-coupling due to the distinctive features of organosilane reagent such as less reactivity, availability, non-toxicity, high sustainability and cheap, in addition to its ability to functionalize stereo and regioselective compounds.53–55 Rhodium-catalyzed o-C–H arylation reaction of arylsilane 14 with ethyl benzimidate 15 with in the presence of pentamethylcyclopentadienyl rhodium dichloride dimer [Cp*RhCl2]2, silver hexafluoroantimonate(v) (AgSbF6), silver oxide (Ag2O) and TBAF produced biphenyl-2-carbonitrile derivative 16.56 Whereby, refluxing a mixture of arylsilane 14 with arylhalide 1 in a mixture of AcOH and toluene, Pd catalyst, and tetrabutylammonium fluoride (TBAF) as a base afforded biphenyl 2. Furthermore, this method was applied under mild condition and tolerated high functional groups (Scheme 10).57–59
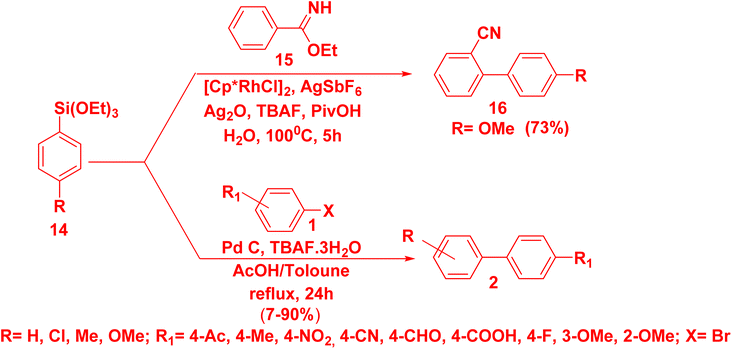 |
| Scheme 10 Synthesis of biphenyls 2 via Hiyama cross-coupling. | |
Noor and coworkers57 suggested the proposed mechanism for Hiyama cross coupling. Analogous to other metal catalyzed cross coupling mechanism, Hiyama cross coupling passed through three steps. The oxidative addition of aryl halide to palladium metal leaded to the conversion of Pd(0) to Pd(ii). Then, transmetallation step occurred in the presence of base such as (KF, n-Bu4NF) which encouraged the C–Si bond to split and establish a new C–Pd bond.53,60 Finally, in the reductive elimination step a new C–C bond was formed and Pd returned back to the oxidation state (0) (Scheme 11).24
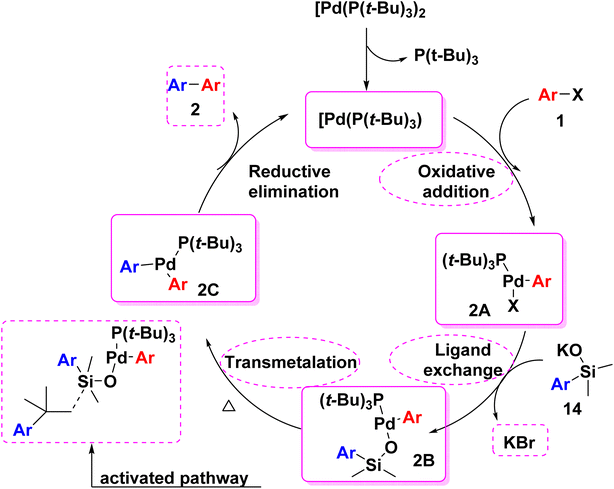 |
| Scheme 11 The postulated mechanism for Hiyama cross-coupling reaction. | |
2.6 Suzuki–Miyaura cross-coupling
In the last decade, Suzuki–Miyaura coupling reaction (SMC) has witnessed an increase interest due to its widespread academic and industrial application in the production of fine chemicals, polymers, materials, and pharmaceuticals.61 SMC reaction is also used to tag DNA with luminous intercalator substituents for DNA sequencing and diagnostics.62 In 1979, Akira Suzuki, Norio Miyaura and Kinji Yamada reported SMC which is the most common method in the formation of carbon–carbon bond in drug discovery.28,29 In the context, the reaction of various aryl bromide derivatives 1 with commercially available aryl boronic acids 7 under action of palladium-catalyzed Suzuki coupling reaction on porous carbon nanospheres (Pd/CNS) afforded biphenyl derivatives 2. It is worthy to mention that, using (Pd/CNS) as catalyst is preferable than other Pd salts due to its highly effective, and lower cost (Scheme 12).63
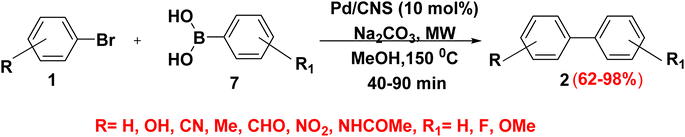 |
| Scheme 12 Suzuki coupling reaction toward synthesis of biphenyl derivatives. | |
The simple mechanism for (SMC) reaction passed through three steps.23,64 The first step is the oxidative addition in which organo palladium(II) complex 2A is formed via coupling of aryl halide 1 with palladium catalyst and this is happening via breaking carbon–halogen bond and by squeezing palladium between the aryl and halogen group. The second step is the transmetalation whereas organoboron compound is converted to nucleophilic borate in the presence of base such as sodium tert-butoxide and carbonate salts then attacking on a Pd(II) complex 2B to form 2C complex. Finally, reductive elimination step in which the two aryl groups are reductively eliminated from Pd(II) complex 2C and combine together to form C–C bond and the palladium is reformed (Scheme 13).65,66
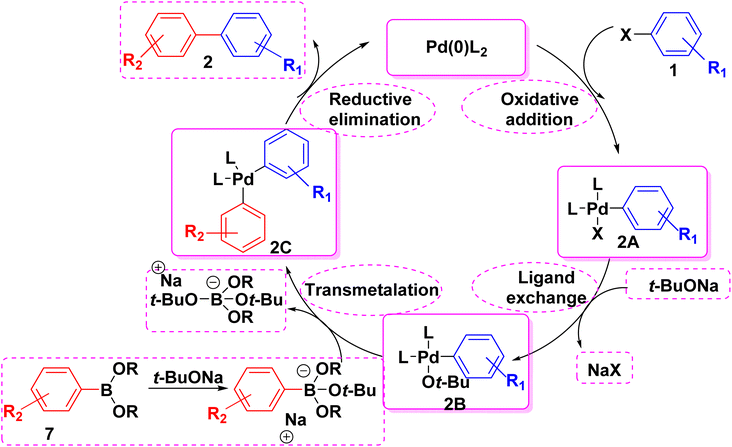 |
| Scheme 13 The postulated mechanism for biphenyl synthesis via Suzuki–Miyaura coupling reaction. | |
Biphenyl phosphine was effective ligand in metal catalyzed reaction as hydroformylation, asymmetric hydrogenation and allylic alkylation. Reaction of equimolar amount of (2-bromophenyl)diphenylphosphine oxide ((2-BrC6H4)OPPh2) 17 with aryl boronic acids 7 in the presence of bis(dibenzylideneacetone)palladium catalyst (Pd(dba)2), triphenylphosphine (PPh3) (4 equiv.) and potassium phosphate (K3PO4) (2 equiv.) as a base in refluxing dioxane at 105 °C yielded biphenyl phosphine oxide derivatives 18 followed by reduction with a mixture of trichlorosilane and catalytic drops of triethylamine (TEA) produced biphenyl phosphine derivatives 19 (Scheme 14).67
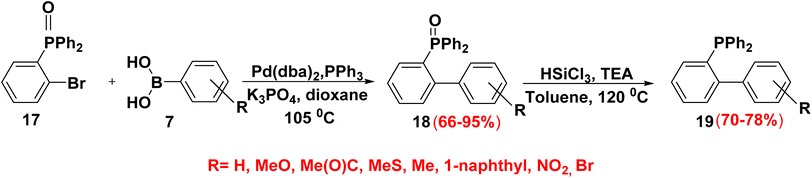 |
| Scheme 14 Synthetic pathway to generate biphenyl-based phosphine. | |
Substituted biphenyl anilines play a vital role in the synthesis of pharmaceutical, dyes, organometallic complexes and ferromagnetic materials due to the ability of amino group to react with aldehyde and ketone to produce Schiff products with strong electronic donor and versatile frameworks.68 Furthermore, treatment of 4-chloroaniline (20) with fluorinated phenyl boronic acid 7 in refluxing a mixture of toluene and water with a catalytic amount of palladium acetate (Pd(OAc)2) and bulky phosphine ligand (SPhos) yielded fluorinated aminobiphenyl derivatives 21.69 Analogously, refluxing of 4-chloroaniline (20) with fluorinated phenyl boronic acid 7 with DMF in the presence of catalytic Pd(OAc)2 and K3PO4 as a base at 80 °C afforded fluorinated aminobiphenyl derivatives 21 (Scheme 15).68
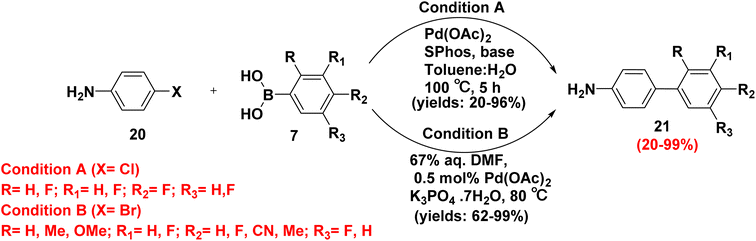 |
| Scheme 15 Preparation of amino biphenyl derivatives. | |
Fluorinated biphenyls are scaffolds for various applications due to their rigidity, chemical stability and electron poor nature. Furthermore, fluorinated biphenyls are used to develop OLEDs, liquid crystal displays (LCDs), organic semiconductors, metal–organic frameworks (MOF), and organic polymer of intrinsic microporosity (OMIMs). In addition, organoflourine as substituents of biphenyl have become widespread drug motifs, as they affect nearly adsorption, metabolism, distribution and excretion properties of lead compounds.70 Bulfield et al.71 prepared a mixture of fluorinated biphenyl compounds 22, 23 via Suzuki–Miyaura cross coupling reaction of tetrafluoroiodobenzene 1 with trifluoroboronic acid 7 in the presence of sodium carbonate (Na2CO3) as a base and tris(dibenzylideneacetone)dipalladium(0) (Pd2(dba)3) as a catalyst with SPhos as a ligand in refluxing with a mixture of THF, toluene, and H2O. Whereas, using XPhos as a ligand and potassium carbonate (K2CO3) as base afforded fluorinated biphenyl 22 only with an excellent yield (99%) (Scheme 16). The reactivity of aryl halides is dependent on the dissociation energy of the C–X bond according to (I > Br > Cl > F). As a larger halogen atom will be a better leaving group due to having a lower bond dissociation, thus increasing the reactivity. As a result of aforementioned information, boronic derivative 7 couples with iodide atom faster than fluorine atom (Scheme 16).72
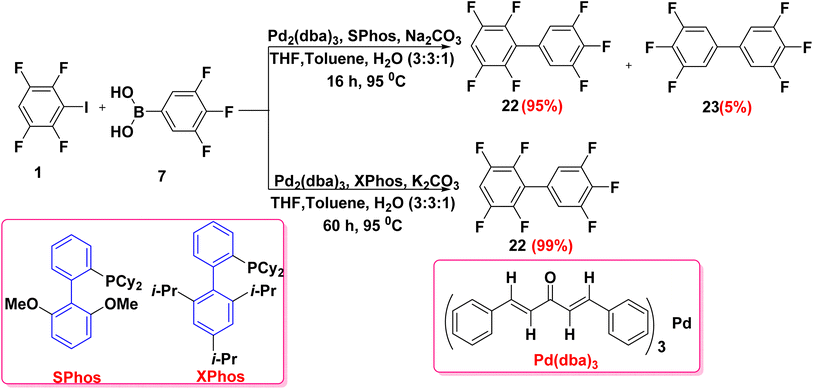 |
| Scheme 16 Preparation of fluorinated biphenyl via Suzuki–Miyaura cross coupling reaction. | |
Whereby, reaction of aryl halide 1 with various fluorinated boronic acids 7 in the presence of palladium catalyst complex and K3PO4 as a base in a refluxing solvent mixture of tetrahydrofuran (THF) and water furnished fluorinated biphenyl 24.6 Additionally, fluorinated biphenyl 26 was synthesized by refluxing iodobenzene 1 and arylboronate 25 in Cu catalyst, phenanthroline ligand (phen) and DMF (Scheme 17).73
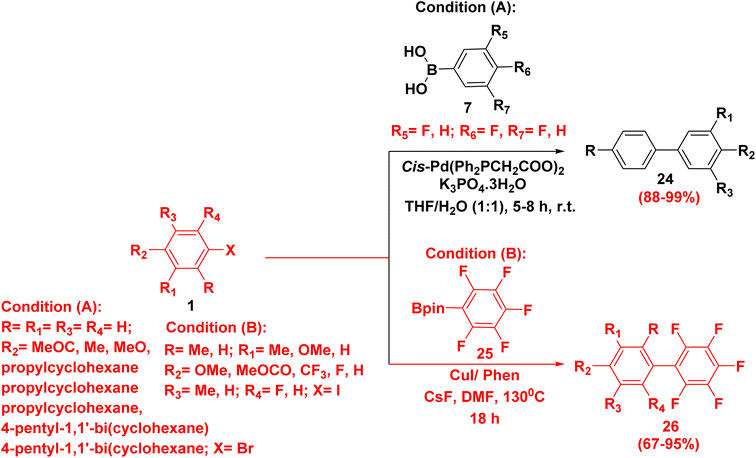 |
| Scheme 17 Formation of fluorinated biphenyls. | |
Palladium catalyst was predominantly used in Suzuki coupling reaction, but due to its scarcity and high price, it was replaced by Ni and Co catalyst. Cobalt salts have stronger catalytic activity and a reduced tendency to yield homocoupling by-products. However, iron was considered the best substitute for a Pd catalyst because it was the least toxic and the most abundant transition metal.74 Biphenylpyrrole derivative 29 was obtained via the reaction of arylhalide 28 with lithium arylboronates 27 in the presence of Fe catalyst and 1,3-bis(trimethylphenyl)-1,3-dihydro-2H-imidazol-2-ylidene (IMes) as a ligand.75 Whereas, the reaction of chloromethylbenzene 30 with 27 in the presence of cobalt(II) chloride (CoCl2) and a ligand/precursor named bis(2,6-diisopropylphenyl)-1H-imidazol-3-ium afforded methylbiphenyl 31 (Scheme 18).76
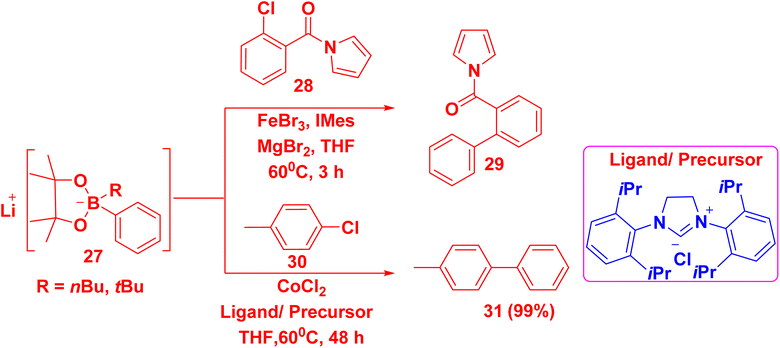 |
| Scheme 18 Iron catalyzed Suzuki–Miyaura cross coupling reaction for biphenyl synthesis. | |
The suggested mechanism involved the formation of a low-valent molecular iron complex that engages in the reversible coordination of both bromide and the pyrrole-containing substrate 28. The C–Cl bond in 28 is reductively activated by the resulting ferrate complex. The biphenylpyrrole product 29 was delivered by reductive elimination, and the active catalyst was renewed by bromide abstraction (Scheme 19).75
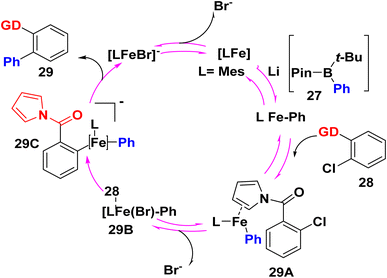 |
| Scheme 19 The suggested mechanism for biphenylpyrrole synthesis. | |
Additionally, nickel catalyzed Suzuki coupling reaction of chlorobenzene derivative 1 with substituted phenylboronic acid 7 in the presence of Ni catalyst, ferrocenylmethylphosphines derivative as a ligand, K3PO4 and THF afforded biphenyl derivative 2 (Scheme 20).77
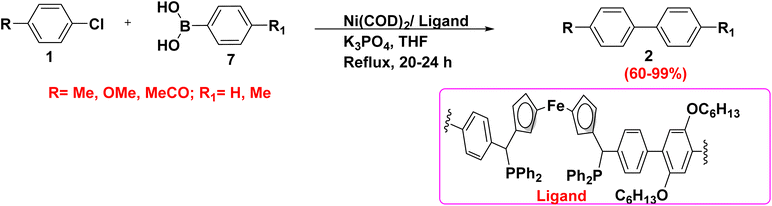 |
| Scheme 20 Synthesis of biphenyl derivatives 2 via nickel catalyzed SMC. | |
Biphenyl tyrosine derivatives are biologically active molecules including arylomycin A2 act as an antibacterial drug. Tyrosine derivatives were keys for the synthesis of biaryl derivatives via SMC reaction. Nevertheless, tyrosine compounds must be protected in order to react. 3-Iodo tyrosine (32) was esterified using methanol (MeOH) with thionyl chloride (SOCl2) to afford 2-amino-3-iodophenyl-propanoate 33. Then, the amino group was protected using tert-butyloxycarbonyl (t-Boc) and sodium bicarbonate (NaHCO3) in MeOH to furnish N-tert-butyloxycarbonyl-3-iodotyrosine methyl ester (34). Refluxing N-tert-butyloxycarbonyl-3-iodotyrosine ester 34 in the presence of Pd(OAc)2, K2CO3 and hetero aryl trifluoroborate salt 35 in MeOH to afford t-butoxycarbonyl-(6-hydroxy-[biphenyl]-yl)propanoate derivatives 36. Finally, deprotection of compounds 36 was achieved via heating them with dil. HCl at 70 °C for 3 h to give the free amino acid 37 (Scheme 21).4
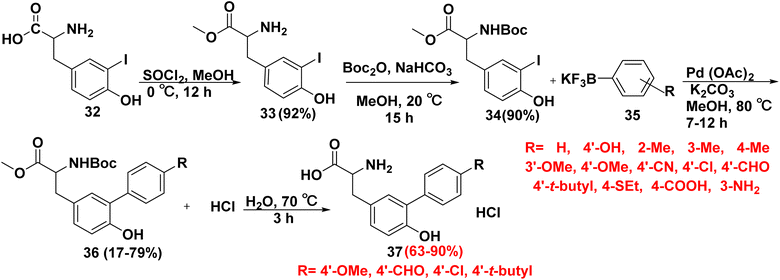 |
| Scheme 21 Synthetic strategy for preparation of biphenyl tyrosine using aryl trifluoroborate salts. | |
Burmaoglu et al.78 synthesized new biphenyl chalcone derivatives 42 according to the reaction sequences. First, preparation of 3-bromo-2,4,6-trimethoxyacetophenone (39) through bromination reaction of 2,4,6-trimethoxy acetophenone (38) with ceric ammonium nitrate (CAN) and lithium bromide (LiBr) in acetonitrile (CH3CN), followed by applying SMC of compound 39 with phenylboronic acid (7) in the presence of Pd(PPh3)4 and K2CO3 in a mixture of ethanol (EtOH) and toluene afforded 2,4,6-trimethoxy-[1,1′-biphenyl]ethan-1-one 40 (Scheme 22). Claisen–Schmidt condensation reaction of substituted biphenyl 40 and benzaldehydes 41 yielded biphenyl chalcone derivatives 42 (Scheme 22). Consequently, biphenyl-substituted chalcone scaffolds which are classified as privileged metabolic enzyme inhibitor motifs which essential support in the treatment of Alzheimer's disease (AD) and glaucoma due to hydrophobic effect and their ability to bind with multiple receptors.
