DOI:
10.1039/D3RA03478J
(Paper)
RSC Adv., 2023,
13, 22928-22935
Rapid synthesis of functional poly(ester amide)s through thiol–ene chemistry†
Received
24th May 2023
, Accepted 19th July 2023
First published on 28th July 2023
Abstract
Poly(ester amide)s (PEAs) bearing various side chains were synthesized by post-polymerization modification of PA-1, a vinylidene containing PEA. The thiols 1-dodecanethiol (1A-SH), 2-phenylethanethiol (1B-SH), 2-mercaptoethanol (1C-SH), thioglycolic acid (1D-SH), furfuryl mercaptan (1E-SH) and sodium-2-mercaptoethanesulfonate (1F-SH) were reacted with PA-1 to form PEAs PA-1A through PA-1F respectively. PEAs containing non-polar thiol side chains (PA-1A, PA-1B, PA-1E), showed little change in solubility compared to PA-1, while PEAs with more polar side chains improved solubility in more polar solvents. PA-1F, functionalized with sodium-2-mercaptoethanesulfonate, became water-soluble. The introduction of pendant functional groups impacted the thermal behaviors of PEAs in a wide range. The PEAs were thermally stable up to 368 °C, with glass transition temperatures (Tg) measured between 117 to 152 °C. Moreover, to demonstrate the versatility of the PEAs, thermal reprocessable networks and polyurethanes were successfully fabricated by reacting with a bismaleimide (1,6-bis(maleimido)hexane, 1,6-BMH) and a diisocyanate (4,4′-diphenylmethane diisocyanate, 4,4′-MDI), respectively. This study paves the way for the facile synthesis of functional poly(ester amide)s with great potential in many fields.
Introduction
Poly(ester amide)s (PEAs) are polymers with ester and amide linkages in their polymeric backbone.1 PEAs combine the good biocompatibility and environmental degradability of polyesters with the high thermal stability and mechanical strength of polyamides. In recent years, these promising materials received increasing attention in various fields, especially in biomedical applications. For example, PEAs have been applied in controlled drug delivery, non-viral gene carriers, hydrogels, and tissue engineering scaffolds.2–5
Typical synthetic methods for PEAs are polycondensation or ring-opening polymerization (ROP).1,5 For instance, Li synthesized a series of semi-aromatic PEAs via the ROP of eight-membered cyclic(ester-amide)s in the presence of organocatalysts such as 1,5,7-triazabicyclo[4.4.0]-dec-5-ene (TBD) or 1,8-diazabicyclo[5.4.0]undecane-7-ene (DBU)/thiourea (TU) (Scheme 1a).6 Loos reported a strategy for the enzymatic polymerization of furan-based poly(ester amide)s. PEAs (Mn up to 4.4 kDa) were successfully synthesized by the polycondensation of dimethyl 2,5-furandicarboxylate (DMFDCA) with aliphatic diols, diamines, or amino alcohols in ionic liquids – BMIMPF6 or EMIMBF4 (Scheme 1b).7 Other methods like polyaddition of bis(2-oxazoline)s and dicarboxylic acids have also been used for the preparation of PEAs (Scheme 1c).8 Despite the many merits of these methods, an inert atmosphere is often required for polymerizations, and there are restrictions on the available functional groups in the final polymer.