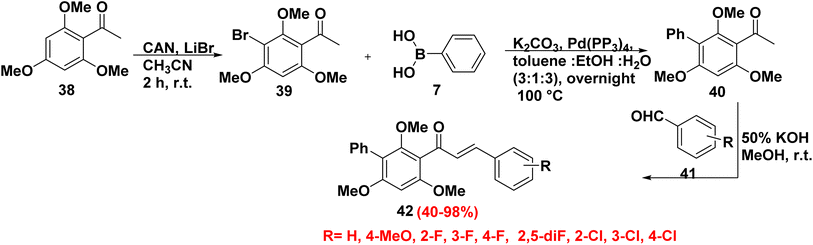 |
| Scheme 22 Formation of biphenyl chalcone derivatives by SMC. | |
Treatment of methyl 2-bromo-6-iodobenzoate (43) with (4-methoxyphenyl)boronic acid (7) in the presence of Na2CO3, bis-triphenylphosphine palladium dichloride (PdCl2(PPh3)2), and a mixture of H2O and THF afforded methyl-3-bromo-4′-methoxy-[1,1′-biphenyl]-2-carboxylate (44). In order to produce biphenyl acetylene derivatives 46, compound 44 was reacted with phenylacetylene derivatives 45
79 (Scheme 23). Diphenylacetylene-based all-trans retinoic acid (ATRA) analogues with enhanced stability may hold tremendous promise as therapies for large number of cancers and neurological conditions.80 In addition, diphenylacetylene derivatives acted as antimicrobial peptide mimetics.81
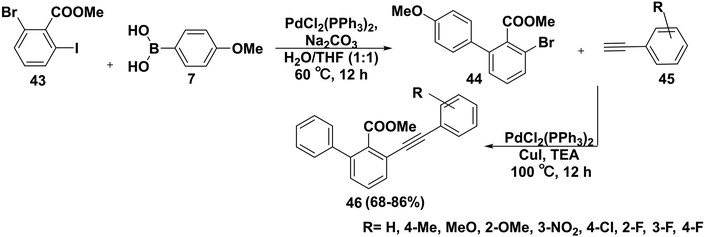 |
| Scheme 23 Synthesis of phenylacetylene derivatives. | |
Over the past decade, olefinic polymerization which catalyzed by transition metals has great prominence in both academic and industrial research.82,83 Due to the recent adoption of high-throughput screening (HTS) methods, the field of olefin polymerization facilitated by Ziegler–Natta catalysts is particularly active.84 A significant number of novel nonmetallocene ligands have been discovered using these techniques for polymerization catalysis. Application of SMC of 2,2′-biphenyldiboronic acid 47 with 2-bromoindene 48 in the presence of tetrakis(triphenylphosphine)palladium [Pd(PPh3)4] furnished polycyclic 2,2′-di(1H-inden-2-yl)-1,1′-biphenyl (49) which showed promising activities in olefin metathesis polymerization (Scheme 24).85
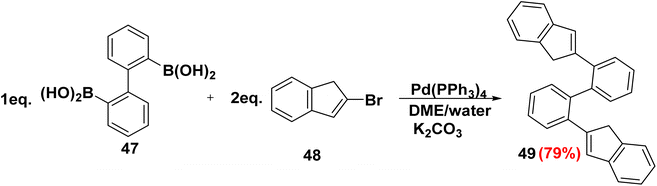 |
| Scheme 24 Synthesis of (1H-inden-2-yl) biphenyl via palladium-catalyzed Suzuki–Miyaura coupling reaction. | |
Polyaromatic hydrocarbons are distinguishable class of aromatic compounds. As aromatic polyphenyl scaffolds give a crowded molecule that usually relieves the congestion by distortion from planarity displaying highly reversible electrochemical properties as this merit shared by a few organic compounds.86 Whereas, a mixture of four different polycyclic products 52–55 were furnished by reaction of 2,2′-diiodo-biphenyl 50 with (1H-inden-2-yl)boronic acid (51) in the presence of palladium catalyst [Pd(PPh3)4] and K3PO4 in refluxing DME/H2O (Scheme 25).85
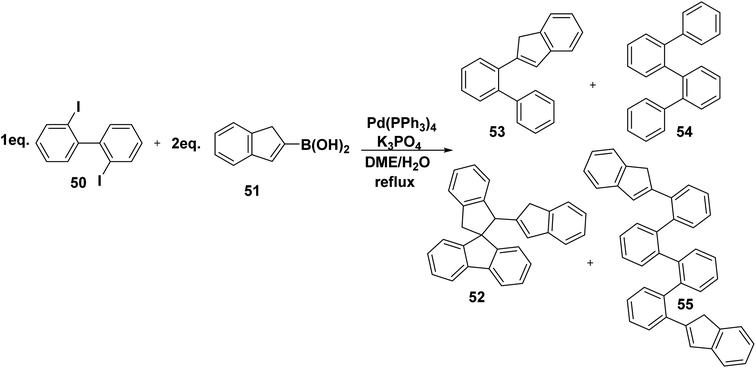 |
| Scheme 25 Synthesis of polycyclic biphenyl compounds. | |
Therefore, a proposed mechanism for the formation of 1H-inden-1′,3′-dihydrospiro[fluorene-9,2′-indene] 52 is depicted in Scheme 26. Initially, a palladium catalyst is inserted between C–I bond, then (1H-inden-2-yl) boronic acid (51) is coupled with (2′-iodo-[1,1′-biphenyl]-2-yl) palladium(II)iodide (52A), followed by intramolecular Heck reaction from 52B to 52C. Finally, Suzuki coupling reaction of (1′,3′-dihydrospiro[fluorene-9,2′-inden]-1′-yl) palladium(II) iodide (52C) with (1H-inden-2-yl) boronic acid (51) to afford 1H-inden-2-yl-dihydrospiro[indene] 52 with 58% yield (Scheme 26).85
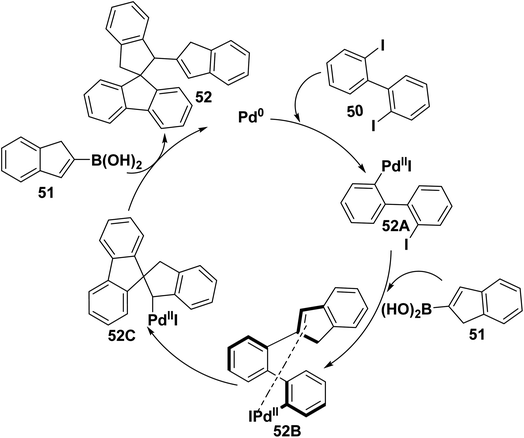 |
| Scheme 26 The proposed mechanism for the formation of polycyclic product 52. | |
Biphenyl benzamide derivatives inhibit the filamenting temperature-sensitive mutant Z (FtsZ) protein, in addition to having antibacterial activities.87 Firstly, 2,6-difluoro-3-hydroxybenzoic acid (56) was alkylated with 3-bromobenzyl bromide (57) in the presence of sodium iodide (KI) and K2CO3 to furnish fluorinated acid derivative 58. Then, fluorinated acid derivative 58 was reacted with oxalyl chloride (COCl)2 in the presence of a mixture of dimethylformamide and dichloromethane (DMF/DCM) to afford difluorobenzoyl chloride derivative 59. The acid was transformed to benzamide 60 by using oxalyl chloride, then treatment with ammonium carbonate ((NH4)2CO3). Finally, biphenyl benzamide derivatives 61 were produced in a wide range of yields (73–89%) via coupling commercially available boronic acids 7 with compound 60 (Scheme 27).87
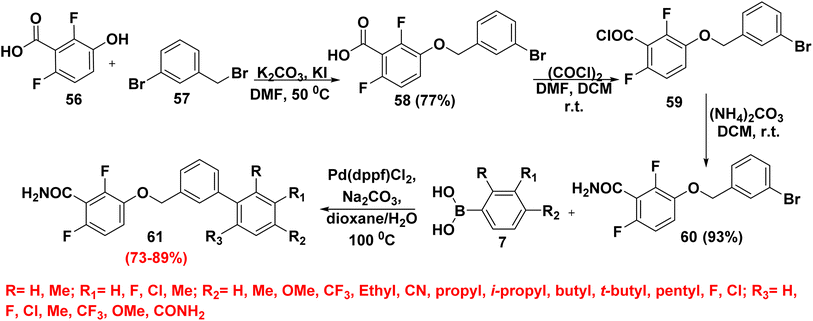 |
| Scheme 27 Formation of biphenyl benzamide derivatives. | |
N-Biphenyl pyrrolidine derivatives are employed as ligand in arylaminations that are catalyzed by palladium. Furthermore, 2′-bromo-2-fluoro-5-nitro-1,1′-biphenyl (63) were produced in (81% yield) via Suzuki–Miyaura cross coupling between 2-iodo 4-nitro fluorobenzene (62) and boronic acid 7 in the presence of palladium catalyst and PPh3 in refluxing dioxane. Then, the fluorine atom was nucleophilically substituted by (R)-(3-N,N-dimethylamino)pyrrolidine (64) in the presence of K2CO3 in DMF to yield 1-(2′-bromo-5-nitro-[1,1′-biphenyl]-2-yl)-N,N-dimethylpyrrolidin-3-amine (65). (R)-[1-(2′-Bromo-5-amino-biphenyl]-2-yl)-pyrrolidin-3-yldimethylamine (66) was produced by refluxing zinc powder and calcium chloride (CaCl2) with compound (R)-65 in EtOH. After that, hypophosphorous acid (H3PO2) was added to compound (R)-66 in a mixture of THF and H2O, followed by treatment with cupper(i)oxide, and sodium nitrite (NaNO2) furnished bromo(biphenyl-2-yl)-dimethylpyrrolidin-3-amine 67. Finally, 2′-(dicyclohexylphosphaneyl)-[1,1′-biphenyl]-2-yl-N,N-dimethylpyrrolidin-3-amine 68 was obtained in two steps involving adding n-butyl lithium dropwise to compound (R)-67 in a mixture of THF and hexane as first step. In the second step, chlorodicyclohexylphosphane (Cy2PCl) was added with stirring to replace bromide by dicyclohexylphosphane group (Scheme 28).88
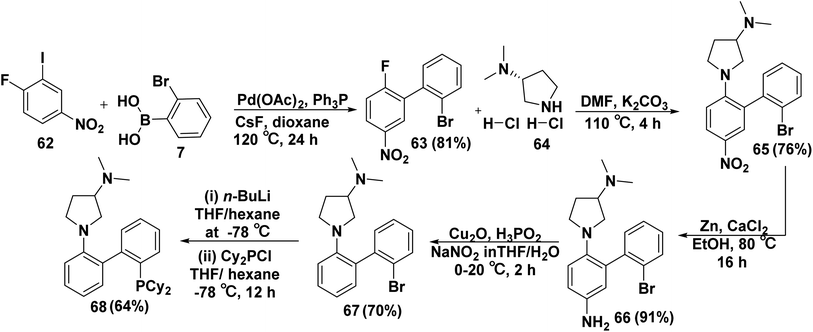 |
| Scheme 28 Synthesis of N-biphenyl pyrrolidine derivatives. | |
The synthesis of biphenyl oxazole derivatives 70 was reported by Ahmad et al.89 under mild conditions which were enzyme inhibitors and estimated as therapeutic seeds for ailments associated with NPP1 and NPP3 isozymes via Suzuki–Miyaura cross coupling of bromo-phenyloxazole 69 with various boronic acids 7 in a mixture solvent system (toluene/water) (Scheme 29).
 |
| Scheme 29 Suzuki coupling reaction for synthesis of biphenyl oxazole derivatives. | |
Moreover, an efficient synthesis of biphenyl oxazole derivatives 77 was achieved through alkylation reaction of 1-bromo-3-(chloromethyl)-2-methylbenzene (71) with 3-chloro-4-hydroxybenzaldehyde (72) produced 4-((3-bromo-2-methylbenzyl)oxy)-3-chlorobenzaldehyde (73). Next, refluxing 4-((3-bromo-2-methylbenzyl)oxy)-3-chlorobenzaldehyde (73) with bis(pinacolato)diboron (B2pin2) (74) in 1,4-dioxane and potassium acetate (CH3COOK) in the presence of 1,1′-bis(diphenylphosphino)ferrocene-palladium(II)dichloride diCrClchloromethane complex [pd(dppf)Cl2CH2Cl2] afforded 3-chloro-4-((2-methyl-3-(tetramethyl-1,3,2-dioxaborolan-2-yl)benzyl)oxy)benzaldehyde (75). Finally, compound 75 was reacted with (3-bromophenyl)isoxazole derivatives 76 via Suzuki coupling reaction yielded biphenyl oxazole derivatives 77 (Scheme 30).90
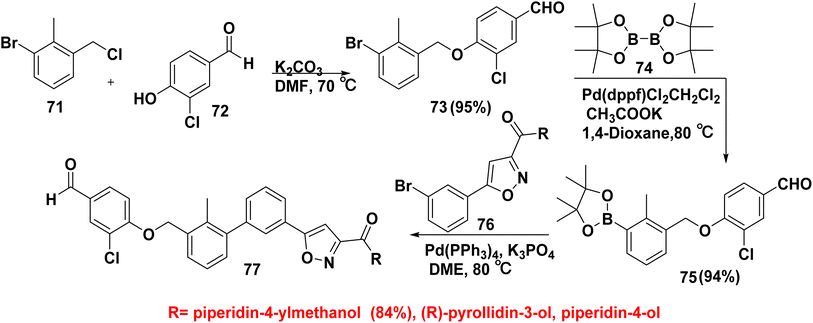 |
| Scheme 30 Synthesis of biphenyl oxazole derivatives. | |
2.7 Stille cross coupling
Stille cross coupling reaction is one-step coupling reaction for nucleosides synthesis was first reported in 1986.26,91 Stille cross coupling is still a significant reaction to form C–C bond, and one of the most selective and general methods for palladium catalyzed cross coupling reaction. Therefore, it was constituted to produce a various ring system bearing functional groups and to synthesize several alkenes, alkenyls, oligoarylenes and biaryls.92–94 The interesting advantage of Stille reaction is the using of organotin compounds which are mild reagents and tolerate numerous of functional groups. On the other hand, the only drawback is the water insoluble tin reagents and toxicity of the hydrophobic.92 Stille cross coupling reaction of aryl halide 1 with triphenyl tin chloride (Ph3SnCl) (78) in the presence of catalytic amount of palladium grafted on natural asphalt sulfonate Na[Pd-NAS], cesium carbonate (Cs2CO3) as a base in refluxing ethanol furnished biphenyl derivatives 2 (Scheme 31).88,95
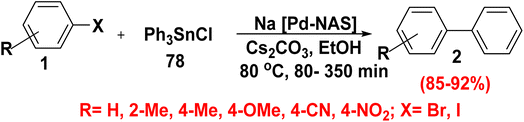 |
| Scheme 31 Synthesis of parent biphenyl via Stille cross coupling. | |
In 1986, Chemie John K. Stille described the possible mechanism for Stille cross coupling reaction. Stille cross coupling mechanism passed through three steps: oxidative addition, transmetalation and reductive elimination. In oxidative addition step, the catalytic species Pd(0)Ln is reacted with aryl halide 1 to form Pd(II) complex 2A. In transmetalation step, Pd(II) complex 2A cleavage the C–Sn bond of organotin reagent 78 to produce complex 2B. Finally, reductive elimination step for forming C–C bond and the catalyst is regenerated at the same time (Scheme 32).96
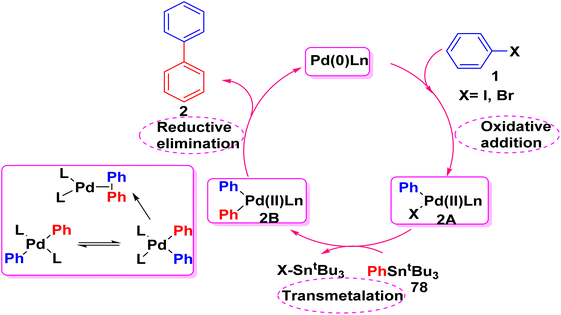 |
| Scheme 32 The proposed mechanistic pathway for Stille cross coupling reaction. | |
2.8 Negishi cross coupling
Negishi cross coupling reaction among the most important in organic chemistry for forming C–C bond between electrophiles with organozinc reagents and organic halides. Negishi reported an easy regio- and chemoselective method for the synthesis of unsymmetrical biaryl using an aryl–aryl coupling process that is catalyzed by nickel salts.25,97 Treatment of cyanophenyl zinc bromide 79 with o-iodobenzoate 1 in the presence of palladium bis(dibenzylideneacetone) (Pd(dba)2) and tris-o-furylphosphine (tfp) furnished biphenyl derivatives 2.98 In 2009, Phapale and Cárdenas99 discovered that Ni-based catalysts (Ni(acac)2/PPh3) facilitate coupling between aryl zinc chlorides 79 and haloarenes 1 affording biphenyl derivatives 2. The reaction of aryl zinc halide 79 and aryl halide 1 in the presence heterogeneous Ni catalyst on charcoal and microwave irradiation afforded biphenyls 2 in acceptable yields (75–95%) (Scheme 33).
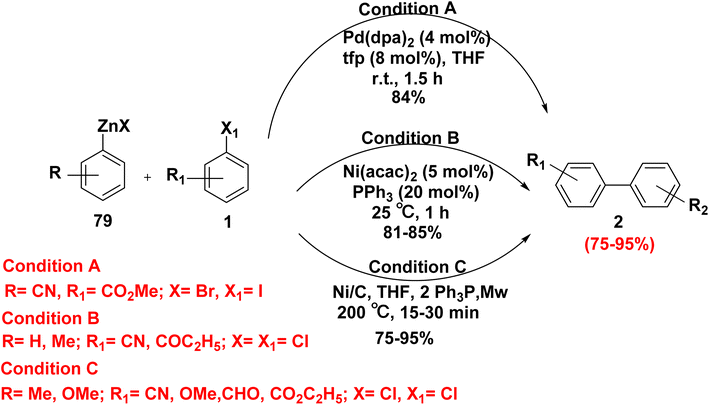 |
| Scheme 33 Synthesis of biphenyl derivatives via Negishi cross coupling reaction. | |
Mechanism of Negishi cross coupling reaction passed through three steps. Initially, an organohalide or pseudohalide (such as triflate) is oxidatively added to a low valent metal complex to produce an organometallic derivative with a higher formal oxidation state on the metal center. Then, producing diorganometal species is processed, whereby, the nucleophilic carbon is transmetalate from the nucleophile to the transition metal complex. Finally, a new C–C bond is formed by further reductive elimination, which reproduces the catalytically active species (Scheme 34).99
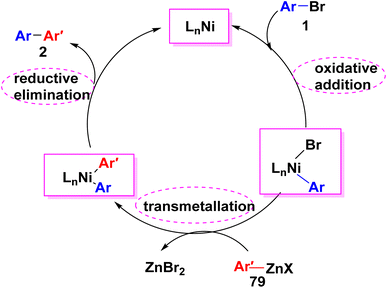 |
| Scheme 34 Plausible mechanism of the formation of biphenyl derivatives via Negishi cross coupling reaction. | |
2.9 Ball-milling method
Recently, direct mechanocatalysis in ball-milling as a highly efficient synthetic tool applied for the cocondensation of alkali metals earth (Ae) such as K, Cs or Rb with benzene afforded C6H5˙− salts and radical coupling to (biphenyl)2− companied with emission of H2 gas. Similarly, the direct dehydrogenative coupling of benzene through low-valent alkaline-earth metals (Ae) intermediates was achieved via formation of metal (Ae) complex with a C6H52− dianion which acts as reducing agent to bulky β-diketiminate ligand BDI 80A to yield [(DIPePBDI)Ca]2(biphenyl) 81 as dianionic N,C-chelating ligand, which rapidly decomposed to afford biphenyl scaffold 2 (Scheme 35).100
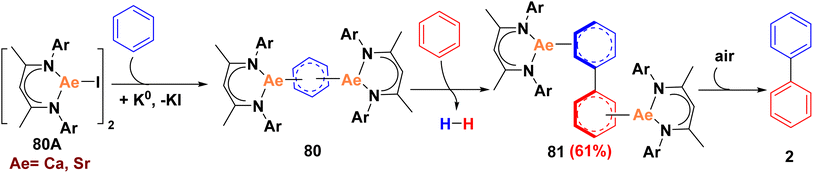 |
| Scheme 35 Dehydrogenative benzene–benzene coupling for biphenyl synthesis. | |
3. Chemical reactions
3.1 The Friedel–Crafts
Friedel–Crafts reactions are subdivided into two fundamental types: alkylation and acylation reactions.101 Whereby, ionic liquids have already been used in a number of Friedel–Crafts processes, which could significantly reduce the associated environmental concerns.102
3.1.1 Friedel–Crafts alkylation of biphenyl with haloalkane. For more than a century, Friedel–Crafts alkylation reaction is a vital reaction for the synthesis of alkyl substituted aromatic compounds.103 4,4′-Di-tert-butylbiphenyl (82) was synthesized from biphenyl (2), which then, in the presence of catalytic amounts of anhydrous ferric chloride (FeCl3), interacted with tert-butyl chloride in halogenated solvents.104 In the same manner, Friedel–Crafts cycloannulation process produced octamethyl-octahydro-2,2′-binaphthalene 83 through adding catalytic amounts of anhydrous AlCl3 to a solution of biphenyl (2) in 1,2-dichloroethane (DCE) followed by dropwise addition of readily available 2,5-dichloro-2,5-dimethylhexane.105 In the same context, a mixture of ammonia and lithium was added dropwisely to a solution of biphenyl (2) in THF till brick red suspension was formed. Then, a quick addition of 1,2-dibromoethane (EDB) was added to afford 2-bromoethyl-1,1′-biphenyl 84.106 A mixture of biphenyl (2), 2-chlorohexane and tributyl phosphine (PBu3) was heated for 6 h to afford 4-(hexan-2-yl)-1,1′-biphenyl (85) (Scheme 36).103
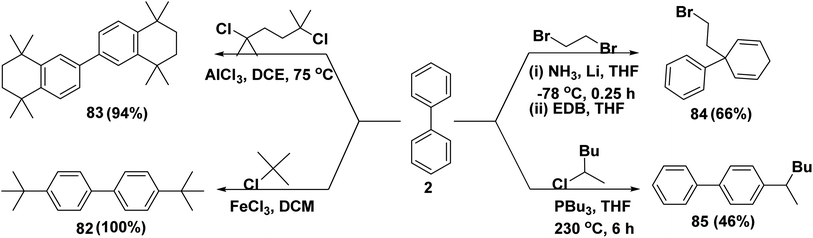 |
| Scheme 36 Friedel–Crafts alkylation of biphenyl with haloalkane. | |
Moreover, treatment of biphenyl (2) with cyclopentene (86) in the presence of AlCl3 and DCE at 0 °C for 24 h afforded 4-cyclopentyl-1,1′-biphenyl (87).107 Additionally, using trichloromethane (CHCl3) instead of DCE at 20 °C for 10 h led to formation of 4,4′-dicyclopentyl-1,1′-biphenyl (88) (Scheme 37).108
 |
| Scheme 37 Reaction of biphenyl with cyclopentene. | |
3.1.2 Friedel–Crafts acylation. The Friedel–Crafts acylation is the reaction of acid anhydrides or acyl chlorides with aromatic compounds in the presence of Lewis acid catalyst for the synthesis of methyl aryl ketone, which are used in pharmaceuticals and fine chemical such as ibuprofen, naproxen, dyes, agrochemicals, and fragrances.109,110
3.1.2.1 Reaction of biphenyl with acid anhydride. 4-Phenylbenzoyl-propionic acid (89) can be easily prepared via Friedel–Crafts acylation of biphenyl (2) and succinic anhydride in the presence of AlCl3 and dichloromethane (DCM).111 While, treatment of biphenyl (2) with dihydro-2H-pyran-2,6(3H)-dione in the presence of AlCl3 and nitrobenzene (C6H5NO2) afforded biphenyl-4-yl-5-oxopentanoic acid (90).112 Further, biphenyl-4-carbonyl-benzoic acid (91) was synthesized through free-solvent mechanochemical ball milling method via reaction of biphenyl (2) with phthalic anhydride in the presence of AlCl3.113 Whereas, gaseous boron trifluoride (BF3) was passed through a homogenous mixture of biphenyl (2) and acetic anhydride to synthesize biphenyl-4-yl-2,2-difluoro-6-methyl-dioxaborinine (92) (Scheme 38).114
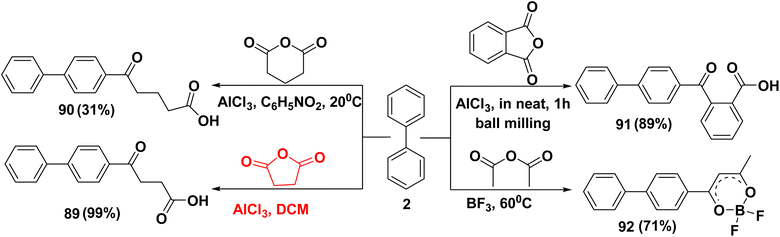 |
| Scheme 38 Friedel–Crafts acylation of biphenyl with anhydrides. | |
3.1.2.2 Reaction of biphenyl with acid chloride. Reaction of biphenyl (2) with a mixture of activated iron(III)oxide (Fe2O3) and acetyl chloride, 1-([1,1′-biphenyl]-4-yl)ethan-1-one (93) was produced.110 In contrast, acetyl chloride in dried carbon disulfide (CS2) was added drop by drop with stirring into a suspension solution containing biphenyl (2), anhydrous AlCl3, and dried (CS2) to obtain 1,1′-([1,1′-biphenyl]-4,4′-diyl)bis(ethan-1-one) (94).115 Additionally, biphenyl (2) was refluxed with propionyl chloride for 4 h in DCM containing a catalytic amount of AlCl3 to furnish 1-([1,1′-biphenyl]-4-yl) propan-1-one (95),116 whereas 1,1′-([1,1′-biphenyl]-4,4′-diyl)bis(propan-1-one) (96) was produced by refluxing biphenyl (2) with propionyl chloride for 2 h at −5 °C.117 Under anhydrous conditions, a stirred mixture of CS2, biphenyl (2), and anhydrous AlCl3 was treated with pentanoyl chloride to afford 1,1′-biphenyl-4-pentan-1-one (97) (Scheme 39).118
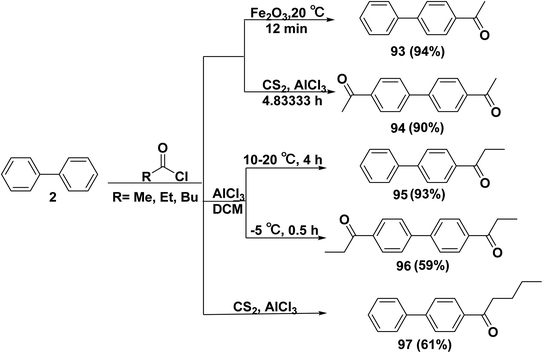 |
| Scheme 39 Reaction of biphenyl with acyl chloride derivatives. | |
The proposed mechanism for Friedel–Crafts acylation shows that the reaction began with the Lewis acids MCl3 (M = Fe or Al) activating the acetyl chloride to produce an adduct of acyl chloride with MCl3 (93A). This complex is quickly equilibrated with the acylium ion [CH3CO]+[MCl4] (93B), which then attacks biphenyl (2) to produce biphenyl alkyl ketone 93 (Scheme 40).102
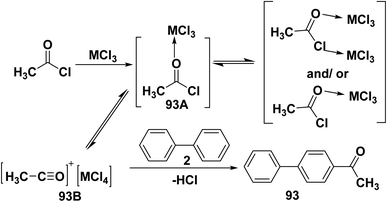 |
| Scheme 40 Friedel–Crafts acylation mechanism. | |
3.1.2.3 Reaction of biphenyl with ketene. 1-([1,1′-Biphenyl]-4-yl)ethan-1-one (93) was synthesized via Friedel–Crafts reaction by stirring ethenone (98) with a solution of biphenyl (2) in CS2, then adding AlCl3 (Scheme 41).119
 |
| Scheme 41 Reaction of biphenyl (2) with ethenone. | |
3.1.2.4 Reaction of biphenyl with oxalyl chloride. A facile synthesis of 4,4′-biphenyl ketone 99 was accomplished via the reaction of biphenyl (2) with oxalyl chloride (ClCO)2 in the presence of AlCl3 and DCM.120 While, the reaction of biphenyl (2) with oxalyl chloride under the influence of AlCl3 in DCE produced 1-([1,1′-biphenyl]-4-yl)ethan-1-one (93) and 1,2-di([1,1′-biphenyl]-4-yl)ethane-1,2-dione (100) (Scheme 42).121
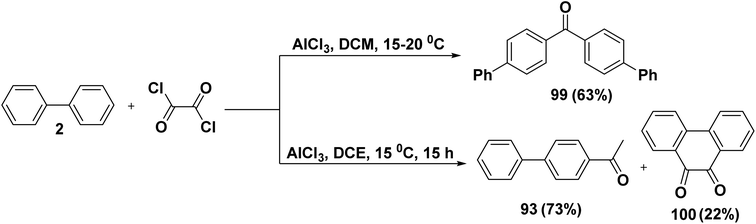 |
| Scheme 42 Acylation of biphenyl with (ClCO)2. | |
3.2 Isopropylation reaction of biphenyl
Reaction of biphenyl with propene (101) in the presence of lithium metal (Li), and THF, followed by hydrolysis afforded a mixture of 4-isopropyl-1,4-dihydro-1,1′-biphenyl (102) and 4-isopropyl-3,4-dihydro-1,1′-biphenyl (103).122 Furthermore, a mixture of 4-isopropyl-1,1′-biphenyl (104), 4,4′-diisopropyl-biphenyl (105), and 3,4′-diisopropyl-1,1′-biphenyl (106) were produced through the reaction of biphenyl (2) with propene (101) in the presence of [Fe]-SSZ-24 catalyst (Scheme 43).123
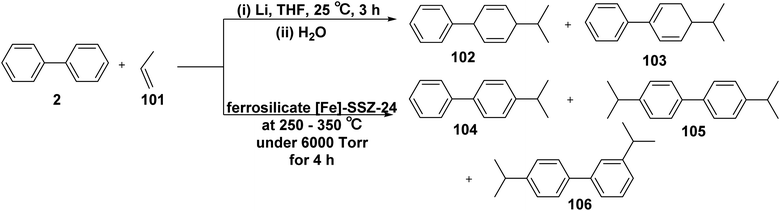 |
| Scheme 43 Reaction of biphenyl with propene. | |
The proposed mechanism for the synthesis of isopropyl biphenyl derivatives starts with in situ formation of carbolithiation step in which the biphenyl (2) is doubly reduced in a mixture of lithium metal (Li) and THF affording biphenyl dianion intermediate 102A. Then, biphenyl dianion 102A reacted with propene (101) in THF, followed by addition step to yield intermediate 102B, finally the hydrolysis step is achieved to furnish 4-isopropyl-1,4-dihydro-1,1′-biphenyl (102) and 4-isopropyl-3,4-dihydro-1,1′-biphenyl (103) (Scheme 44).122
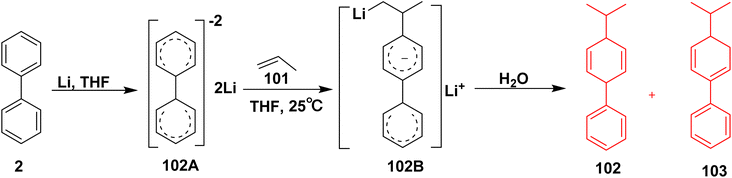 |
| Scheme 44 A postulated mechanism for biphenyl isopropyl synthesis. | |
3.3 Halogenation reactions
3.3.1 Chlorination reactions. Polychlorinated biphenyls (PCBs) are a class of chlorinated organic compounds which were widely used in commercial and industrial applications. PCBs have many industrial applications including heat transfer, electrical, lubricants, and hydraulic equipment; as plastics, rubber products, coating, adhesive, capacitors, and plasticizers in paints; carbonless copy paper, and dyes.124 A solution of biphenyl (2) and anhydrous tin(IV)chloride (SnCl4) in DCM was stirred, then lead tetraacetate Pd(OAc)4 was added in portion to produce 4-chloro-1,1′-biphenyl (107).125 Whereby, dichlorobiphenyl 108 was synthesized through passing chlorine gas (Cl2) to a solution of biphenyl (2) in glacial acetic acid (AcOH).126 On the other hands, biphenyl (2) was exposed to chlorine gas to obtain poly chlorinated biphenyl derivative 109 (Scheme 45).127
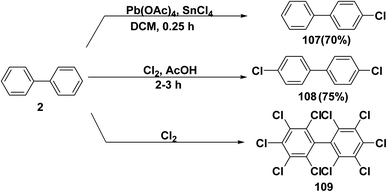 |
| Scheme 45 Chlorination of biphenyl. | |
3.3.2 Bromination reactions. On the area of research, the study of bromine containing molecules has been found to have an extensive range of biological activities, including those that are antiparasitic, antibacterial, antiviral, antioxidant, anti-inflammatory, and anticancer. Furthermore, mixtures of polybrominated biphenyls (PBBs) have been employed as additives in polymers as well as flame retardants in the textile and electronic sectors.128,129 Stirring of biphenyl (2) with N-bromosuccinimide (NBS) in 2-methyltetrahydrofuran (2-MTHF) as solvent furnished mono bromobiphenyl 110.130 Whereas, the bromination of compound 2 by NBS in the presence of AgSbF6 and Trip-SMe in DCE furnished dibromo-biphenyl 111.131 Further, bromine gas (Br2) was mixed with biphenyl (2), cooled in an ice bath, and AlBr3 was added dropwise under the synthesis of HBr to afford PBBs 112, 113 (Scheme 46).129
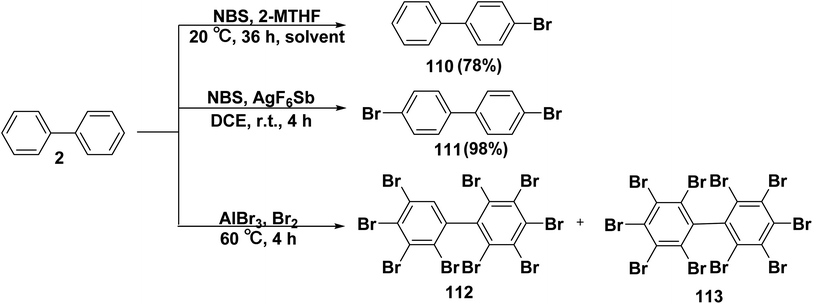 |
| Scheme 46 Bromination of biphenyl. | |
3.3.3 Iodination reactions. Iodo-organic molecules are extensible and useful synthetic intermediates which have numerous applications in pharmacology, medicine, and polymer science.132 Lithiation of biphenyl (2) with n-butyllithium (n-BuLi) in the presence of tetra methyl ethylenediamine (TMEDA) led to formation of 2,2′-dilithiobiphenyl (114). Then, dilithiobiphenyl 114 reacted with iodine gas (I2) in THF to give 2,2′-diiodobiphenyl (115).133,134 On the other hand, a mixture of biphenyl (2), water, AcOH, concentrated sulfuric acid (H2SO4), I2, periodic acid (HIO4), and carbon tetrachloride (CCl4) was heated for 4 h to produce diiodobiphenyl 116.135 Furthermore, stirring of biphenyl (2) with dichloroiodoisocyanuric acid (DCICA) in CH3CN afforded 4-iodo-1,1′-biphenyl (117) (Scheme 47).132
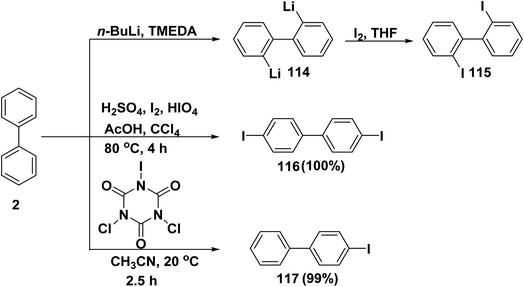 |
| Scheme 47 Different iodinated derivatives of biphenyl. | |
3.4 Chloromethylation reaction of biphenyl