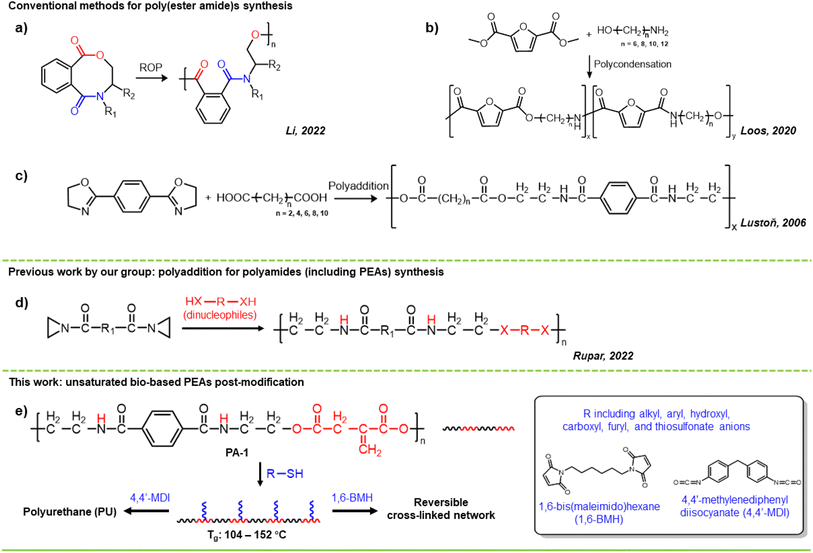 |
| Scheme 1 (a) Ring-opening polymerization for PEAs synthesis; (b) polycondensation for PEAs synthesis; (c) polyaddition for PEAs synthesis; (d) polyamides (including PEAs) prepared by step-growth polyaddition of bis(N-carbonyl aziridine)s; (e) unsaturated bio-based PEAs post-polymerization modification (this work). | |
Aziridines, three-membered heterocycles with ring strain, are versatile and powerful building blocks in polymer synthesis.9–11 Aziridine and other nonactivated aziridines tend to polymerize via chain-growth, cationic ring-opening polymerizations (CROPs), often forming branched structures.12–14 In contrast, aziridines activated with electron-withdrawing groups on the nitrogen (typically sulfonyl groups), polymerize anionically to form linear polymers, of which many have been reported.9,10,15–24 Recently, bissulfonyl aziridines are also finding application in step-growth polymerizations. For example, Yoon and Zhang independently reported a series of bis(N-sulfonyl aziridine) monomers, which can be polymerized with various dinucleophiles such as dicarboxylic acids, diphenols, dithiols and diamines.25–29
We recently reported on chain-growth AROP of carbamate activated aziridines11 and the step-growth polymerization bis(N-carbonyl aziridine)s with dinucleophiles (Scheme 1d).30 We found that bis(N-carbonyl aziridine)s rapidly polymerize with a variety of dinucleophiles, including dicarboxylic acids to produced PEAs.31–33 These polymerizations were conducted under mild conditions and are very functional group tolerant, allowing the synthesis of highly functional PEAs (e.g., PA-1 in Scheme 1e).
We propose that PA-1 (and similar PEAs) can be valuable, sustainably sourced polymers for a wide range of applications. In this paper, we explore the post-polymerization modification of PA-1, which forms from the copolymerization of postconsumer PETE-derived terephthalaziridine (TP-Az) and itaconic acid (Scheme 1e). We use thiol–ene chemistry to synthesize a variety of PEAs from the renewably sourced PA-1, creating polymers with tunable thermal properties, a thermal reprocessable network, and a polyurethane film (Scheme 1e).
Results and discussion
Thiol–ene couplings are a powerful synthetic tool for the post-polymerization modification of polymers.34 However, to the best of our knowledge, there are only a few examples of polymerization modification of PEAs using thiol–ene chemistry. Furthermore, the preparation of the parent PEAs in those studies was relatively complicated and only a few thiol reagents were examined for post-polymerization modification.35,36
We synthesized a single batch of PA-1 from the copolymerization of TP-Az and itaconic acid for use in this study.30 PA-1 was isolated as white power with an Mn of 6.4 kDa, dispersity of 2.08, Tg of 117 °C, and onset of thermal mass loss of 225 °C (Table 1). We next selected a variety of thiols to react with the vinylidene groups of PA-1, so to explore the various properties of the resulting PEAs and to introduce new functionalities (Scheme 2). Thiols 1-dodecanethiol and 2-phenylethanethiol were chosen with the anticipation that the introduction of the alkyl and phenyl groups will increase hydrophobicity and improve solubility in non-polar solvents.37 Next, 2-mercaptoethanol was chosen for its ability to add hydroxyl groups, which could results in a significant change in hydrogen bonding interactions