Chloromethyl-substituted aromatic compounds are significant intermediates due to their easy transformation into a wide range of fine or special chemicals, pharmaceuticals, and polymers.136 The chloromethylation reaction of biphenyl (2) with formalin (HCHO) in the presence of HCl and FeCl3 produced 4-(chloromethyl)-1,1′-biphenyl (118) in 80% yield.137 Whereas, 4-(chloromethyl)-1,1′-biphenyl (118) was afforded in 78% yield through the treatment of biphenyl (2) with dimethoxymethane (CH2(OCH3)2) and chlorosulfonic acid (ClSO3H) in the presence of a catalytic amount of zinc iodide (ZnI2) in DCM (Scheme 48).136
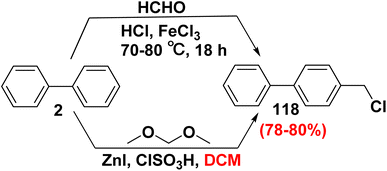 |
| Scheme 48 Chloromethylation of biphenyl. | |
Scheme 49 depicts a probable mechanism for the formation of chloromethyl-1,1′-biphenyl 118. The first step involves treatment of CH2(OCH3)2 with ClSO3H affords methyl chloromethyl ether. Then, ZnI2 promotes the production of the chloromethyl cation ([ClCH2]+). Finally, a chloromethylated derivative 118 is obtained by the electrophilic substitution reaction of biphenyl 2 with [ClCH2]+ (Scheme 49).136
 |
| Scheme 49 Possible mechanism of chloromethylation of biphenyl. | |
3.5 Reduction of biphenyl
Reduction of biphenyl (2) with reducing lithium metal and ammonia afforded tetrahydro-1,1′-biphenyl 119.138 Analogously, 3-phenyl-1,4-cyclohexadiene (120) was synthesized when liquid ammonia was added to Et2O containing biphenyl (2), followed by the addition of lithium metal.139 While, the treatment of biphenyl (2) with a noble metal catalyst (e.g., Ru/C) in the presence of Lewis acid (Hf(OTF)4) and octane (OCT) furnished 1,1′-bi(cyclohexane) (121).140 Whereas, nickel catalyzed hydrogenation of biphenyl (2) in the presence of isopropyl alcohol (i-PrOH) as a hydrogen donor afforded cyclohexylbenzene (122).141 Whereby, reduction of biphenyl 2 with a combination of samarium dibromide (SmBr2) and N-methylethanolamine (NMEA) as a chelating ligand furnished a mixture of 120 and 122 (Scheme 50).142
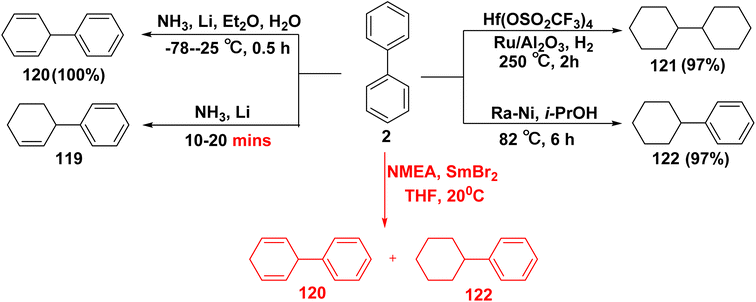 |
| Scheme 50 Reduction of biphenyl. | |
3.6 Reaction of biphenyl with triazine derivatives
4,6-Di([1,1′-biphenyl]-4-yl)-1,3,5-triazin-2-amine (124a) and 4,6-di([1,1′-biphenyl]-4-yl)-1,3,5-triazin-2-ol (124b) were synthesized by heating 4,6-dichloro-1,3,5-triazin derivatives 123 with a solution of o-dichlorobenzene (ODCB) with AlCl3, followed by adding a biphenyl solution of ODCB.143,144 In addition, biphenyl (2) was allowed to react with 2,4,6-trichloro-1,3,5-triazine (123) in the presence of hydrogen chloride (HCl) and AlCl3 in chlorobenzene yielded trisubstituted-6-chloro-1,3,5-triazine 124a–c (Scheme 51).145
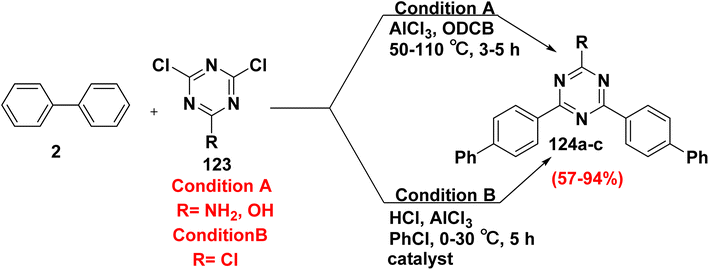 |
| Scheme 51 Formation of biphenyl triazine scaffolds. | |
3.7 Rection of biphenyl with acid
Acetylation of arenes is a significant reaction and still essential need in chemistry. Oxygenated arenes, for example aryl acetates, are valuable synthetic precursors for pharmaceuticals, natural products, and functional materials.146 Luke et al.147 investigated the use of β-aryl-β-ketophosphonates as bone anabolic agent, thyroid receptor ligands, and tumor cell profiler inhibitors. Dimethyl(2-([biphenyl]-4-yl)-2-oxoethyl)phosphonate 126 was synthesized by stirring biphenyl (2) with trifluoroacetic anhydride (TFAA), which was then treated with dimethylphosphonoacetic acid (125) and phosphoric acid (H3PO4). Under purified nitrogen condition, a mixture of sodium nitrate (NaNO3) in trifluoroacetic acid (TFA), ACOH, and Ac2O was mixed with a solution of biphenyl (2) afforded [1,1′-biphenyl]acetate (127).146 Erbium(III) triflate (Er(OTf)3) catalyzed Friedel–Crafts acylation of biphenyl (2) using acylating agent, such as benzoic acid (125) under microwave irradiation furnished [1,1′-biphenyl]-4-yl(phenyl)methanone (128).148 Furthermore, (3-amino-phenyl)-biphenyl-4-yl-methanone (129) was obtained by adding 3-aminobenzoic acid (125) and polyphosphoric acid (PPA) to biphenyl (2) (Scheme 52).149
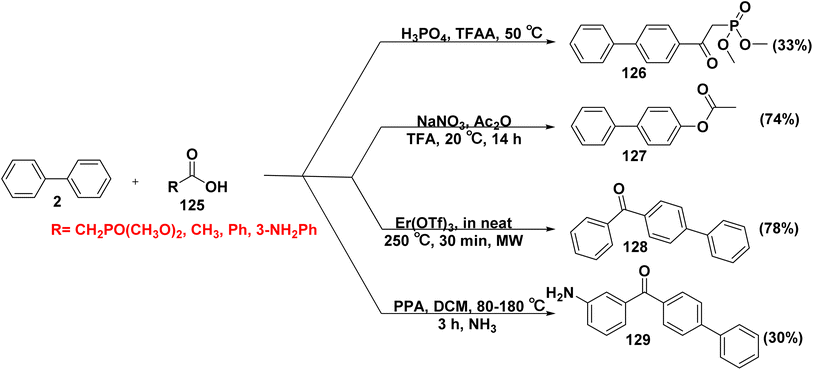 |
| Scheme 52 Acetoxylation of biphenyl with various acids. | |
3.8 Rection of biphenyl with diazonium salt
Manchoju et al.150 emphasized that several tetronic acid derivatives displayed a wide range of pharmacological activities, including acaricidal, insecticidal, HIV-I protease inhibitory, anti-inflammatory, antineoplastic, and cyclooxygenase inhibitory activity. Under conventional heating, synthesis of 1,1′-bipheny-4-hydroxyfuran-2(5H)-one 131 was achieved in excellent yield (51%) by reaction of biphenyl (2) and 3-diazofuran-2,4-dione 130. Gomberg–Bachmann–Hey reactions of benzendiazonium tetrafluoroborate derivatives 132 with biphenyl (2) in the presence of copper catalyst and cesium carbonate (Cs2CO3) in CH3CN afforded terphenyl derivatives 133.151 In the same context, heterogeneous gold(I)-catalyzed reaction of biphenyl (2) with 2-chlorophenyl-2-diazoacetate 134 afforded methyl 2-([biphenyl]-4-yl-2-chlorophenyl)acetate 135 (Scheme 53).152
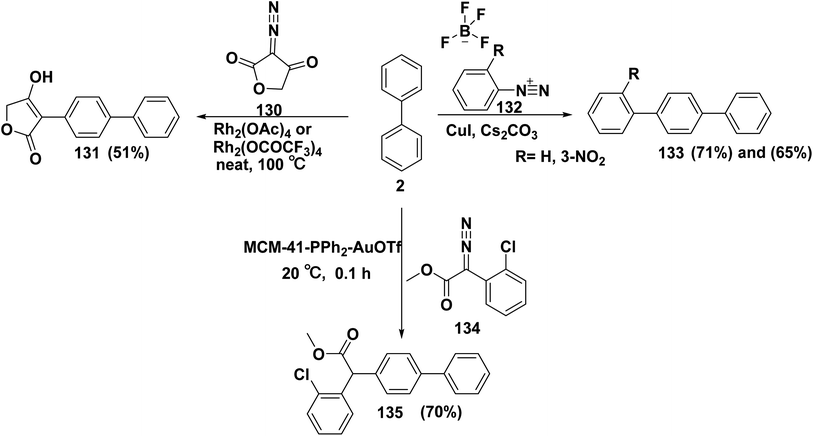 |
| Scheme 53 Reaction of biphenyl with diazonium salts. | |
Heterogeneous gold(I)-catalyzed mechanism of inactivated arenes is passed via sequence steps. Initially, for the synthesis of diazonium ion intermediate 135A, the cationic gold MCM-41–PPh2–AuOTf exchanges ligands with the diazo ester 134. Then, intermediate 135A collapses to produce the MCM-41-bound gold(I)carbene intermediate 135B, followed by combination between the nucleophilic arene 2 and the electrophilic gold(I)carbene intermediate 135B, the gold ionic intermediate 135C is produced. Last, intermediate 135C goes through 1,4-H shift and deauration to afford product 135 and MCM-41–PPh2–AuOTf complex is regenerated (Scheme 54).152
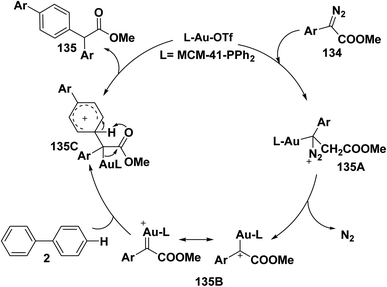 |
| Scheme 54 Heterogeneous gold(I)-catalytic cycle mechanism. | |
3.9 Reaction of biphenyl with isothiazol-3(2H)-one-1,1-dioxide derivatives
Copper-catalyzed reaction of biphenyl (2) with saccharin derivatives 136 in the presence of 1,10-phenanthroline (phen), K2CO3, and selectfluor as oxidant in refluxing CH3CN furnished biphenyl saccharin derivatives 137 (Scheme 55).153
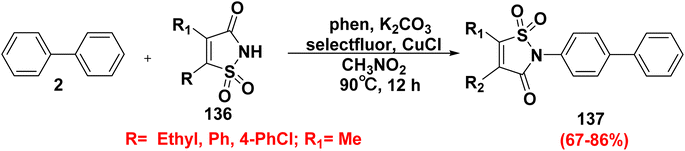 |
| Scheme 55 Formation of biphenyl saccharin derivatives. | |
3.10 Nitration of biphenyl
Several reagents could be used to perform the nitration of aromatic compounds. The nitro aromatic compounds are extensively used in the manufacture of perfumes, pharmaceuticals, explosives, plastics, and dyes. Nitration of biphenyl (2) gave various oriented nitrated products 138 and 139 depending upon the nature of conditions and reagents applied on the reaction, as the nitration of biphenyl (2) was employed by refluxing NaNO3with SO3H-functionalized magnetic core/shell nano catalyst in DCM furnished 4-nitro-1,1′-biphenyl (138).154 While, treatment of a biphenyl with nitric acid (HNO3) in CCl4 solvent afforded 2-nitro-biphenyl 139 (Scheme 56).155
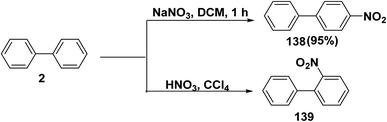 |
| Scheme 56 Nitration of biphenyl. | |
3.11 Reaction of biphenyl with fused compounds
A class of fused ring, such as thioxanthone, dibenzothiophene, and thianthrene are applied as cationic photo-initiator, specifically for usage in surface coating applications, for example, varnishes and inks. 5-([1,1′-biphenyl]-4-yl)-5H-dibenzo[b,d]thiophen-5-ium-hexafluorophosphate(v) (141) was obtained in two steps (Scheme 57) starting of mixing biphenyl (2) and dibenzo[b,d]thiophene 5-oxide (140) with AcOH and Ac2O in DCM, followed by adding sulfuric acid (H2SO4) dropwise. In the second stage, the intermediate product was neutralized by potassium hexafluorophosphate (KPF6) in water. Under the same conditions, compounds 143 and 145 were produced by reacting biphenyl (2) with thianthrene 5-oxide (142) and 2-chloro-9H-thioxanthen-9-one 10-oxide (144), respectively (Scheme 57).156
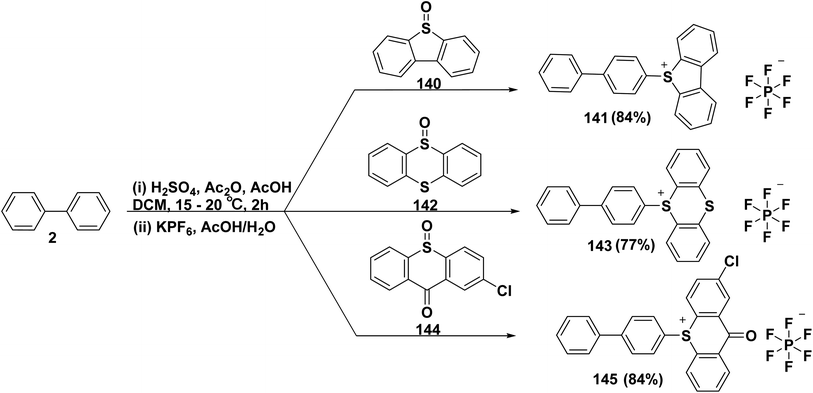 |
| Scheme 57 Reaction of biphenyl with fused compounds. | |
3.12 Reaction of biphenyl with phenol
Terphenyl-3,5-diol 147 was synthesized through stirring phloroglucinol (146) with AlCl3 in chlorobenzene (PhCl), followed by adding biphenyl (Scheme 58).157
 |
| Scheme 58 Reaction of biphenyl with phenol. | |
3.13 Rection of biphenyl with sulfonanilide derivatives
The development of new methods for C–N bond synthesis is of great importance due to the prevalence nitrogenous compounds in numerous synthetic intermediates, natural products, pharmaceutical agents, and biologically active molecules. Sulfonanilides are a crucial class of synthetic scaffolds used in organic synthesis due to their potent electron-withdrawing effect of the connected sulfonyl group, in addition to, sulfonanilides have a special reactivity toward hypervalent iodine(III). Biphenyl sulfonanilide derivatives 149 were produced in wide range yields (33–87%) by adding meta-chloroperbenzoic acid (mCPBA) to a stirred solution of sulfonamide derivatives 148, biphenyl 2, and iodobenzene (PhI) in a mixture of hexafluoroisopropanol (HFIP) and DCM (Scheme 59).158
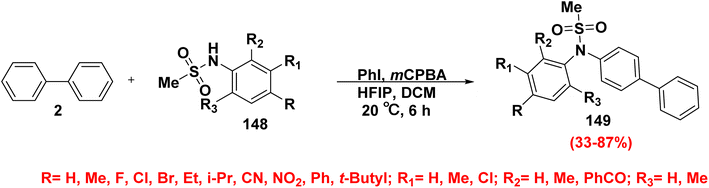 |
| Scheme 59 Synthesis of biphenyl sulfonanilide derivatives. | |
3.14 Amination reaction
Primary aromatic amines are important building blocks for the formation of biologically active pharmaceutical and agrochemical chemicals, as well as organic functional materials like dyes and pigments. Electrochemical oxidation of biphenyl (2) in a solution of tetrabutylammonium tetrafluoroborate (Bu4NBF4) and a mixture of CH3CN and pyridine followed by adding piperidine afforded aminobiphenyl 150 (Scheme 60).159 Analogously, under an oxygen atmosphere conditions, the reaction of ammonium carbamate (H2NCO2−H4N+) as a nucleophile with biphenyl (2) as the arene coupling partner in the presence of a catalytic amount of mesityl acridinium salt, 2,2,6,6-tetramethylpiperidine-1-oxyl (TEMPO), and a mixture of DCE and H2O afforded para and ortho aminobiphenyl products 150 and 151 respectively (Scheme 60).160
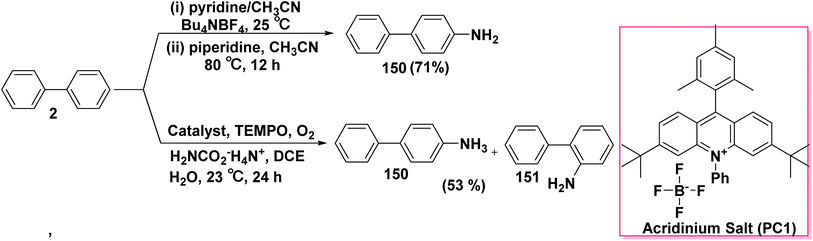 |
| Scheme 60 Reaction of biphenyl with different amines. | |
A proposed mechanism for 4-aminobiphenyl (150) synthesis involves the nucleophilic attack of pyridine on biphenyl (2), followed by one-electron oxidation to produce the intermediate ion 150A which is underwent aromatization process to yield N-arylpyridinium ion intermediate 150B. Then, piperidine is added to the 2-position of the N-arylpyridinium ion 150B, accompanied by ring opening and imine hydrolysis (Scheme 61).159
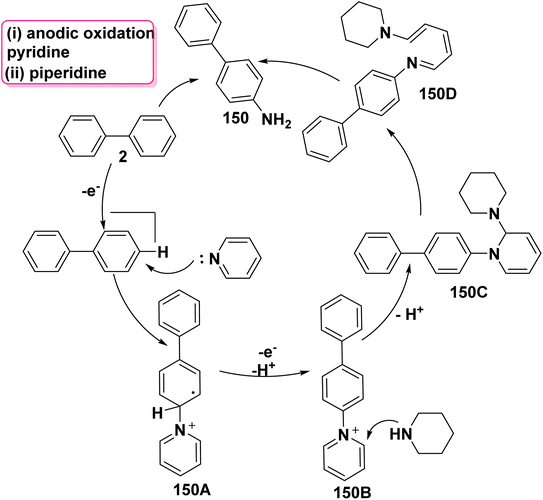 |
| Scheme 61 The possible mechanism for aminobiphenyl synthesis. | |
On the other hand, the proposed mechanism for the formation of aminobiphenyl 150 involves photoinduced electron transfer (PET) of biphenyl 2 in the presence of acridinium salt as a catalyst to an excited state photoredox radical (cat*) and biphenyl cation radical 150A, which reacted with amine derivatives to yield distonic cation radical 150B. Then, the deprotonation reaction of 150B furnished the radical intermediate 150C, which led to production of aminobiphenyl 150 by oxidative aromatization (Scheme 62).160
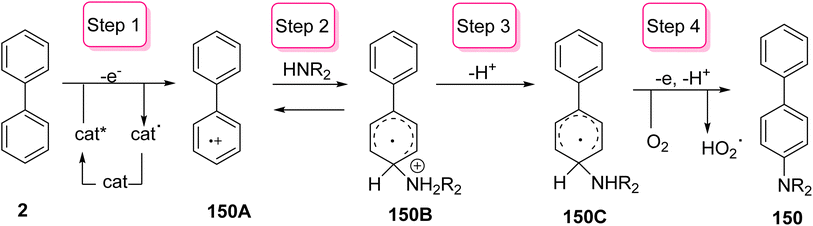 |
| Scheme 62 A postulated mechanism for the formation of aminobiphenyl. | |
3.15 Reaction of bromo-biphenyl with boronic acid
Kashid and coworkers161 reported the synthesis of biphenyl-4-yl-naphthalene 153 via the treatment of bromo-biphenyl 2 with naphthalen-2-yl-boronic acid (152) in the presence of tetrabutylammonium bromide (TBABr), K3PO4, Pd(OAc)2 and Ni complex in a mixture of dioxane and water (Scheme 63).
 |
| Scheme 63 Synthesis of biphenyl naphthalene derivative. | |
3.16 Reaction of biphenyl with bis(pinacol)diborane
The borylation reaction of biphenyl (2) with B2pin2 74 in the presence of potassium t-butoxide (t-BuOK) and (1,5-cyclooctadiene) (methoxy)iridium(I) dimer ([Ir(OMe)COD]2) catalyst in THF with dimethylbipyridyl (dmbpy) afforded tetrakis(Bpin)biphenyl 154.162 On the other hand, refluxing of biphenyl 2 with B2pin2 74 and a catalytic amount of N-heterocyclic carbene platinum(0) complex (IPr*Pt(dvtms)) gave two different oriented products namely, meta-(Bpin)biphenyl (155) and para-(Bpin)biphenyl (156) (Scheme 64).163
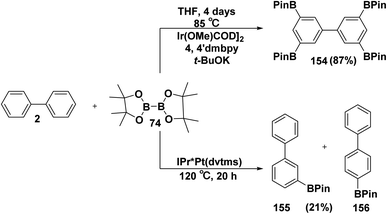 |
| Scheme 64 Borylation of biphenyl. | |
3.17 MCRs for the synthesis of biphenyl amide derivative
Multicomponent reaction (MCRs) of biphenyl 2, 2-aminobenzoimidazole (157), and dihydro-2H-pyran-2,6-dione in the presence of 4-amino glucose functionalized silica propyl coated nickel ferrite nanoparticle (NiFe2O4@SiO2-propyl@glucosamine) catalyst and H2O were stirred at room temperature for 1 h afforded biphenyl amide derivative 158 (Scheme 65).164
 |
| Scheme 65 MCRs for the synthesis of biphenyl amide derivative. | |
3.18 Cyanation reaction of biphenyl
One-pot arene C–H cyanation reaction of biphenyl 2, phenoxathiine-10-oxide and tert-butyl isocyanide mediated by photoactive electron acceptor donor complex (EDA) such as tris(4-bromophenyl)amine in the presence of Tf2O, DCE and basic additive (NaOAc) under blue-light irradiation produced biphenyl carbonitrile 159 (Scheme 66).165 Whereby, phenylbenzonitrile derivatives 159 and 16 were obtained through the reaction of biphenyl (2) with trimethylsilyl cyanide (TMSCN) in the presence of acridinium photocatalyst and phosphate buffer in O2-sparged CH3CN.166
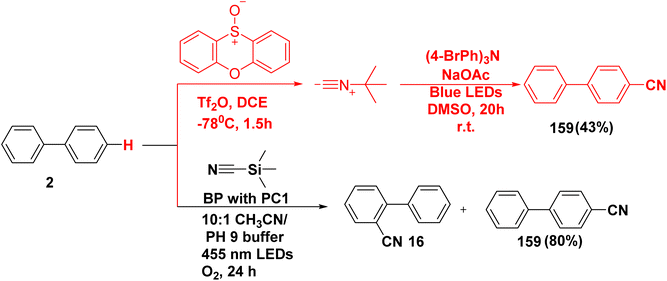 |
| Scheme 66 Construction of biphenyl nitrile derivative. | |
According to the proposed mechanism supporting the photochemical arene C–H functionalization method. Sulfonium salt 159A is produced by site-selective addition of heteroaromatic sulfoxides 160 to unfunctionalized biphenyl 2 via interrupted Pummerer reactivity. The sulfonium salt 159A label's electron-deficient heteroaromatic system can form photoactive electron donor–acceptor (EDA) complexes with triaryl amine donors. Blue-light irradiation of the EDA complex causes single-electron transfer (SET) from the amine donor to salt 159A, which forms the radical cation of the electron donor and stimulates the synthesis of the aryl radical 159B. The radical trap 159B intercepting the aryl radical intermediate produces open shell species 159C, which are prone to oxidation to yield cations 159D, then the active electron-donor catalyst is regenerated. Finally, fragmentation of either tert-butyl isocyanide cation from 159D yields the required C–H functionalization products 159 (Scheme 67).165
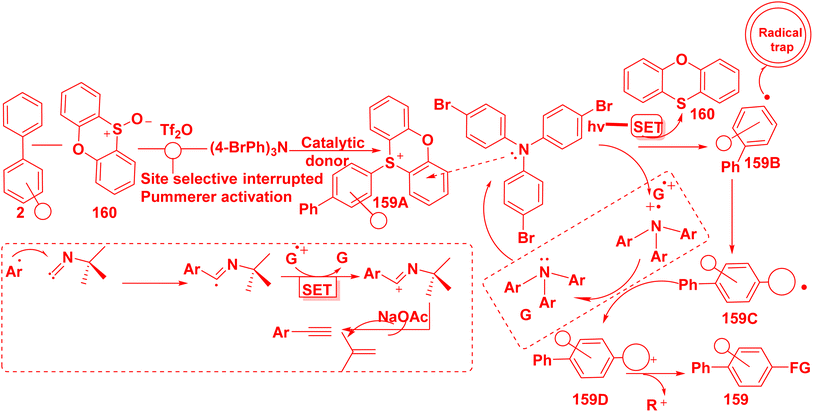 |
| Scheme 67 The postulated mechanism for biphenyl carbonitrile synthesis. | |
The proposed mechanism for phenylbenzonitrile synthesis 159 starts with photo irradiation of acridinium catalyst to its oxidizing excited state Mes-Ac+*, followed by oxidation of biphenyl 2 to its radical cation 2A to produce the reduced acridinium Mes-Ac radical. Then, cyanide engages with 2A at ortho or para position. According to Fukuzumi cyclohexadienyl radical (2B) undergoes oxidation using molecular oxygen to give phenylbenzonitrile 159. Finally, molecular oxygen or hydroperoxy radical oxidizes Mes-Ac radical to reproduce the catalyst (Scheme 68).166
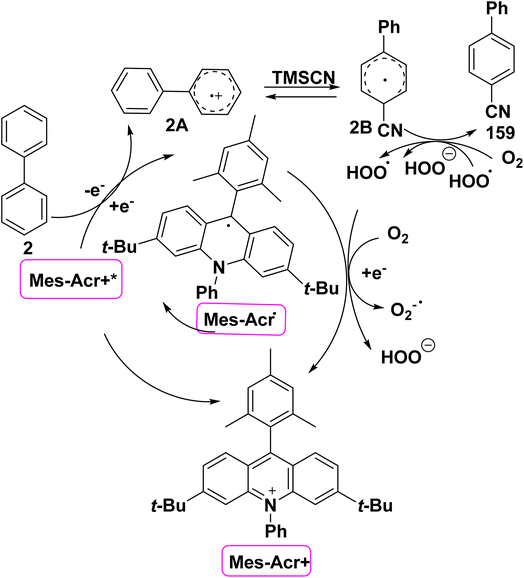 |
| Scheme 68 The suggested mechanism for the synthesis of cyano biphenyl. | |
3.19 Reaction of biphenyl with sulfonium salt
Several methods reported for the introduction of SCF3 group into biphenyl derivative, for example stirring of sodium trifluoromethanesulfinate (CF3SO2Na) with biphenyl derivative 2 in trifluoromethanesulfonic anhydride (Tf2O) and DCM at ambient conditions furnished [1,1′-biphenyl]-4-yl(trifluoromethyl)sulfane derivatives 161 in range yields (65–82%) (Scheme 69).167
 |
| Scheme 69 Trifluoromethylthiolation of biphenyl derivatives 161. | |
The proposed mechanism for the synthesis of [1,1′-biphenyl]-4-yl(trifluoromethyl)sulfane 161 starts with the promotion of trifluoromethylthiolation using Tf2O and CF3SO2Na to afford an intermediate sulfonate sulfinate anhydride through self-disproportionation. Two possible routes lead to the final product. In path I, half of sulfonate sulfinate anhydride intermediate was oxidized to form TfO-SO2CF3 and another part is reduced to form CF3SOSO2CF3. Finally, the transfer of trifluoromethylthiolation from CF3SOSO2CF3 to biphenyl (2) to afford SCF3 product 161 (Scheme 70).167
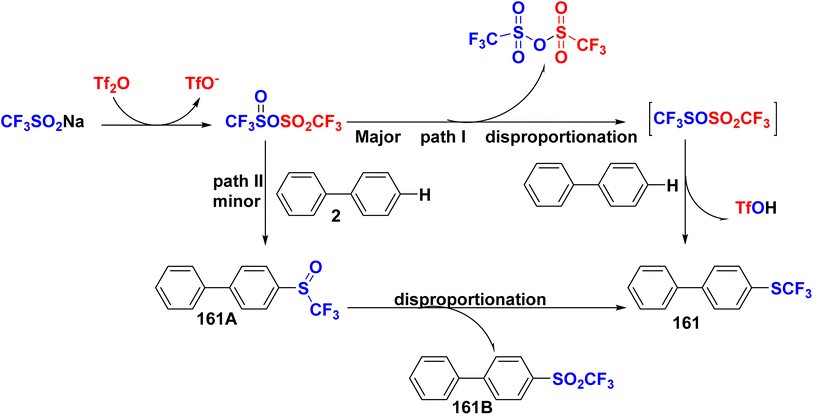 |
| Scheme 70 Mechanism pathway for biphenyl SCF3 synthesis. | |
3.20 Reaction of biphenyl with azole
N-Aryl azoles are a key motif in a number of biologically active compounds used in medicinal chemistry and crop protection science. The reaction of biphenyl (2) with parent pyrazole 162 in a mixture of HFIP and DCM,168 or in the presence of dipotassium peroxodisulfate (K2S2O8) and CH3CN under O2 atmosphere produced 4′-(1H-pyrazol-1-yl)-[1,1′-biphenyl]-4-ylium (163).169 Whereby, Sen et al.170 reported oxidative azolation of unactivated biphenyl derivatives via using phenalenyl-based organic Lewis acid as powerful photooxidant in DCE to afford compound 163. While, under blue light irradiation, the photocatalytic oxidation of biphenyl 2 with azole 162 using DDQ catalyst and tert-butyl nitrite (TBN) yielded 1H-pyrazol-biphenyl-4-ylium 163 and 1-([1,1′-biphenyl]-2-yl)-1H-pyrazole (164) (Scheme 71).171
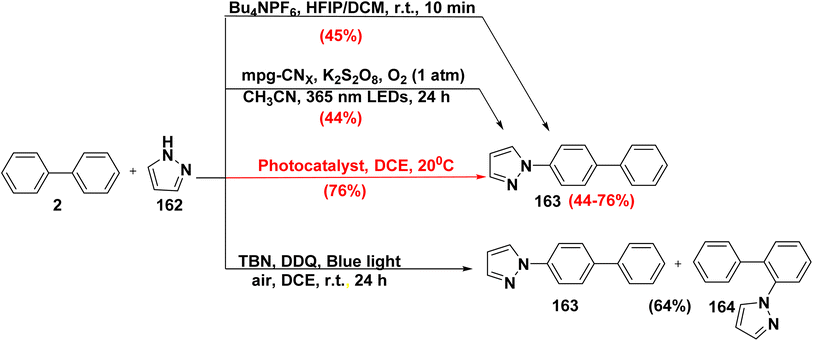 |
| Scheme 71 Synthesis of biphenyl pyrazole derivative. | |
3.21 Reaction of related biphenyl
The anti-tyrosinase properties of biphenyl ester makes it effective for treating dermatological conditions and for cosmetic lightening. A series of [1,1′-biphenyl]-4-yl-oxoethylbenzoate derivatives 166 were produced through two stages. First step involving a mixture of NBS, and 1-([1,1′-biphenyl]-4-yl)ethan-1-one (93) was refluxed in a mixture of petroleum ether (PET) and methanol for 2 h afforded 1-([1,1′-biphenyl]-4-yl)-2-bromoethan-1-one (165). In the second stage, compound 165 was reacted with different phenyl carboxylic acid derivatives in the presence of K2CO3 in DMF at room temperature led to formation of 2-([1,1′-biphenyl]-4-yl)-2-oxoethyl benzoate derivatives 166a. Biphenyl-4-yl-oxoethyl-pyridine carboxylate derivatives 166b were obtained by stirring of 1-([1,1′-biphenyl]-4-yl)-2-bromoethan-1-one (165) with pyridine carboxylic acid derivatives in DMF containing anhydrous K2CO3 (Scheme 72).10
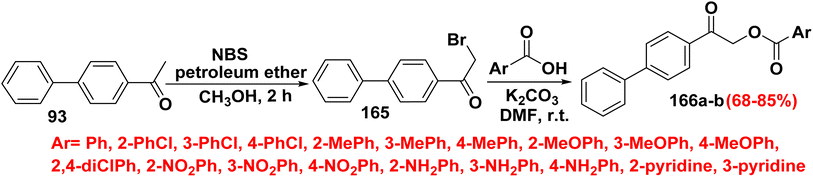 |
| Scheme 72 Formation of biphenyl ester. | |
Compounds containing biphenyl underwent electrophilic substitution to aromatic substrates. Dihydro-6H-dibenzo[1,5]dioxonine 168 was produced by refluxing biphenyl-diol 167 with dibromopropane in acetone containing catalytic amount of anhydrous K2CO3. Whereas, under the same condition, refluxing of biphenyl-2,2′-diol (167) with dibromomethane afforded dibenzo[1,3]dioxepine 169. Furthermore, treatment of biphenyl-2,2′-diol (167) with 1,3-dibromopropane and K2CO3 in EtOH gave a mixture of 2′-(3-bromopropoxy)-[biphenyl]-2-ol 170a and bis(3-bromopropoxy)biphenyl 170b. In the same manner, a mixture of biphenyl-diol derivative 167 and methyl carbonochloridate in EtOH afforded biphenyl derivative 171. It is worthy to mention that o-alkylated biphenyl derivatives exhibit excellent scavenging of superoxide anion radical and β-glucuronidase inhibition activity (Scheme 73).12
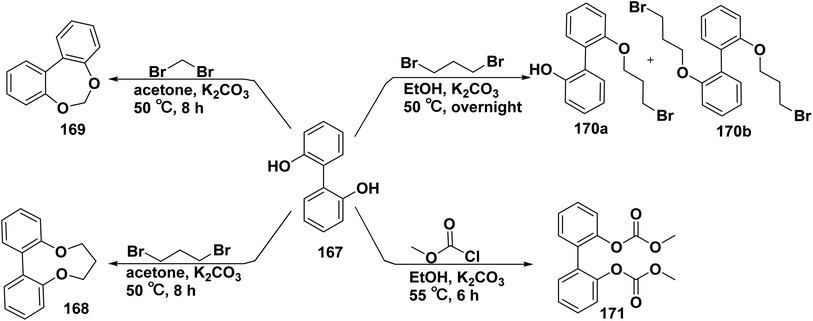 |
| Scheme 73 Reaction of biphenyl diol with various halo-derivatives. | |
According to Suzuki–Miyaura and Hantazch reactions, treatment of 4′-formylbiphenyl (172) with active methylene namely acetone derivatives or substituted pyrazole derivatives and ammonium hydroxide (NH4OH) gave tricyclic ring of 4-biphenyl-1,4-dihydropyridine derivatives 173 and pentacyclic ring of [4-biphenyl]-1,7-dihydrodipyrazolo[3,4-b]pyridine derivatives 175, respectively. Then, oxidation of 173 and 175 with nitric acid (HNO3) afforded 4-(biphenyl-4-yl)-pyridine derivatives 174 and 4-biphenyl-dipyrazolo[3,4-b]pyridine derivatives 176, respectively which are used as anti-microbial resistance, antibacterial, antifungal and anticancer (Scheme 74).172
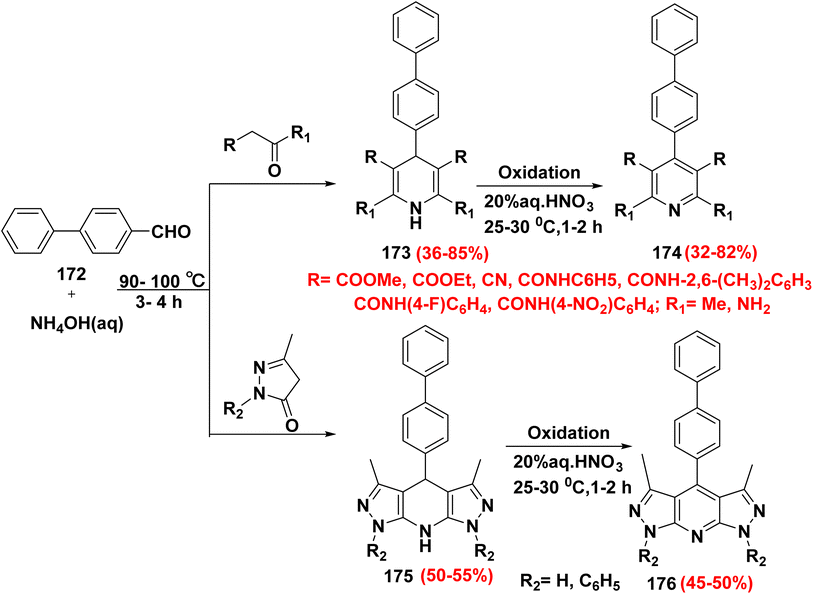 |
| Scheme 74 Suzuki–Miyaura and Hantazch reaction for preparation of annulated pyridine systems. | |
Reduction of biphenyl dicarboxylic acid 177 with lithium aluminum hydride (LiAlH4) afforded biphenyl diol 178, followed by bromination reaction with phosphorus tribromide (PBr3) produced biphenyl dibromide 179. Then, compound 179 underwent cyclization reaction to produce azepine derivative 180. Finally, dealkylation of compound 180 furnished biphenyl diazepine derivative 181 (Scheme 75).173
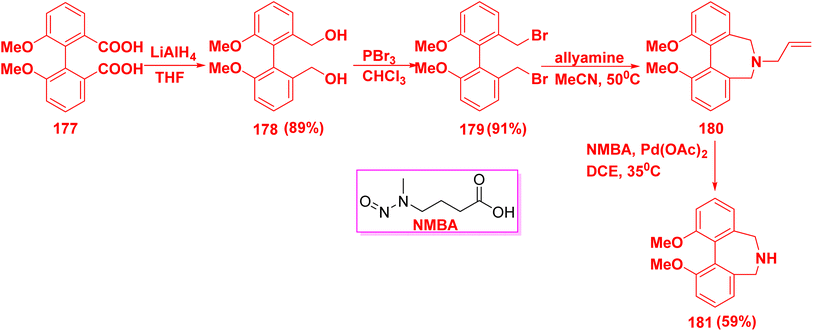 |
| Scheme 75 Synthesis of biphenyl diazepine derivative 181. | |
4. Biphenyl atropisomerism
As reflected in the last few years, the crest of a sizable wave in the development of new ligands in drug discovery and medicinal chemistry, the recent literature addressing atropisomerism has been hard to miss, as biphenyl atropisomers have received considerable attention as chiral building blocks for chiral catalysts in asymmetric syntheses field. The first experimentally described molecule with atropisomerism phenomenon was 6,6′-dinitro-2,2′-diphenic acid by Christie and Kenner in 1922.174,175 As the inclusion of the biphenyl isosteres represent 4.3% of all known drugs.176 Atropisomerism is defined as stereochemistry arising from highly hindered bond rotation that creates a chiral axis. The rotational barrier of an atropisomeric molecule is sufficiently high to allow for isolation of the individual conformers. Whereby, atropisomeric pharmaceutical ingredients can differ in their pharmacokinetic and selectivity properties towards a hitting target (Fig. 3).177–183
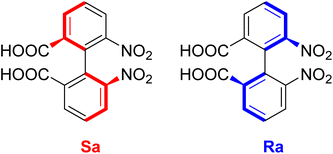 |
| Fig. 3 Atropisomerism of biphenyl compound 2. | |
5. Applications
Fenbufen is a non-steroidal anti-inflammatory, antipyretic effects (NSAIDs) and analgesic drug with less irritative effect on gastrointestinal tract (Table 1). The synthesis of fenbufen (183) was performed in two steps. First, Friedel–Crafts acylation reaction of bromobenzene (1) and succinic anhydride catalyzed by AlCl3 afforded 1-(4-bromophenyl)-4-hydroperoxybutan-1-one (182), then coupling the product 182 with phenyl boronic acid (7) (Scheme 76).15
Table 1 Examples of a vast array of biologically active molecules towards some diseases
Structure |
Activities |
Action mechanism & references |
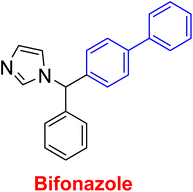 |
Antibacterial: (Staphylococcus aureus and Salmonella spp.) |
The inducing of chlorine atoms on the biphenyl ring connected to the imidazole ring led to alterations in the physico-chemical properties, therefore these factors can affect the affinity of molecules for the iron of the heme binding site.184 Addition of biphenyl moiety improves its antifungal selectivity, potency and bioisosteric effects185 |
Therapy for dermatomycoses |
Antifungal: (Candida albicans, Cryptococcus neoformans and Aspergillus fumigatus)184,185 |
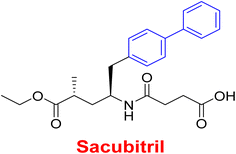 |
Anti-tubercular: (dormant tuberculosis) |
Due to the presence of substituted biphenyl increases the polarity of the heterocyclic skeleton which might be the reason for high anti-tubercular (anti-TB) activities via interacting with the MurB inhibitors186 |
Treatment of heart failure with a combination of valsartan |
Neprilysin inhibitor186,187 |
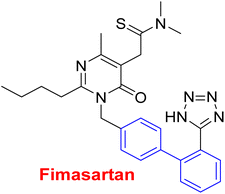 |
Antihypertensive |
Since inhibition of angiotensin II type 1 (AT1) receptor reduces chronic inflammation associated with hypertension, we evaluated the anti-inflammatory potential and the underlying mechanism of fimasartan188 |
Anti-inflammatory188 |
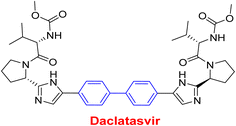 |
Antiviral |
As a result of sp2 hybridization of CNTs between the drug and target protein sequence, leads to improving the fluorescence reactivity. Because the conjugated system of biphenyl and the presence of Cs/CNT can increase the electroactive surface area of the electrode, leading to an increase in the number of structural flaws189 |
Treatment selections for hepatitis C virus189,190 |
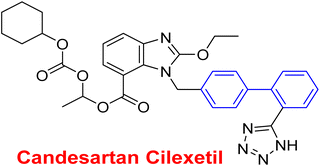 |
Antihypertensive |
Biphenyl acts as lipophilic moiety in candesartan drug; whereas the candesartan is a selective nonpeptide angiotensin II type 1 (AT1) receptor antagonist which reduces blood pressure effectively.191 Sartans incorporates with membrane receptor in lipid bilayers causing highly transportation possibility of sartans via the receptor192 |
Active for the AT1 receptor191–193 |
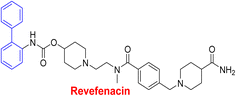 |
Lung-selective muscarinic cholinergic receptor (mAChR) antagonist |
Biphenyl moiety enhances long-lasting and potency of mediated antagonism of mAChR-causing contraction of human bronchial tissues194 |