and increases the possibility of further modification reactions, like substitution, elimination, oxidation, etc.38 Similarly, thioglycolic acid, which containing a carboxyl group, may endow PEAs with tunable hydrogen bond strength and coordinative sites for potential applications.39 Furfuryl mercaptan was then explored as this should allow reversible Diels–Alder reactions, which we intend to use for reversible network formation with bismalemides.40 Finally, sodium-2-mercaptoethanesulfonate was selected to introduce anionic moieties into the polymer backbones. This was done to study potential differences in solubility and thermal properties of neutral and ionic poly(ester amide)s.41
Table 1 Poly(ester amide) (PA-1) post-modifications (thiol–ene click reactions) with various thiol reagents were performed at 50 °C for 24 h
Entry |
Polymer |
Functional thiol |
Mna/kDa |
Đa |
Yield (%) |
Td,5%b (°C) |
Tgc (°C) |
Number-average molecular weight (Mn) and dispersity (Đ) determined by GPC in HFIP at 35 °C (PMMA calibration). The temperatures at which a weight loss of 5% (Td,5%, °C) of the PEAs are recorded as the decomposition temperatures. Differential scanning calorimetry (DSC) of the synthesized poly(ester amide)s (PEAs). The data from the 3rd heating cycle is reported. |
1 |
PA-1 |
n/a |
6.4 |
2.08 |
80 |
225 |
117 |
2 |
PA-1A |
1-Dodecanethiol |
12.7 |
2.02 |
76 |
309 |
119 |
3 |
PA-1B |
2-Phenylethanethiol |
11.4 |
1.81 |
70 |
316 |
112 |
4 |
PA-1C |
2-Mercaptoethanol |
8.3 |
2.14 |
89 |
368 |
104 |
5 |
PA-1D |
Thioglycolic acid |
5.3 |
3.51 |
84 |
249 |
123 |
6 |
PA-1E |
Furfuryl mercaptan |
9.4 |
2.17 |
75 |
267 |
109 |
7 |
PA-1F |
Sodium-2-mercaptoethanesulfonate |
10.3 |
2.31 |
79 |
270 |
152 |
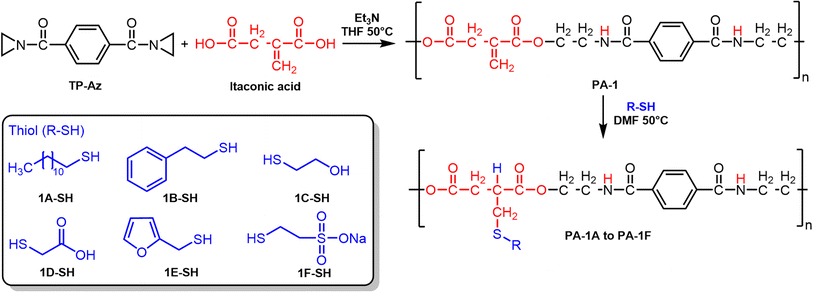 |
| Scheme 2 Step-growth polymerizations of TP-Az with itaconic acid for PA-1 synthesis, and thiol–ene click reactions based on PA-1 with various thiol reagents. | |
All the thiol–ene reactions with PA-1 were performed under mild conditions (50 °C, 24 h), without the assistance of any radical initiators or base, to produce PA-1A through PA-1F. In each case, the desired polymer product was confirmed by 1H NMR spectroscopic analysis (Fig. 1 and S2–S7†). Using the 1H NMR spectrum of PA-1A as an example (Fig. 1), the characteristic signals of the vinylidene protons at δ = 6.22 and 5.79 ppm disappeared and signals corresponding to the protons of the long aliphatic chain were observed at 1.37, 1.19, and 0.82 ppm. Other chemical shifts from the 1H NMR spectrum were assigned to different protons of the PA-1A backbone. For example, the signals at δ = 4.11 and 3.49 ppm are attributed to the –CH2–CH2– linkages in the backbone formed by the ring opening of TP-Az. The signal at δ = 7.90 ppm is from the phenylene protons originated from TP-Az and the resonance at δ = 8.65 ppm is attributed to the amide proton. The GPC trace for PA-1A showed a shift to higher molecular weight compared to PA-1 (Mn,GPC = 12.7 kDa, Đ = 2.02, Table 1 and Fig. S11†). The estimated molecular weight of PA-1A (Mn,GPC = 12.7 kDa, n = 23) is comparable to the calculated molecular weight (Mn,GPC = 10.4 kDa, n = 19) based on the polymerization degree of the parent PA-1. Similar increases in the molecular weights of the remaining thiol–ene modified PEAs were also observed (Table 1 and Fig. S11†). These result, together with NMR analysis, suggest that the thiol–ene coupling proceeded quantitatively.