Nebulized inhalation solution to produce long-acting bronchodilation194 |
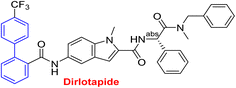 |
Microsomal triglyceride transfer protein inhibitor |
Biphenyl scaffold for nesting |
Treatment for human dyslipidemias195 |
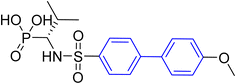 |
Anti-human neutrophil collagenase (MMP-8)196 |
Biphenyl residues hit the active site close the catalytic zinc ion that would consequently inhibit the collagenase activity196 |
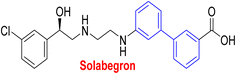 |
β3-Adrenergic receptor agonist |
RHS of biphenyl ring affords potent human β3-AR agonists with a chlorophenyl ring on the LHS side197 |
Evokes bladder relaxation |
Overactive urinary bladder |
Increases micturition reflex threshold in the dogs197–199 |
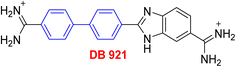 |
Analysis of water mediated binding in the context of a DNA complex |
The interactions of the molecules containing of biphenyl with DNA AT sites increasing DNase I footprinting depending on increasing conjugation process which enhancing biosensor-surface plasmon resonance, circular dichroism microcalorimetry, and isothermal titration200 |
Promising agent against parasites |
Change in AT sequences with destruction of the kinetoplast and cell death200 |
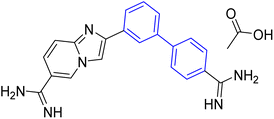 |
Antiprotozoal |
Biphenyl scaffold for nesting |
Anti-trypanosomal201 |
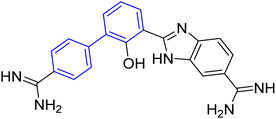 |
Anti-protozoan202 |
Biphenyl scaffold for nesting |
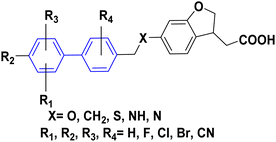 |
Treating diabetes mellitus203 |
Biphenyl scaffold for nesting |
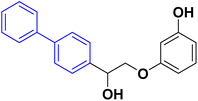 |
Treatment CNs impairments involving major depressive disorder204 |
Biphenyl-based NMDA negative allosteric modulator (NAM) has low affinity for the human ether-a-go-go-related gene ion channel (hERG) and the dynamics calculations suggest a various binding mode (ifenprodil-like) compared to another biaryl-based NMDA NAM EVT-101 204 |
 |
| Scheme 76 Synthetic strategy for the preparation of fenbufen. | |
The trade name for valsartan is Diovan, valsartan belongs to the non-peptide angiotensin (II) receptor class and is used to treat cardiovascular disorders that are connected to hypertension. Synthesis of valsartan (190) began with coupling reaction of L-valine methyl ester hydrochloride (184) with valeryl chloride in the presence of triethylamine (Et3N) in DCM to produce methyl N-pentanoyl-L-valinate (185). N-Protection of compound (185) with 1-bromo-4-(bromomethyl) benzene (186) in the presence of sodium hydride (NaH) in THF furnished methyl N-(4-bromobenzyl)-N-pentanoyl-D-valinate (187). Then, organozinc compound was synthesized via o-metalation of 5-phenyl-1-trityl-1H-tetrazole (188) with n-butyllithium (n-BuLi), followed by adding zinc chloride (ZnCl2). Negishi coupling reaction of organozinc chloride compound with aryl bromide 187 in the presence of palladium acetate (Pd(OAc)2) with 1,2,3,4,5-pentaphenyl-1′-(di-tert-butylphosphino)ferrocene (Q-phos) ligand in THF afforded N-pentanoyl-N-(2′-(1-trityl-1H-tetrazol-5-yl)-[1,1′-biphenyl]-4-yl)-valinate (189). Finally, valsartan 190 was produced via hydrolysis of ester 189 with sodium hydroxide (Scheme 77).205
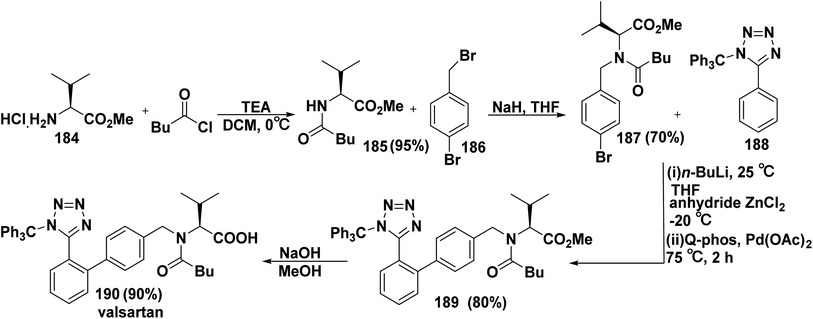 |
| Scheme 77 Negishi coupling reaction for valsartan synthesis. | |
The synthesis of 2-fluoro-[1,1′-biphenyl]-4-yl-propanoic acid which trade name mark (flurbiprofen) 197 has been achieved in subsequent steps. First step involving the reaction of 2,4-difluoronitrobenzene (191) with diethyl 2-methylmalonate (192) in the presence of NaH to afford diethyl 2-(3-fluoro-4-nitrophenyl)-2-methylmalonate (193), followed by catalytic hydrogenation of compound 193 to 4-amino-3-fluorophenyl-2-methylmalonate 194. After that, diazotization of the amino compound 194 into corresponding diazonium salt which then reacted with potassium iodide (KI) to produce diethyl 2-(3-fluoro-4-iodophenyl)-2-methylmalonate (195). Compound 195 underwent hydrolysis and decarboxylation reaction to yield 2-(3-fluoro-4-iodophenyl)propanoic acid (196). Finally, SMC reaction of 3-fluoro-4-iodophenylpropanoic acid 196 with phenylboronic acid in the presence of a catalytic amount of Pd(PPh3)4, 1,2-dimethoxyethane and Na2CO3 produced flurbiprofen (197). Flurbiprofen, a prostaglandin biosynthesis inhibitor, is extensively used as potent anti-inflammatory, antifungal, antipyretic, analgesic, in addition to, treatment Alzheimer's disease (AD) (Scheme 78).206,207
 |
| Scheme 78 Synthesis of flurbiprofen. Reagents and conditions: (i) NaH, DMSO; (ii) H2, Pd/C; (iii) 1. NaNO2, HCl, 0 °C; 2. KI; (iv) NaOH, EtOH–H2O, 100 °C, 57% (four steps); (v) PhB(OH)2, Pd(PPh3)4, Na2CO3, DME-H2O, 80 °C. | |
Sonidegib which is marketed under Odomzo trade name is a Hedgehog pathway inhibitor. Sonidegib is effective against skin cancer, tumor, and medulloblastoma. Synthesis of sonidegib 201 started with the coupling of dimethylmorpholinopyridin-3-amine 198 with 3-bromo-2-methylbenzoic acid (199) in the presence of coupling agent such as dicyclohexylcarbodiimide (DCC) and 4-(dimethylamino)pyridine (DMAP) and TPGS-750-M-H2O as a surfactant to afford 3-bromo-(2,6-dimethylmorpholino)pyridin-3-yl)-2-methylbenzamide 200. Then, SMC reaction of compound 200 with (4-(trifluoromethoxy)phenyl)boronic acid (7) in the presence of Pd(OAc)2, PPh3, Pd, K3PO4 and THF (Scheme 79).208
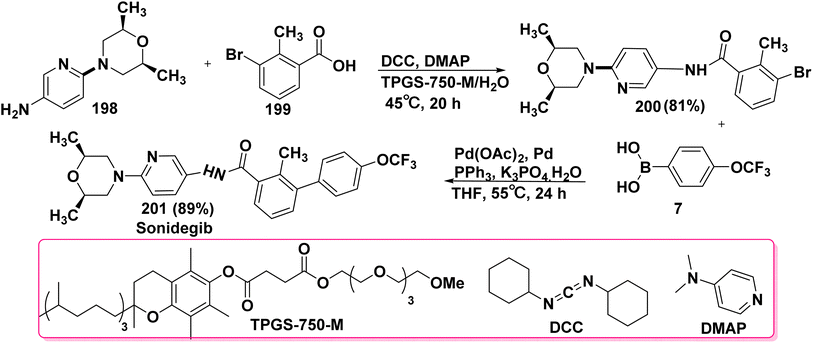 |
| Scheme 79 SMC reaction for biphenyl synthesis. | |
One-pot three component synthesis of 4-(2,4-difluorophenyl)anisole (205) through stirring a mixture of anisole (202), isoamyl nitrite (203), and 2,4-difluoroaniline (204) in the presence of cupper(i)salt. Demethylation reaction of difluorophenylanisole (205) with hydrogen iodide (HI) afforded 4-(2,4-difluorophenyl)-phenol (206). Kolbe–Schmitt phenol carboxylation of 206 with carbon dioxide (CO2) in the presence of K2CO3 afforded diflunisal (207). Whereby, diflunisal (207) has anti-inflammatory, anti-fever, and analgesic properties. In cases of osteoarthritis and rheumatoid arthritis, it is utilized to relief pain (Scheme 80).209,210
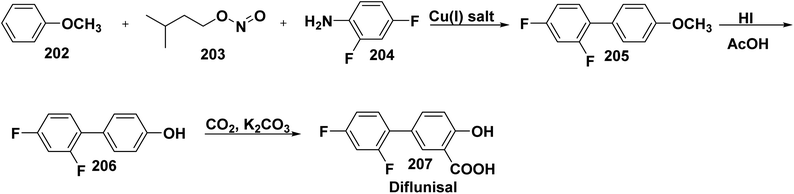 |
| Scheme 80 MCRs for the synthesis of diflunisal. | |
Reaction of glycine methylester 208 with pentanoyl chloride in TEA produced methyl pentanoylglycinate (209). Compound 209 reacted with biphenylcarbonitrile derivative 16 in the presence of KOt-Bu afforded biphenylamide derivative 210. Then, cyclization reaction of compound 210 in the presence of TFA afforded imidazobiphenyl carbonitrile derivative 211. Alkylation reaction of compound 211 with dihaloalkane furnished spirocyclic compound 212.211 Finally, irbesartan (213) was synthesized via reaction of key intermediate 212 with sodium azide, tributyltin chloride (TBTC) and dimethoxycyclohexadiene 213. In pancreatic cancer, irbesartan overcomes gemcitabine resistance by decreasing iron metabolism and stemness via inhibition of the Hippo/YAP1/c-Jun axis.212 In addition, irbesartan lowers cholesterol via inhibiting the expression of the low-density lipoprotein receptor. Furthermore, irbesartan is used for the treatment of hypertension and type 2 diabetes (Scheme 81).213
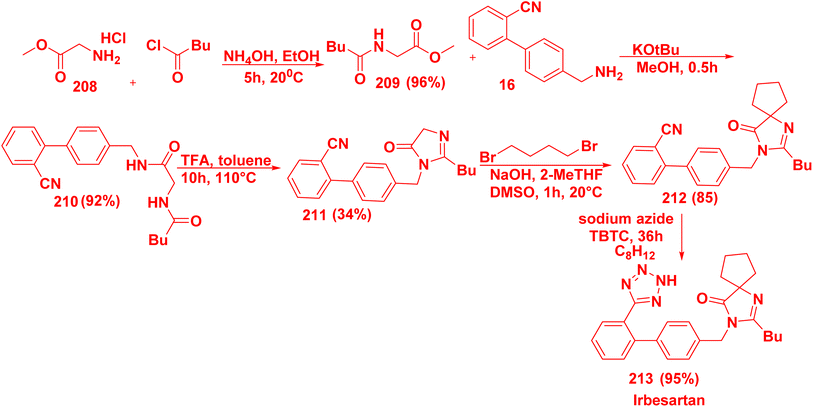 |
| Scheme 81 Synthetic strategies for irbesartan synthesis. | |
6. Patents
Biphenyl sulfothiadiazole derivatives (I) are used in clinical immunotherapy of tumour patients and act as a PD1/PDL1 inhibitor.214 Whereas, biphenyl biaryl pyrimidine derivatives (II) are utilized to treat AIDS and are expected to be anti-HIV drugs.215 5′-((2-((3,4-Dimethoxyphenethyl)amino)-2-oxoethyl)amino)-2′-hydroxy-[1,1′:3′,1′′-biphenyl]-3,3′′-dicarboxylic acid (III) is developed to treat malignant tumours and as a transcription factor.216 3-(2-([1,1′-Biphenyl]-4-yl)-2-hydroxyethoxy) phenol (IV) is a therapy for a disorder or disease accompanied by high activation of the NMDA receptor.217 4-(1-Substituted phenylvinyl)biphenyl derivatives (V) can be used for the manufacture of protein kinase, tubulin inhibitors and drugs for colon and breast cancer.218 2-[(3,5-Difluoro-3′-methoxy-1,1′-biphenyl-4-yl)amino]nicotinic acid is used to treat psoriasis disease.219 (2E)-[2-(Biphenyl)imidazo[1,2-a]pyrimidine-3-yl](4-nitrophenyl)-prop-2-en-1-one, 2-(biphenyl)imidazo[1,2-a]pyrimidine-3-carbaldehyde and 6-[2-(biphenyl)imidazo[1,2-a]pyrimidine-3-yl]-4-nitro pyrimidine-2(1H)-one exhibit promising antitumor activity by reducing the level of liver function enzyme near the normal level after damaged by CCl4.220 Whereby, trimethoxy-[1,1′-biphenyl]-2-ol (VI) displays anti-inflammatory activity221 (Fig. 4).
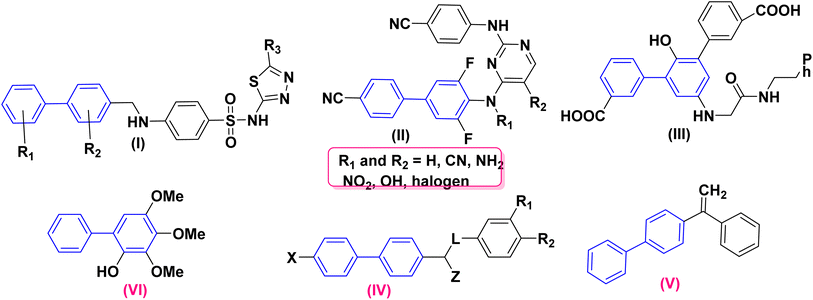 |
| Fig. 4 Some biologically active biphenyl derivatives. | |
7. Conclusion
With the continuously increasing importance of biphenyl scaffolds as the structurally decisive scaffolds in bioactive natural products and pharmaceuticals drugs, formidable efforts have been carried out to summarize gradual developments of biphenyl compounds and their isosteres via metallic catalyzed reaction which covered the literature reported in the last decades based on this thrilling area of research. In this review several chemical reactions of biphenyl with different reagents have been discussed. The profound discussion of different captivating mechanisms associated with the role of numerous key catalysts and reagents through this review is believed to be beneficial for the synthetic community to apply these methods for practical purposes. Additionally, the efficacy of biphenyl compounds in medicinal chemistry and academic industry has been covered, and thereby will be helpful for future research. Although, obtaining these frameworks with particular substitution patterns is still an intimidating exercise. Atropisomerization phenomena of biphenyl derivatives also has taken into account Therefore, we believe that this review can be a guiding principle for synthetic and medicinal chemists working in this field.
Conflicts of interest
The authors confirm that this article content has no conflict of interest.
Abbreviations
H2NCO2−H4N+ | Ammonium carbamate |
AD | Alzheimer's disease |
ATRA | All-trans retinoic acid |
NiFe2O4@SiO2-propyl@glucosamine | 4-Amino glucose functionalized silica propyl coated nickel ferrite nanoparticle |
Ae | Alkali metals earth |
Pd(dba)2 | Bis(dibenzylideneacetone)palladium |
PdCl2(PPh3)2 | Bis-triphenylphosphine palladium dichloride |
B2pin2 | Bis(pinacolato)diboron |
IMes | 1,3-Bis(2,4,6-trimethylphenyl)-1,3-dihydro-2-H-imidazol-2-ylidene |
(2-BrC6H4)OPPh2 | (2-Bromophenyl)diphenylphosphine oxide |
[pd(dppf)Cl2CH2Cl2] | 1,1′-Bis(diphenylphosphino)ferrocene-palladium(II)dichloride dichloromethane complex |
CrCl3 | Chromium(III) chloride |
Cy2PCl | Chlorodicyclohexylphosphane |
CAN | Ceric ammonium nitrate |
[Ir(OMe)COD]2 | (1,5-Cyclooctadiene)(methoxy)iridium(I) dimer |
CoCl2 | Cobalt(II) chloride |
DCM | Dichloromethane |
EDB | 1,2-Dibromoethane |
DCICA | Dichloroiodoisocyanuric acid |
K2S2O8 | Dipotassium peroxodisulfate |
dmbpy | Dimethylbipyridyl |
DCC | Dicyclohexylcarbodiimide |
DMAP | 4-(Dimethylamino)pyridine |
FtsZ | Filamenting temperature-sensitive mutant Z protein |
HTS | High-throughput screening |
HFIP | 1,1,1,3,3,3-Hexafluoroisopropanol |
LCDs | Liquid crystal displays |
LiAlH4 | Lithium aluminium hydride |
MOF | Metal–organic frameworks |
2-MTHF | 2-Methyltetrahydrofuran |
mCPBA | meta-Chloroperbenzoic acid |
MCRs | Multicomponent reaction |
NBS | N-Bromosuccinimide |
NSAIDs | Non-steroidal anti-inflammatory drug |
NMEA | N-Methylethanolamine |
OLEDs | Organic light-emitting diodes |
(COCl)2 | Oxalyl chloride |
OMIMs | Organic polymer of intrinsic microporosity |
ODCB | O-Dichlorobenzene |
Pd/CNS | Palladium-catalyst on porous carbon nanospheres |
Na[Pd-NAS] | Palladium grafted on natural asphalt sulfonate |
PCBs | Polychlorinated biphenyls |
PBBs | Polybrominated biphenyls |
PPA | Polyphosphoric acid |
phen | 1,10-Phenanthroline |
KPF6 | Potassium hexafluorophosphate |
[Cp*RhCl2]2 | Pentamethylcyclopentadienyl rhodium dichloride dimer |
PBr3 | Phosphorus tribromide |
SMC | Suzuki–Miyaura coupling reaction |
CF3SO2Na | Sodium trifluoromethanesulfinate |
AgSbF6 | Silver hexafluoroantimonate (V) |
Ag2O | Silver oxide |
SmBr2 | Samarium dibromide |
SET | Single-electron transfer |
PPh3 | Triphenylphosphine |
TEA | Triethylamine |
(Pd2(dba)3) | Tris(dibenzylideneacetone)dipalladium(0) |
SOCl2 | Thionyl chloride |
Ph3SnCl | Triphenyltin chloride |
tfp | Tris-o-furylphosphine |
SnCl4 | Tin(IV)chloride |
PBu3 | Tributyl phosphine |
CHCl3 | Trichloromethane |
t-Boc | tert-Butyloxycarbonyl |
Pd(PPh3)4 | Tetrakis(triphenylphosphine)palladium |
TMEDA | Tetra methyl ethylenediamine |
TFAA | Trifluoroacetic anhydride |
Bu4NBF4 | Tetrabutylammonium tetrafluoroborate |
TEMPO | 2,2,6,6-Tetramethylpiperidine-1-oxyl |
TBABr | Tetrabutylammonium bromide |
TMSCN | Trimethylsilyl cyanide |
Tf2O | Trifluoromethanesulfonic anhydride |
TBN | tert-Butyl nitrite |
TBTC | Tributyltin chloride |
References
- S. S. Panda and N. K. Sharma, Org. Biomol. Chem., 2023, 21, 1468–1477 RSC.