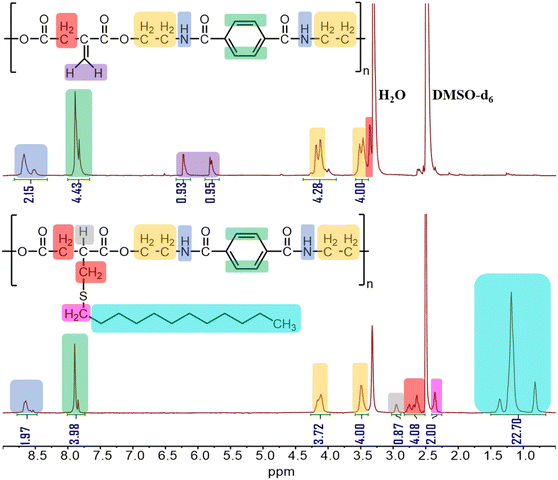 |
| Fig. 1 1H NMR spectrum (500 MHz, DMSO-d6) of poly(ester amide)s PA-1 (top, the template) and PA-1A (bottom, after modification). | |
Solubility properties
We observed that the solubilities of the PEAs are impacted by the nature of the thiol–ene grafted side chains. The parent PA-1 is soluble in DMF, DMSO, and HFIP, but it is insoluble in THF, toluene, DCM, n-hexane, methanol, and water. When neutral side chains were added to polymer backbones (i.e., PA-1A to PA-1E), there was no significant changes in solubility compared to PA-1. However, the sodium sulfonate containing PA-1F exhibited improved solubility, as it became soluble in methanol and water, in addition to DMF, DMSO, and HFIP.
One basic characteristic of ionic polymers is that the molecular structure contains covalent and ionic bonds. Previous research has explored water-soluble neutral and cationic PEAs,42,43 but as far as we know, water-soluble anionic PEAs have not been reported before. The changed solubility may allow different processing options and possible applications.
Thermal properties
The thermal properties of poly(ester amide)s PA-1 and PA-1A to PA-1F were examined by thermogravimetric analysis (TGA) and differential scanning calorimetry (DSC). The parent polymer (PA-1) remained stable to mass loss until 225 °C. All the modified PEAs (PA-1A to PA-1F) exhibited improved thermal stability with onsets of mass loss ranging from 249 °C to a remarkably high 368 °C (Fig. S8† and Table 1). These data indicated that the addition of pedant groups increased poly(ester amide)s’ thermal stability; similar results have been observed in other polymeric systems modified by thiol–ene click chemistry.44 The highest decomposition temperature was observed in PA-1C (368 °C), which maybe be attributed to the strong inter- and intra-polymer chains hydrogen bonding interaction caused by the hydroxyl substituents. We hypothesize that the relatively poor thermal stability of PA-1D (249 °C) is due to the –COOH pendant groups catalyzing chain scission.
The glass transition temperature (Tg) for the parent PA-1 was measured to be 117 °C by DSC. Upon thiol–ene modification, the Tg of PA-1A to PA-1F were found to be between 104 to 152 °C. The highest glass transition temperature was observed for the sodium 2-thiosulfonate containing PA-1F; anionic moieties are known to increase internal rotation activation energy barriers that can contribute to increased Tg.45 We observed that the PEAs with non-polar side chains (i.e., PA-1A, PA-1B, and PA-1E), showed very little variation in Tg, suggesting that these side chains do little interrupt in chain packing or that they do not induce internal plasticization. The lowest Tg (104 °C) was observed for the alcohol decorated PA-1C (Fig. 2, S10,† and Table 1). According to literature reports, polyols are widely applied as polymer plasticizer.46–48 The high density of hydroxyl groups in PA-1C is likely responsible for the decrease in Tg via a similar intermolecular interaction.
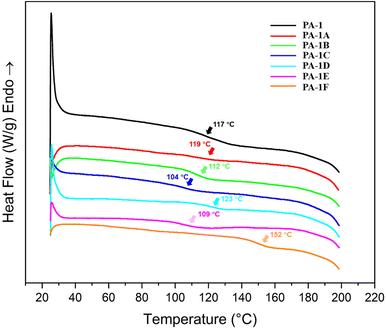 |
| Fig. 2 The DSC curves of PEAs with various pendant thiol groups (heating/cooling rate, 10 °C min−1; under nitrogen). All samples were heated through 3 complete heating/cooling cycles, and the data from the 3rd heating cycle were used for plotting. | |
As mentioned above, one advantage of poly(ester amide)s over polyesters is superior thermal performance. For instance, the reported Tg of polylactide (PLA) is in a temperature range of 35–60 °C,49 which is at least 50 °C lower than the Tg of PA-1 (Tg = 117 °C). As thermoplastics, improved Tg's endow poly(ester amide)s with higher upper temperature limits and better heat resistances. Post-modified poly(ester amide)s with tunable Tg exhibited in this study will further expand the processing window and application field of these polymeric materials.