- F. Leroux, ChemBioChem, 2004, 5, 644–649 CrossRef CAS PubMed.
- G. Bringmann, R. Walter and R. Weirich, Angew. Chem., Int. Ed., 1990, 29, 977–991 CrossRef.
- S. N. S. Vasconcelos, C. S. Barbeiro, A. N. Khan and H. A. Stefani, J. Braz. Chem. Soc., 2015, 26, 765–774 CAS.
- J. Yang, V. Giuso, M. Hou, E. Remadna, J. Forté, H. Su, C. Gourlaouen, M. Mauro and B. Bertrand, Inorg. Chem., 2023, 62, 4903–4921 CrossRef CAS PubMed.
- Z. J. Jain, P. S. Gide and R. S. Kankte, Arabian J. Chem., 2017, 10, S2051–S2066 CrossRef CAS.
- S. Thareja, S. K. Verma, A. K. Jain, M. Kumar and T. R. Bhardwaj, J. Mol. Struct., 2023, 1274, 134546 CrossRef CAS.
- K. M. Orritt, L. Feng, J. F. Newell, J. N. Sutton, S. Grossman, T. Germe, L. R. Abbott, H. L. Jackson, B. K. L. Bury, A. Maxwell, M. J. McPhillie and W. G. Fishwick, RSC Med. Chem., 2022, 13, 831–839 RSC.
- J. Ehlert, J. Konemann, N. Zumbrägel and M. Preller, Molecules, 2019, 24, 4272 CrossRef PubMed.
- H. C. Kwong, C. S. C. Kumar, S. H. Mah, T. S. Chia, C. K. Quah, Z. H. Loh, S. Chandraju and G. K. Lim, PLoS One, 2017, 12, 0170117 Search PubMed.
- V. K. Ol'khovik, Y. V. Matveienko, D. A. Vasilevskii, G. V. Kalechits and R. A. Zheldakova, Russ. J. Gen. Chem., 2013, 83, 329–335 CrossRef.
- M. Irshad, S. B. Jamal, M. Faheem, M. Aslam, S. S. Shafqat and A. Kanwal, Russ. J. Gen. Chem., 2021, 91, 1084–1092 CrossRef CAS.
- A. Rusu, C. Tanase, G. Pascu and N. Todoran, Pharmaceuticals, 2020, 13, 217 CrossRef CAS PubMed.
- M. Migden, A. S. Farberg, R. Dummer, N. Squittieri and C. W. Hanke, J. Drugs Dermatol., 2021, 20, 156–165 CrossRef PubMed.
- J. Wang, T. Li, Z. Zhao, X. Zhang and W. Pang, Catal. Lett., 2022, 152, 1545–1554 CrossRef CAS.
- N. M. Davies, Clin. Pharmacokinet., 1995, 28, 100–114 CrossRef CAS PubMed.
- I. N. Egorov, A. Mukherjee, S. Santra, D. S. Kopchuk, I. S. Kovalev, Y. Liu, G. V. Zyryanov, A. Majee, O. N. Chupakhin and B. C. Ranu, Adv. Synth. Catal., 2022, 364, 2462–2478 CrossRef CAS.
- K. Tamao, K. Sumitani and M. Kumada, J. Am. Chem. Soc., 1972, 94, 4374–4376 CrossRef CAS.
- R. J. P. Corriu and J. P. Masse, J. Chem. Soc., Chem. Commun., 1972, 144a RSC.
- W. Guo and Z. Wang, J. Org. Chem., 2013, 78, 1054–1061 CrossRef CAS PubMed.
- A. R. Hajipour and F. Rafiee, Appl. Organomet. Chem., 2013, 27, 412–418 CrossRef CAS.
- F. Foubelo, C. Nájera and M. Yus, Chem. Rec., 2016, 16, 2521–2533 CrossRef CAS PubMed.
- E. Negishi, A. O. King and N. Okukado, J. Org. Chem., 1977, 42, 1821–1823 CrossRef CAS.
- A. Olding, C. C. Ho, D. Maiti and A. C. Bissember, Chem. Commun., 2023, 59, 5144–5155 RSC.
- M. M. Heravi, E. Hashemi and N. Nazari, Mol. Diversity, 2014, 18, 441–472 CrossRef CAS PubMed.
- J. K. Stille, Angew. Chem., Int. Ed., 1986, 25, 508–524 CrossRef.
- A. S. Levashov, D. S. Buryi, O. V. Goncharova, V. V. Konshin, V. V. Dotsenko and A. A. Andreev, New J. Chem., 2017, 41, 2910–2918 RSC.
- P. S. Gahlaut, D. Gautam, P. Lama and B. Jana, New J. Chem., 2023, 47, 6871–6879 RSC.
- N. Miyaura, K. Yamada and A. Suzuki, Tetrahedron Lett., 1979, 20, 3437–3440 CrossRef.
- Z. Wang, Wurtz-Fittig Reaction, Comprehensive Organic Name Reactions and Reagents, John Wiley & Sons, 2010, vol. 686, pp. 3100–3104, ISBN 9780470638859 Search PubMed.
- R. B. Richards, Trans. Faraday Soc., 1940, 36, 956–960 RSC.
- Q. Sun, L. Cai, Y. Ding, Y. H. Ma, C. Yuan and W. Xu, Phys. Chem. Chem. Phys., 2016, 18, 2730–2735 RSC.
- S. Asako, I. Takahashi, H. Nakajima, L. Ilies and K. Takai, Commun. Chem., 2021, 4, 76 CrossRef CAS PubMed.
- J. M. Tour, Adv. Mater., 1994, 6, 190–198 CrossRef CAS.
- F. Ullmann and J. Bielecki, Ber. Dtsch. Chem. Ges., 1901, 34, 2174–2185 CrossRef CAS.
- Y. Zhang, Y. Wang, C. Gao, Z. Ni, X. Zhang, W. Hu and H. Dong, Chem. Soc. Rev., 2023, 52, 1331–1381 RSC.
- J. Lindley and T. J. Mason, Chem. Soc. Rev., 1987, 16, 275–311 RSC.
- A. I. Meyers, T. D. Nelson, H. Moorlag, D. J. Rawson and A. Meier, Tetrahedron, 2004, 60, 4459–4473 CrossRef CAS.
- S. Perveen, S. Zhang, L. Wang, P. Song, Y. Ouyang, J. Jiao, X. Duan and P. Li, Angew. Chem., Int. Ed., 2022, 134, 1–8 CrossRef.
- G. M. Bennett and E. E. Turner, J. Chem. Soc., Trans., 1914, 105, 1057–1062 RSC.
- H. Gilman and M. Lichtenwalter, J. Am. Chem. Soc., 1939, 61, 957–959 CrossRef CAS.
- J. Krizewsky and E. E. Turner, J. Chem. Soc., Trans., 1919, 115, 559–561 RSC.
- C. C. J. Seechurn, M. O. Kitching, T. J. Colacot and V. Snieckus, Angew. Chem., Int. Ed., 2012, 51, 5062–5085 CrossRef PubMed.
- H. Do and O. Daugulis, J. Am. Chem. Soc., 2009, 131, 17052–17053 CrossRef CAS PubMed.
- A. S. Demir, Ö. Reis and M. Emrullahoglu, J. Org. Chem., 2003, 68, 10130–10134 CrossRef CAS PubMed.
- N. Kirai and Y. Yamamoto, Eur. J. Org. Chem., 2009, 1864–1867 CrossRef CAS.
- K. K. Manar and P. Ren, Recent progress on group 10 metal complexes of pincer ligands: from synthesis to activities and catalysis, Elsevier Inc., 1st edn, 2021, vol. 76 Search PubMed.
- G. Manolikakes, Coupling Reactions Between sp3 and sp2 Carbon Centers, Elsevier Ltd, 2014, vol. 3 Search PubMed.
- S. Zhang, X. Li, H. Sun, O. Fuhr and D. Fenske, J. Organomet. Chem., 2016, 820, 41–45 CrossRef CAS.
- P. Walla and C. O. Kappe, Chem. Commun., 2004, 4, 564–565 RSC.
- L. Zhang, G. Y. Ang and S. Chiba, Org. Lett., 2010, 12, 3682–3685 CrossRef CAS PubMed.
- S. E. Allen, R. R. Walvoord, R. Padilla-Salinas and M. C. Kozlowski, Chem. Rev., 2013, 113, 6234–6458 CrossRef CAS PubMed.
- E. Hagiwara, K. I. Gouda, Y. Hatanaka and T. Hiyama, Tetrahedron Lett., 1997, 38, 439–442 CrossRef CAS.
- H. M. Lee and S. P. Nolan, Org. Lett., 2000, 2, 2053–2055 CrossRef CAS PubMed.
- J. H. Li, C. L. Deng, W. J. Liu and Y. X. Xie, Synthesis, 2005, 3039–3044 CrossRef CAS.
- X. Zhang, F. Zhang, X. Li, M. Lu, X. Meng, L. Huang and H. Luo, Org. Lett., 2022, 24, 5029–5033 CrossRef CAS PubMed.
- R. Noor, A. F. Zahoor, M. Irfan, S. M. Hussain, S. Ahmad, A. Irfan, K. Kotwica-Mojzych and M. Mojzych, Molecules, 2022, 27, 5654 CrossRef CAS PubMed.
- A. Monfared, R. Mohammadi, S. Ahmadi, M. Nikpassand and A. Hosseinian, RSC Adv., 2019, 9, 3185–3202 RSC.
- T. Yanase, Y. Monguchi and H. Sajiki, RSC Adv., 2012, 2, 590–594 RSC.
- A. Adenot, L. Anthore-Dalion, E. Nicolas, J. Berthet, P. Thuéry and T. Cantat, Chem.–Eur. J., 2021, 27, 18047–18053 CrossRef CAS PubMed.
- S. E. Hooshmand, B. Heidari, R. Sedghi and R. S. Varma, Green Chem., 2019, 21, 381–405 RSC.
- N. Biteau, V. Hervin, V. Roy and L. A. Agrofoglio, Suzuki-Miyaura Cross-Coupling as a Synthetic Tool for Nucleoside and Nucleotide Modification, in Palladium-Catalyzed Modification of Nucleosides, Nucleotides and Oligonucleotides, Elsevier, 2018, pp. 37–74, ISBN: 9780128112922 Search PubMed.
- S. Supriya, G. S. Ananthnag, V. S. Shetti, B. M. Nagaraja and G. Hegde, Appl. Organomet. Chem., 2020, 34, e5384 CrossRef CAS.
- A. J. J. Lennox and G. C. Lloyd-Jones, Angew. Chem., Int. Ed., 2013, 52, 7362–7370 CrossRef CAS PubMed.
- C. Amatore, G. L. Duc and A. Jutand, Chem.–Eur. J., 2013, 19, 10082–10093 CrossRef CAS PubMed.
- B. P. Carrow and J. F. Hartwig, J. Am. Chem. Soc., 2011, 133, 2116–2119 CrossRef CAS PubMed.
- C. Baillie, W. Chen and J. Xiao, Tetrahedron Lett., 2001, 42, 9085–9088 CrossRef CAS.
- C. Liu, X. Song, Q. Ni and J. Qiu, Arkivoc, 2012, 62–75 Search PubMed.
- T. Jastrząbek, A. Ulikowski and R. Lisiak, ChemRxiv, 2019, preprint, DOI:10.26434/chemrxiv.8850296.v1.
- K. Müller, C. Faeh and F. Diederich, Science, 2007, 317, 1881–1886 CrossRef PubMed.
- D. Bulfield and S. M. Huber, J. Org. Chem., 2017, 82, 13188–13203 CrossRef CAS PubMed.
- J. Almond-Thynne, D. C. Blakemore, D. C. Pryde and A. C. Spivey, Chem. Sci., 2017, 8, 40–62 RSC.
- Y. P. Budiman, A. Friedrich, U. Radius and T. B. Marder, ChemCatChem, 2019, 11, 5387–5396 CrossRef CAS.
- C. Gosmini and M. Gao, Co-catalyzed C–C Coupling Reactions with Csp2 Electrophiles, Top. Organomet. Chem., 2023, 1–35 Search PubMed.
- G. Wu and A. J. V. Wangelin, Nat. Catal., 2018, 1, 377–378 CrossRef CAS.
- S. Asghar, S. B. Tailor, D. Elorriaga and R. B. Bedford, Angew. Chem., Int. Ed., 2017, 56, 16367–16370 CrossRef CAS PubMed.
- Y. Lu, E. Plocher and Q. Hu, Adv. Synth. Catal., 2006, 348, 841–845 CrossRef CAS.
- S. Burmaoglu, E. A. Kazancioglu, M. Z. Kazancioglu, R. Sağlamtaş, G. Yalcin, I. Gulcin and O. Algul, J. Mol. Struct., 2022, 1254, 132358 CrossRef CAS.
- S. Wang, X. Wang, Y. Shao, K. Shao, Y. Wang, X. Guo, H. Dong, W. Zhao, Y. Li and G. Li, Bioorg. Chem., 2021, 116, 105298 CrossRef CAS PubMed.
- L. Hudhud, D. R. Chisholm, A. Whiting, A. Steib, K. Pohóczky, A. Kecskés, E. Szőke and Z. Helyes, Molecules, 2022, 27, 977 CrossRef CAS PubMed.
- H. Peacock, S. T. Henriques, A. H. Benfield, A. G. Elliott, J. Luo, J. Luccarelli, M. Nagano, D. J. Craik and A. D. Hamilton, ChemMedChem, 2020, 15, 1932–1939 CrossRef CAS PubMed.
- W. Zhang, B. Wu and W. Sun, Synthesis, characterization and ethylene reactivity of 2-ester-6-iminopyridyl metal complexes, Progress in Olefin Polymerization Catalysts and Polyolefin Materials, ed. T. Shiono, K. Nomura and M. Terano, Elsevier B.V., 2006, vol. 161, pp. 141–146 Search PubMed.
- G. W. Son, K. B. Bijal, D. Park, C. Ha and I. Kim, Catal. Today, 2006, 111, 412–416 CrossRef CAS.
- C. D. Rosa, R. D. Girolamo and G. Talarico, A General Model to Explain the Isoselectivity of Olefin Polymerization Catalysts, in Computational Quantum Chemistry, Elsevier, 2019, ch. 8, pp. 269–285 Search PubMed.
- E. G. IJpeij, F. H. Beijer, H. J. Arts, C. Newton, J. G. Vries and G. M. Gruter, J. Org. Chem., 2002, 67, 169–176 CrossRef CAS PubMed.
- Y. Du, A. McSkimming, J. T. Mague and R. A. Pascal Jr, Chem.–Eur. J., 2022, 28, e202200931 CAS.
- J. Deng, T. Zhang, B. Li, M. Xu and Y. Wang, Eur. J. Med. Chem., 2022, 239, 114553 CrossRef CAS PubMed.
- L. Jean, M. Pouliquen, J. Blanchet, M. Lasne and J. Rouden, Sci. China: Chem., 2010, 53, 1907–1913 CrossRef CAS.
- H. Ahmad, S. Ullah, F. Rahman, A. Saeed, J. Pelletier, J. Sévigny, A. Hassan and J. Iqbal, Eur. J. Med. Chem., 2020, 208, 112759 CrossRef CAS PubMed.
- P. Zhu, J. Zhang, Y. Yang, L. Wang, J. Zhou and H. Zhang, Mol. Diversity, 2022, 26, 245–264 CrossRef CAS PubMed.
- A. R. Kapdi and Y. S. Sanghvi, The future of drug discovery: the importance of modified nucleosides, nucleotides,
and oligonucleotides, in Palladium-catalyzed modification of nucleosides, nucleotides and oligonucleotides, Elsevier, 2018, pp. 1–18 Search PubMed.
- J. Li, Y. Liang, D. Wang, W. Liu, Y. Xie and D. Yin, J. Org. Chem., 2005, 70, 2832–2834 CrossRef CAS PubMed.
- P. Vosáhlo, I. Císařová and P. Štěpnička, New J. Chem., 2022, 46, 21536–21552 RSC.
- S. Xu, E. H. Kim, A. Wei and E. Negishi, Sci. Technol. Adv. Mater., 2014, 15, 044201 CrossRef CAS PubMed.
- H. Kohzadi and M. Soleiman-Beigi, Mol. Diversity, 2022, 26, 1957–1967 CrossRef CAS PubMed.
- P. Espinet and A. M. Echavarren, Angew. Chem., Int. Ed., 2004, 43, 4704–4734 CAS.
- L. Huo, X. Wang, Z. Zhang, Z. Jia, X. Peng and H. N. C. Wong, Chem. Sci., 2023, 14, 1342–1362 RSC.
- M. Rottländer, N. Palmer and P. Knochel, Synlett, 1996, 573–575 CrossRef.
- V. B. Phapale and D. J. Cárdenas, Chem. Soc. Rev., 2009, 38, 1598–1607 RSC.
- J. Mai, M. Morasch, D. Jędrzkiewicz, J. Langer, B. Rösch and S. Harder, Angew. Chem., Int. Ed., 2023, 135, e202212463 CrossRef.
- T. B. Poulsen and K. A. Jørgensen, Chem. Rev., 2008, 108, 2903–2915 CrossRef CAS PubMed.
- S. Csihony, H. Mehdi and I. T. Horváth, Green Chem., 2001, 3, 307–309 RSC.
- I. N. Jung, A. R. Cho, S. H. Kang and Y. M. Kim, US Pat., US2021/0188739A1, 2021 Search PubMed.
- J. H. Yao, C. Zhen, K. P. Loh and Z. Chen, Tetrahedron, 2008, 64, 10814–10820 CrossRef CAS.
- R. Rathore and S. H. Wadumethrige, J. Photochem. Photobiol., A, 2019, 382, 111882 CrossRef CAS.
- W. Chen and M. Oestreich, Org. Lett., 2019, 21, 4531–4534 CrossRef CAS PubMed.
- L. Xianwei, M. Qingsong, M. Xinwang, S. Dongyan, W. Lijing, L. Pengying and Z. Huazhi, CN111635287A, 2020.
- L. Xianwei, M. Xinwang, M. Qingsong, S. Dongyan, W. Lijing, L. Jiao and S. Jian, CN111606784A, 2020.
- H. Firouzabadi, N. Iranpoor and F. Nowrouzi, Tetrahedron, 2004, 60, 10843–10850 CrossRef CAS.
- H. Sharghi, M. Jokar, M. M. Doroodmand and R. Khalifeh, Adv. Synth. Catal., 2010, 352, 3031–3044 CrossRef CAS.
- R. Guo, J. Huang, H. Huang and X. Zhao, Org. Lett., 2016, 18, 504–507 CrossRef CAS PubMed.
- J. A. Cisneros, E. Björklund, I. Gonzalez-Gil, Y. Hu, A. Canales, F. J. Medrano, A. Romero, S. Ortega-Gutiérrez, C. J. Fowler and M. L. Lopez-Rodriguez, J. Med. Chem., 2012, 55, 824–836 CrossRef CAS PubMed.
- M. Đud, A. Briš, I. Jušinski, D. Gracin and D. Margetić, Beilstein J. Org. Chem., 2019, 15, 1313–1320 CrossRef PubMed.
- E. V. Fedorenko, A. G. Mirochnik and A. Y. Beloliptsev, J. Lumin., 2018, 196, 316–325 CrossRef CAS.