Preparation of a PEA-based reversible cross-linked network
Retro-Diels–Alder reactions between dienes and dienophiles are an efficient strategy for reversible cross-linked network formation.50,51 To illustrate the potential applications of our modified PEAs, a PA-1E-based reversible network was prepared via a retro-Diels–Alder reaction. 1,6-Bis(maleimido)hexane (1,6-BMH, Fig. S8†) was added into a PA-1E DMF solution to form a reaction mixture. At room temperature, the viscosity of the mixture progressively increased and within 5 min the medium stopped as the solution gelled. The gel was then heated to 100 °C at which point the mixture became free flowing again with the viscosity becoming like the initial solution after 5 minutes. As shown in Fig. 3, reverse gelation in a covalently cross-linked Diels–Alder poly(ester amide) network was observed by simply changing the ambient temperature. This versatile click–unclick feature undoubtedly contributes to reshaping and reprocessing of the PEAs-based organogel.
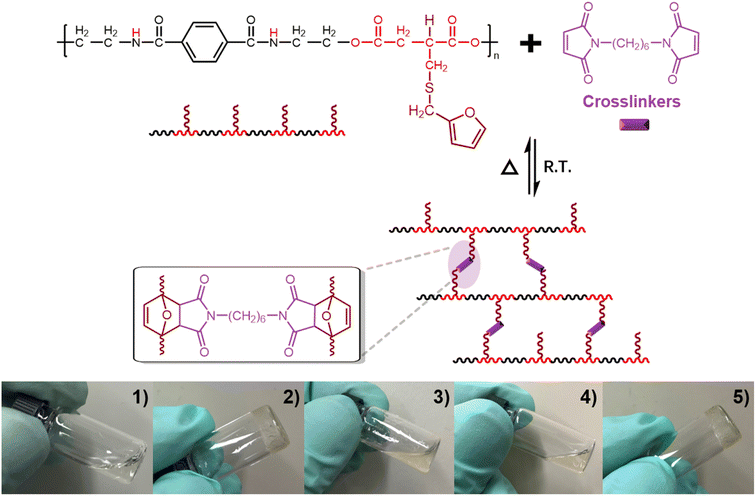 |
| Fig. 3 Top: the retro-Diels–Alder reaction (rDA) based on PA-1E and 1,6-bis(maleimido)hexane (1,6-BMH) for reversible cross-linked polyamide organogel formation; bottom: digital photos of (1) PA-1E in DMF; (2) after added 1,6-BMH for 5 min at room temperature; (3) after heated for 1 min at 100 °C; (4) continue heated for 4 min at 100 °C; (5) cooling to room temperature. | |
Preparation of a PEA-based polyurethane film
Polymers bearing pendant hydroxyl groups are versatile platforms to produce value-added polymeric materials for application in biomedicine, packaging, and others via post-polymerization modification.52–55 Polyurethane PU was synthesized via reacting PA-1C and 4,4′-methylenediphenyl diisocyanate (4,4′-MDI) to demonstrate high reactivity of these bio-based hydroxyl-containing poly(ester amide)s (or polyols). The formation of PU occurred in two steps: firstly, PA-1C was dissolved in a small amount of DMF, followed by mixing 4,4′-MDI (–NCO
:
–OH equal to 1
:
1); secondly, the mixture was heated at 120 °C for 30 min to cure. Then DMF was removed under vacuum to afford PU as a colorless film. The PU film was analyzed by FT-IR to confirm the formation of urethane groups. Fig. 4 shown FT-IR spectra for (a) PA-1C (b) 4,4′-MDI and (c) polyurethane (PU) produced by PA-1C and 4,4′-MDI. The PU spectrum (Fig. 4c) presented the characteristic bands: the stretching vibration of carbonyl of the carbamate (NCOO) at 1700 cm−1, the deformation vibration of N–H at 1528 cm−1, asymmetric stretching of C–O–C at 1219 cm−1 and the symmetric stretching of C–O–C at 1075 cm−1. One broad signal between 3200 to 3600 cm−1 is attributed to both stretching vibration of O–H and N–H; this was due to the formation of urethane groups and the remaining –OH groups from PA-1C. Other signals corresponding to PA-1C (Fig. 4a) were also observed in the PU spectrum, as alkyl C–H stretching at 2850–2950 cm−1, and carbonyl ester at 1726 cm−1. Meanwhile, the representative isocyanate vibration attributed to 4,4′-MDI at 2200 cm−1 (Fig. 4b) disappeared in the PU spectrum. The FT-IR results indicated that bio-based hydroxyl-containing PA-1C synthesized by post-modification of PA-1 is an appropriate candidate for further reactions like polyurethane (PU) production.