- X. Pang, L. Wang, D. Kang, Y. Zhao, S. Wu, A. Liu and G. Du, Molecules, 2017, 22, 1246 CrossRef PubMed.
- H. Jin, L. Xianwei, M. Qingsong, C. Fang, W. Xiaoying, M. Xinwang and S. Jian, CN112358380A, 2021.
- C. R. Oh, J. H. Lee, D. R. Lee, M. S. Lee, W. J. Lee and Y. I. Cho, KR20170035697A, 2017.
- F. Picard, T. Schulz and R. W. Hartmann, Bioorg. Med. Chem., 2002, 10, 437–448 CrossRef CAS PubMed.
- J. W. Williams and J. M. Osborn, J. Am. Chem. Soc., 1939, 61, 3438–3439 CrossRef CAS.
- Z. Zhang, Y. Zhao, R. Zhang, L. Zhang, W. Cheng and Z. H. Ni, Dyes Pigm., 2015, 118, 95–101 CrossRef CAS.
- N. Crosta, S. Müller, D. Gradl, K. Masters and S. Bräse, Synlett, 2013, 24, 951–954 CrossRef CAS.
- C. Melero, A. Guijarro and M. Yus, Tetrahedron Lett., 2006, 47, 6267–6271 CrossRef CAS.
- H. Kawagoe, K. Komura, J. Kim, G. Seo and Y. Sugi, J. Mol. Catal. A: Chem., 2011, 350, 1–8 CrossRef CAS.
- M. D. Erickson and R. G. Kaley, Environ. Sci. Pollut. Res. Int., 2011, 18, 135–151 CrossRef CAS PubMed.
- H. A. Muathen, Tetrahedron, 1996, 52, 8863–8866 CrossRef CAS.
- F. R. Shaw and E. E. Turner, J. Chem. Soc., 1932, 285–297 RSC.
- M. Bolgar, J. Cunningham, R. Cooper, R. Kozloski, J. Hubball, D. P Miller, T. Crone, H. Kimball, A. Janooby, B. Miller and B. Fairless, Chemosphere, 1995, 31, 2687–2705 CrossRef CAS.
- A. Jităreanu, I. C. Caba and L. Agoroaei, Curr. Anal. Biotechnol., 2019, 2, 11–25 Search PubMed.
- R. V. D. Recke and W. Vetter, Chemosphere, 2008, 71, 352–359 CrossRef PubMed.
- Q. Weijun, X. Haiwang and C. Weibing, CN107602339A, 2018.
- Y. Nishii, M. Ikeda, Y. Hayashi, S. Kawauchi and M. Miura, J. Am. Chem. Soc., 2020, 142, 1621–1629 CrossRef CAS PubMed.
- R. D. S. Ribeiro, P. M. Esteves and M. C. S. D. Mattos, J. Braz. Chem. Soc., 2012, 23, 228–235 CAS.
- W. Neugebauer, A. J. Kos and P. V. R. Schleyer, J. Organomet. Chem., 1982, 228, 107–118 CrossRef CAS.
- T. M. Klapötke, B. Krumm and M. Scherr, Z. Anorg. Allg. Chem., 2010, 636, 1955–1961 CrossRef.
- Z. H. Li, M. S. Wong, Y. Tao and M. D'Iorio, J. Org. Chem., 2004, 69, 921–927 CrossRef CAS PubMed.
- J. Tang, H. Liu, J. Zhou and X. Zhang, J. Chem. Res., 2019, 43, 34–38 CrossRef CAS.
- L. Mingxing, T. Qing and W. Jianhong, CN106905177A, 2017.
- A. J. Birch and G. Nadamuni, J. Chem. Soc., Perkin Trans. 1, 1974, 545–552 RSC.
- A. J. Durie, T. Fujiwara, R. Cormanich, M. Bühl, A. M. Z. Slawin and D. O'Hagan, Chem.–Eur. J., 2014, 20, 6259–6263 CrossRef CAS PubMed.
- H. Wang, H. Wang, E. Kuhn, M. P. Tucker and B. Yang, ChemSusChem, 2018, 11, 285–291 CrossRef CAS PubMed.
- A. A. Philippov, A. M. Chibiryaev and O. N. Martyanov, Catal. Today, 2021, 379, 15–22 CrossRef CAS.
- N. G. Boekell, C. O. Bartulovich, S. Maity and R. A. Flowers, Inorg. Chem., 2023, 62, 5040–5045 CrossRef CAS PubMed.
- R. Garg, M. Patel, P. Kolambkar, M. Kadam, D. Makade and R. Bhatta, WO2020/144094A1, 2020.
- Z. Hong, X. Han, W. Kewei and H. Jianguo, CN113149918A, 2021.
- H. Zhang, Y. Huang, J. Tian, P. Bao, X. Fan, C. Sun, D. Sun, C. Xiong, B. Guan, Z. Bi and H. Li, CN110372620A, 2019.
- T. A. H. Nguyen and D. Hou, Org. Lett., 2021, 23, 8127–8131 CrossRef PubMed.
- G. P. Luke, C. K. Seekamp, Z. Wang and B. L. Chenard, J. Org. Chem., 2008, 73, 6397–6400 CrossRef CAS PubMed.
- P. H. Tran, P. E. Hansen, H. T. Nguyen and T. N. Le, Tetrahedron Lett., 2015, 56, 612–618 CrossRef CAS.
- R. A. Khalaf, G. A. Sheikha, Y. Bustanji and M. O. Taha, Eur. J. Med. Chem., 2010, 45, 1598–1617 CrossRef PubMed.
- A. Manchoju and S. V. Pansare, Org. Lett., 2016, 18, 5952–5955 CrossRef CAS PubMed.
- A. Antenucci, M. Barbero, S. Dughera and G. Ghigo, Tetrahedron, 2020, 76, 131632 CrossRef CAS.
- Y. Du, F. Yao and M. Cai, J. Chem. Res., 2020, 44, 174–180 CrossRef CAS.
- K. Sun, Y. Li and Q. Zhang, Sci. China: Chem., 2015, 58, 1354–1358 CrossRef CAS.
- A. Maleki, M. Aghaei and R. Paydar, J. Iran. Chem. Soc., 2017, 14, 485–490 CrossRef CAS.
- S. A. Osadchii, V. G. Shubin, L. P. Kozlova, V. S. Varlamenko, M. L. Filipenko and U. A. Boyarskikh, Russ. J. Appl. Chem., 2011, 84, 1541–1548 CrossRef CAS.
- S. L. Herlihy, WO2003/072567A1, 2003.
- G. Gulyas-Fekete, C. J. Boluda, B. Westermann and L. A. Wessjohann, Synthesis, 2013, 45, 3038–3043 CrossRef CAS.
- S. Maiti and P. Mal, J. Org. Chem., 2018, 83, 1340–1347 CrossRef CAS PubMed.
- T. Morofuji, A. Shimizu and J. Yoshida, J. Am. Chem. Soc., 2013, 135, 5000–5003 CrossRef CAS PubMed.
- N. A. Romero, K. A. Margrey, N. E. Tay and D. A. Nicewicz, Science, 2015, 349, 1326–1330 CrossRef CAS PubMed.
- A. A. Kashid, D. J. Patil, R. D. Mali, V. P. Patil, T. V. Neethu, H. K. Meroliya, S. A. Waghmode and S. Iyer, Catal. Lett., 2021, 151, 353–358 CrossRef CAS.
- M. N. Eliseeva and L. T. Scott, J. Am. Chem. Soc., 2012, 134, 15169–15172 CrossRef CAS PubMed.
- S. A. Rzhevskiy, M. A. Topchiy, Y. D. Golenko, P. S. Gribanov, G. K. Sterligov, N. Y. Kirilenko, A. A. Ageshina, M. V. Bermeshev, M. S. Nechaev and A. F. Asachenko, Mendeleev Commun., 2020, 30, 569–571 CrossRef CAS.
- L. Z. Fekri and M. Farjood, Org. Prep. Proced. Int., 2021, 53, 291–300 CrossRef.
- A. Dewanji, L. V. Dalsen, J. A. Rossi-Ashton, E. Gasson, G. E. M. Crisenza and D. J. Procter, Nat. Chem., 2023, 15, 43–52 CrossRef CAS PubMed.
- J. B. McManus and D. A. Nicewicz, J. Am. Chem. Soc., 2017, 139, 2880–2883 CrossRef CAS PubMed.
- J. Liu, X. Zhao, L. Jiang and W. Yi, Adv. Synth. Catal., 2018, 360, 4012–4016 CrossRef CAS.
- L. Buglioni, M. Beslać and T. Noël, J. Org. Chem., 2021, 86, 16195–16203 CrossRef CAS PubMed.
- Z. Wen, T. Wan, A. Vijeta, C. Casadevall, L. Buglioni, E. Reisner and T. Noël, ChemSusChem, 2021, 14, 5265–5270 CrossRef CAS PubMed.
- P. P. Sen and S. R. Roy, Org. Lett., 2023, 25, 1895–1900 CrossRef CAS PubMed.
- S. Das, P. Natarajan and B. König, Chem.–Eur. J., 2017, 23, 18161–18165 CrossRef CAS PubMed.
- A. Malani, A. Makwana, J. Monapara, I. Ahmad, H. Patel and N. Desai, J. Biochem. Mol. Toxicol., 2021, 35, e22903 CrossRef CAS PubMed.
- S. Auras and O. Trapp, Helv. Chim. Acta, 2021, 104, e2100147 CrossRef CAS.
- C. H. Christie and J. Kenner, J. Chem. Soc., 1922, 121, 614–620 RSC.
- G. Bringmann, A. J. P. Mortimer, P. A. Keller, M. J. Gresser, J. Garner and M. Breuning, Angew. Chem., Int. Ed., 2005, 44, 5384–5427 CrossRef CAS PubMed.
- D. C. Patel, R. M. Woods, Z. S. Breitbach, A. Berthod and D. W. Armstrong, Tetrahedron: Asymmetry, 2017, 28, 1557–1561 CrossRef CAS.
- M. C. Kozlowski, S. J. Miller and S. Perreault, Acc. Chem. Res., 2023, 56, 187–188 CrossRef CAS PubMed.
- P. W. Glunz, Bioorg. Med. Chem. Lett., 2018, 28, 53–60 CrossRef CAS PubMed.
- J. E. Smyth, N. M. Butler and P. A. Keller, Nat. Prod. Rep., 2015, 32, 1562–1583 RSC.
- E. Masson, Org. Biomol. Chem., 2013, 11, 2859–2871 RSC.
- A. Mazzanti, L. Lunazzi, R. Ruzziconi, S. Spizzichino and M. Schlosser, Chem.–Eur. J., 2010, 16, 9186–9192 CrossRef CAS PubMed.
- S. R. LaPlante, P. J. Edwards, L. D. Fader, A. Jakalian and O. Hucke, ChemMedChem, 2011, 6, 505–513 CrossRef CAS PubMed.
- T. Wang, S. Cai, M. Wang, W. Zhang, K. Zhang, D. Chen, Z. Li and S. Jiang, J. Med. Chem., 2021, 64, 7390–7403 CrossRef CAS PubMed.
- S. E. Hage, B. Lajoie, C. Feuillolay, C. Roques and G. Baziard, Arch. Pharm., 2011, 344, 402–410 CrossRef PubMed.
- G. Menozzi, L. Mosti, P. Fossa, C. Musiu, C. Murgioni and P. L. Colla, Farmaco, 2001, 56, 633–640 CrossRef CAS PubMed.
- S. Konduri, V. Pogaku, J. Prashanth, V. S. Krishna, D. Sriram, S. Basavoju, J. N. Behera and K. P. Rao, ChemistrySelect, 2021, 6, 3869–3874 CrossRef CAS.
- S. Ayalasomayajula, T. Langenickel, P. Pal, S. Boggarapu and G. Sunkara, Clin. Pharmacokinet., 2017, 56, 1461–1478 CrossRef CAS PubMed.
- S. Ryu, J. Shin, Y. Cho, H. K. Kim, S. H. Paik, J. H. Lee, Y. H. Chi, J. H. Kim, J. H. Kim and K. Lee, Biol. Pharm. Bull., 2013, 36, 467–474 CrossRef CAS PubMed.
- S. M. Azab and A. M. Fekry, RSC Adv., 2017, 7, 1118–1126 RSC.
- N. A. Meanwell and M. Belema, The discovery and development of daclatasvir: an inhibitor of the hepatitis C virus NS5A replication complex, HCV: The Journey from Discovery to a Cure, 2019, vol. 32, pp. 27–56 Search PubMed.
- M. B. Zewail, S. A. El-Gizawy, M. A. Osman and Y. A. Haggag, J. Drug Delivery Sci. Technol., 2021, 61, 102320 CrossRef CAS.
- D. Ntountaniotis, I. Andreadelis, T. F. Kellici, V. Karageorgos, G. Leonis, E. Christodoulou, S. Kiriakidi, J. Becker-Baldus, E. K. Stylos, M. V. Chatziathanasiadou, C. M. Chatzigiannis, D. E. Damalas, B. Aksoydan, U. Javornik, G. Valsami, C. Glaubitz, S. Durdagi, N. S. Thomaidis, A. Kolocouris, J. Plavec, A. G. Tzakos, G. Liapakis and T. Mavromoustakos, Mol. Pharmaceutics, 2019, 16, 1255–1271 CrossRef CAS PubMed.
- H. Zhang, M. O. Ihara, N. Nakada, H. Tanaka and M. Ihara, Environ. Sci. Technol., 2020, 54, 1720–1729 CrossRef CAS PubMed.
- S. S. Hegde, M. T. Pulido-Rios, M. A. Luttmann, J. J. Foley, G. E. Hunsberger, T. Steinfeld, T. Lee, Y. Ji, M. M. Mammen and J. R. Jasper, Pharmacol. Res. Perspect., 2018, 6, e00400 CrossRef PubMed.
- J. A. Wren, V. L. King, S. L. Campbell and M. A. Hickman, J. Vet. Pharmacol. Ther., 2007, 30, 33–42 CrossRef CAS PubMed.
- G. M. Happi, G. P. M. Kemayou, H. Stammler, B. Neumann, M. Ismail, S. F. Kouam, J. D. Wansi, J. C. Tchouankeu, M. Frese, B. N. Lenta and N. Sewald, Phytochemistry, 2021, 181, 112537 CrossRef CAS PubMed.
- A. Hicks, G. P. McCafferty, E. Riedel, N. Aiyar, M. Pullen, C. Evans, T. D. Luce, R. W. Coatney, G. C. Rivera, T. D. Westfall and J. P. Hieble, J. Pharmacol. Exp. Ther., 2007, 323, 202–209 CrossRef CAS PubMed.
- A. B. M. Grudell, M. Camilleri, K. L. Jensen, A. E. Foxx-Orenstein, D. D. Burton, M. D. Ryks, K. L. Baxter, D. S. Cox, G. E. Dukes, D. L. Kelleher and A. R. Zinsmeister, Am. J. Physiol.: Gastrointest. Liver Physiol., 2008, 294, G1114–G1119 CrossRef CAS.
- N. S. O. Ujiantari, S. Ham, C. Nagiri, W. Shihoya, O. Nureki, D. S. Hutchinson and D. Schuster, Mol. Inf., 2022, 41, 2100223 CrossRef CAS PubMed.
- Y. Liu, A. Kumar, S. Depauw, R. Nhili, M. David-Cordonnier, M. P. Lee, M. A. Ismail, A. A. Farahat, M. Say, S. Chackal-Catoen, A. Batista-Parra, S. Neidle, D. W. Boykin and W. D. Wilson, J. Am. Chem. Soc., 2011, 133, 10171–10183 CrossRef CAS PubMed.
- M. A. Ismail, R. Brun, T. Wenzler, F. A. Tanious, W. D. Wilson and D. W. Boykin, J. Med. Chem., 2004, 47, 3658–3664 CrossRef CAS PubMed.
- M. A. Ismail, R. Brun, T. Wenzler, F. A. Tanious, W. D. Wilson and D. W. Boykin, Bioorg. Med. Chem., 2004, 12, 5405–5413 CrossRef CAS PubMed.
- H. Wenlong, Q. Hai, L. Zheng, W. Xuekun, J. Lei and Q. Qianqian, CN105017242A, 2015.
- D. S. Karlov, N. S. Temnyakova, D. A. Vasilenko, O. I. Barygin, M. Y. Dron, A. S. Zhigulin, E. B. Averina, Y. K. Grishin, V. V. Grigoriev, A. V. Gabrel’yan, V. A. Aniol, N. V. Gulyaeva, S. V. Osipenko, Y. I. Kostyukevich, V. A. Palyulin, P. A. Popov and M. V. Fedorov, RSC Med. Chem., 2022, 13, 822–830 RSC.
- S. Ghosh, A. S. Kumar and G. N. Mehta, Beilstein J. Org. Chem., 2010, 6, 1–4 Search PubMed.
- I. Peretto, S. Radaelli, C. Parini, M. Zandi, L. F. Raveglia, G. Dondio, L. Fontanella, P. Misiano, C. Bigogno, A. Rizzi, B. Ricccardi, M. Biscaioli, S. Marchetti, P. Puccini, S. Catinella, I. Rondelli, V. Cenacchi, P. T. Bolzoni, P. Caruso, G. Villetti, F. Facchinetti, E. D. Giudice, N. Moretto and B. P. Imbimbo, J. Med. Chem., 2005, 48, 5705–5720 CrossRef CAS PubMed.
- B. Chowdhury, M. Adak and S. K. Bose, Lett. Appl. Microbiol., 2003, 37, 158–161 CrossRef CAS PubMed.
- B. S. Takale, R. R. Thakore, F. Y. Kong and B. H. Lipshutz, Green Chem., 2019, 21, 6258–6262 RSC.
- R. S. Vardanyan and V. J. Hruby, Analgesics. Synthesis of Essential Drugs, Elsevier, 2006, pp. 19–55, ISBN: 9780080462127 Search PubMed.
- J. Hannah, W. V. Ruyle, H. Jones, A. R. Matzuk, K. W. Kelly, B. E. Witzel, W. J. Holtz, R. A. Houser, T. Y. Shen and L. H. Sarett, J. Med. Chem., 1978, 21, 1093–1100 CrossRef CAS PubMed.
- Z. Chen, Y. Guo, H. Niu, J. Wang, J. Li, C. Li and R. Qiao, Org. Process Res. Dev., 2022, 26, 2438–2446 CrossRef CAS.
- T. Zhou, Y. Xie, X. Hou, W. Bai, X. Li, Z. Liu, Q. Man, J. Sun, D. Fu, J. Yan, Z. Zhang, Y. Wang, H. Wang, W. Jiang, S. Gao, T. Zhao, A. Chang, X. Wang, H. Sun, X. Zhang, S. Yang, C. Haung, J. Hao and J. Liu, J. Exp. Clin. Cancer Res., 2023, 42, 1–27 CrossRef PubMed.
- H. Wang, Z. Lu, Y. Li, T. Liu, L. Zhao, T. Gao, X. Lu and B. Gao, Molecules, 2023, 28, 2643 CrossRef CAS PubMed.
- Y. Jinfei, CN113861130A, 2021.
- C. Fener, Z. Chunlin and D. Li, CN112624983A, 2021.
- Z. Houwei and G. Youwen, CN112608250A, 2021.
- K. D. Sergeevich, RU2736511C1, 2020.
- Z. Youjun, S. Nannan, Z. Ju, L. Jiaguo, Z. Canhui and J. Junhang, CN104230611A, 2014.
- N. G. Marina and C. B. Pelaez, WO2013/076170A1, 2013.
- A. S. Mahmoud, N. J. Owaid and R. M. Al-Ezzy, Asian J. Biol. Sci., 2020, 13, 270–284 CrossRef CAS.
- B. Wang, Y. Gao, L. Chen, C. Zhang, X. Zhang and H. Zhang, Chem. Biodiversity, 2020, 17, e1900609 CAS.
|
This journal is © The Royal Society of Chemistry 2023 |