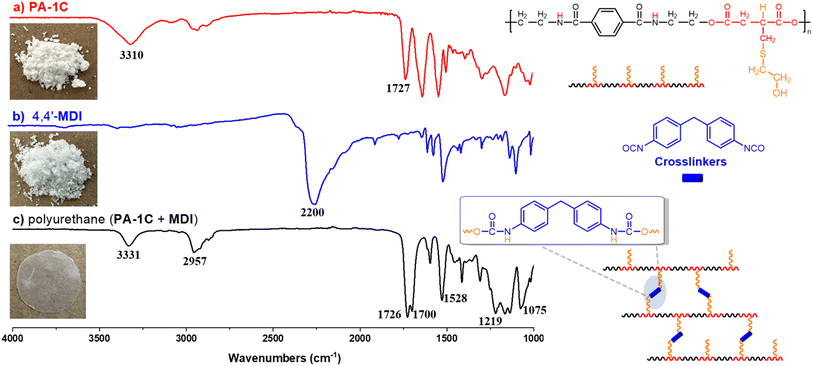 |
| Fig. 4 Comparison of FT-IR spectrum of (a) PA-1C; (b) 4,4′-methylenediphenyl diisocyanate (4,4′-MDI) and (c) polyurethane (PU) produced by a reaction between 4,4′-MDI and PA-1C. The characteristic peaks of the polymers were labeled in the FT-IR spectra. | |
Conclusions
In conclusion, we have demonstrated a range of synthetic opportunities through the thiol–ene click coupling of bio-derived unsaturated poly(ester amide)s with different thiol reagents. Various pendant functional groups, including alkyl, aryl, hydroxyl, carboxyl, furyl, and sulfonate were successfully incorporated into PEAs backbones for thermal behavior adjustment, thermal reprocessable networks production, and polyurethane film synthesis. Thanks to the simple procedures of the thiol–ene click reaction, all conjugation products were made in good yield. Improved thermal stability and tunable glass transition temperature were observed for modified PEAs compared to the parent, pre-thiol–ene PEA. This ability to routinely prepare functionalized linear PEAs represents an advance compared to traditional approaches and is further evidence of the synthetic utility of click reactions in materials chemistry.
Conflicts of interest
There are no conflicts to declare.
Acknowledgements
We thank the National Science Foundation (EFMA-2132133) and the University of Alabama for financial support. We thank the NSF MRI program (CHE1919906) for the purchase of an NMR spectrometer.
References
- M. Winnacker and B. Rieger, Poly(ester amide)s: recent insights into synthesis, stability and biomedical applications, Polym. Chem., 2016, 7(46), 7039–7046 RSC.
- A. C. Fonseca, M. H. Gil and P. N. Simões, Biodegradable poly(ester amide)s – A remarkable opportunity for the biomedical area: Review on the synthesis, characterization and applications, Prog. Polym. Sci., 2014, 39(7), 1291–1311 CrossRef CAS.
- H. Sun, F. Meng, A. A. Dias, M. Hendriks, J. Feijen and Z. Zhong, α-Amino Acid Containing Degradable Polymers as Functional Biomaterials: Rational Design, Synthetic Pathway, and Biomedical Applications, Biomacromolecules, 2011, 12(6), 1937–1955 CrossRef CAS PubMed.
- J. Wu, D. Wu, M. A. Mutschler and C.-C. Chu, Cationic Hybrid Hydrogels from Amino-Acid-Based Poly(Ester Amide): Fabrication, Characterization, and Biological Properties, Adv. Funct. Mater., 2012, 22(18), 3815–3823 CrossRef CAS.
- S. Han and J. Wu, Recent Advances of Poly(Ester Amide)s-Based Biomaterials, Biomacromolecules, 2022, 23(5), 1892–1919 CrossRef CAS PubMed.
- Y.-T. Guo, W. Xiong, C. Shi, F.-S. Du and Z.-C. Li, Facile synthesis of eight-membered cyclic(ester-amide)s and their organocatalytic ring-opening polymerizations, Polym. Chem., 2022, 13(31), 4490–4501 RSC.
- D. Maniar, F. Silvianti, V. M. Ospina, A. J. J. Woortman, J. van Dijken and K. Loos, On the way to greener furanic-aliphatic poly(ester amide)s: Enzymatic polymerization in ionic liquid, Polymer, 2020, 205, 122662 CrossRef CAS.
- J. Lustoň, J. Kronek, O. Markus, I. Janigová and F. Böhme, Synthesis and polymerization reactions of cyclic imino ethers. 3. Poly(ester amide)s of the AA + BB type on the basis of 2-oxazolines, Polym. Adv. Technol., 2007, 18(2), 165–172 CrossRef.
- T. Gleede, L. Reisman, E. Rieger, P. C. Mbarushimana, P. A. Rupar and F. R. Wurm, Aziridines and azetidines: building blocks for polyamines by anionic and cationic ring-opening polymerization, Polym. Chem., 2019, 10(24), 3257–3283 RSC.
- C. Giri, S. E. Sisk, L. Reisman, I. Kammakakam, J. E. Bara, K. N. West, B. D. Rabideau and P. A. Rupar, Anionic Ring-Opening Polymerizations of N-Sulfonylaziridines in Ionic Liquids, Macromolecules, 2022, 55(2), 623–629 CrossRef CAS.
- C. Giri, J.-Y. Chang, P. C. Mbarushimana and P. A. Rupar, The Anionic Polymerization of a tert-Butyl-Carboxylate-Activated Aziridine, Polymers, 2022, 14(16), 3253 CrossRef CAS PubMed.
- S. Kobayashi, Ethylenimine polymers, Prog. Polym. Sci., 1990, 15(5), 751–823 CrossRef CAS.
- M. Jäger, S. Schubert, S. Ochrimenko, D. Fischer and U. S. Schubert, Branched and linear poly(ethylene imine)-based conjugates: synthetic modification, characterization, and application, Chem. Soc. Rev., 2012, 41(13), 4755–4767 RSC.
- M. L. Sarazen and C. W. Jones, Insights into Azetidine Polymerization for the Preparation of Poly(Propylenimine)-Based CO2 Adsorbents, Macromolecules, 2017, 50(23), 9135–9143 CrossRef CAS.
- I. C. Stewart, C. C. Lee, R. G. Bergman and F. D. Toste, Living Ring-Opening Polymerization of N-Sulfonylaziridines: Synthesis of High Molecular Weight Linear Polyamines, J. Am. Chem. Soc., 2005, 127(50), 17616–17617 CrossRef CAS PubMed.
- J. Xu and N. Hadjichristidis, Well-Defined Poly(Ester Amide)-Based Homo- and Block Copolymers by One-Pot Organocatalytic Anionic Ring-Opening Copolymerization of N-Sulfonyl Aziridines and Cyclic Anhydrides, Angew. Chem., Int. Ed., 2021, 60(13), 6949–6954 CrossRef CAS PubMed.
- J. Xu, X. Wang and N. Hadjichristidis, Diblock dialternating terpolymers by one-step/one-pot highly selective organocatalytic multimonomer polymerization, Nat. Commun., 2021, 12(1), 7124 CrossRef CAS PubMed.
- J. Xu, P. Zhang, Y. Yuan and N. Hadjichristidis, Elucidation of the Alternating Copolymerization Mechanism of Epoxides or Aziridines with Cyclic Anhydrides in the Presence of Halide Salts, Angew. Chem., Int. Ed., 2023, 62(14), e202218891 CrossRef CAS PubMed.
- R. Yang, Y. Wang, W. Luo, Y. Jin, Z. Zhang, C. Wu and N. Hadjichristidis, Carboxylic Acid Initiated Organocatalytic Ring-Opening Polymerization of N-Sulfonyl Aziridines: An Easy Access to Well-Controlled Polyaziridine-Based Architectural and Functionalized Polymers, Macromolecules, 2019, 52(22), 8793–8802 CrossRef CAS.
- T. Qu and P. A. Rupar, Polymerizations of 2-(Trimethylsilyl)ethanesulfonyl-activated aziridines, Eur. Polym. J., 2022, 169, 111135 CrossRef CAS.
- T. Gleede, E. Rieger, J. Blankenburg, K. Klein and F. R. Wurm, Fast Access to Amphiphilic Multiblock Architectures by the Anionic Copolymerization of Aziridines and Ethylene Oxide, J. Am. Chem. Soc., 2018, 140(41), 13407–13412 CrossRef CAS.
- C. Bakkali-Hassani, C. Coutouly, T. Gleede, J. Vignolle, F. R. Wurm, S. Carlotti and D. Taton, Selective Initiation from Unprotected Aminoalcohols for the N-Heterocyclic Carbene-Organocatalyzed Ring-Opening Polymerization of 2-Methyl-N-tosyl Aziridine: Telechelic and Block Copolymer Synthesis, Macromolecules, 2018, 51(7), 2533–2541 CrossRef CAS.
- X. Wang, Y. Liu, Z. Li, H. Wang, H. Gebru, S. Chen, H. Zhu, F. Wei and K. Guo, Organocatalyzed Anionic Ring-Opening Polymerizations of N-Sulfonyl Aziridines with Organic Superbases, ACS Macro Lett., 2017, 6(12), 1331–1336 CrossRef CAS PubMed.
- H. Wang, J. Li, Z. Li, B. Liu, K. Chen, Z. Zhang, Y. Hu, F. Zhou, Y. Li and K. Guo, Tetrabutylammonium fluoride initiated anionic ring-opening polymerizations of N-sulfonyl aziridines, Eur. Polym. J., 2020, 140, 109999 CrossRef CAS.
- S. Kang, H. K. Moon and H. J. Yoon, Diaziridyl Ether of Bisphenol A, Macromolecules, 2018, 51(11), 4068–4076 CrossRef CAS.
- L. Zhu, H. Huang, Y. Wang, Z. Zhang and N. Hadjichristidis, Organocatalytic Synthesis of Polysulfonamides with Well-Defined Linear and Brush Architectures from a Designed/Synthesized Bis(N-sulfonyl aziridine), Macromolecules, 2021, 54(17), 8164–8172 CrossRef CAS.
- Z. Zhou, Y. Wang, L. Zhu, D. Dang and Z. Zhang, Tributylphosphine-catalyzed aziridine-based cycloaddition polymerization toward thiacyclic polymers, Polym. Chem., 2022, 13(33), 4809–4816 RSC.
- S. Chen, L. Zhu and Z. Zhang, Catalyst-free aziridine-based step-growth polymerization: a facile approach to optically active poly(sulfonamide amine)s and poly(sulfonamide dithiocarbamate)s, Polym. Chem., 2022, 13(29), 4324–4332 RSC.
- Q. Chen, J. Ye, L. Zhu, J. Luo, X. Cao and Z. Zhang, Organocatalytic multicomponent polymerization of bis(aziridine)s, diols, and tosyl isocyanate toward poly(sulfonamide urethane)s, Eur. Polym. J., 2022, 180, 111585 CrossRef CAS.
- T. Qu and P. A. Rupar, Carbonyl Aziridines: Strained Amides for Rapid Polyamide Synthesis, Macromolecules, 2022, 55(21), 9513–9519 CrossRef CAS.
- K. Parkatzidis, H. S. Wang, N. P. Truong and A. Anastasaki, Recent Developments and Future Challenges in Controlled Radical Polymerization: A 2020 Update, Chem, 2020, 6(7), 1575–1588 CAS.
- J. Gopinathan and I. Noh, Click Chemistry-Based Injectable Hydrogels and Bioprinting Inks for Tissue Engineering Applications, Tissue Eng. Regener. Med., 2018, 15(5), 531–546 CrossRef CAS.
- X. Wang, Y. Ding, Y. Tao, Z. Wang, Z. Wang and J. Yan, Polycondensation of bis(α-diazo-1,3-dicarbonyl) compounds with dicarboxylic acids: an efficient access to functionalized alternating polyesters, Polym. Chem., 2020, 11(10), 1708–1712 RSC.
- K. A. Günay, P. Theato and H.-A. Klok, Standing on the shoulders of Hermann Staudinger: Post-polymerization modification from past to present, J. Polym. Sci., Part A: Polym. Chem., 2013, 51(1), 1–28 CrossRef.
- X.-X. Deng, L. Li, Z.-L. Li, A. Lv, F.-S. Du and Z.-C. Li, Sequence Regulated Poly(ester-amide)s Based on Passerini Reaction, ACS Macro Lett., 2012, 1(11), 1300–1303 CrossRef CAS.
- X.-W. Kan, X.-X. Deng, F.-S. Du and Z.-C. Li, Concurrent Oxidation of Alcohols and the Passerini Three-Component Polymerization for the Synthesis of Functional Poly(ester amide)s, Macromol. Chem. Phys., 2014, 215(22), 2221–2228 CrossRef CAS.
- O. Ikkala and G. ten Brinke, Functional Materials Based on Self-Assembly of Polymeric Supramolecules, Science, 2002, 295(5564), 2407–2409 CrossRef CAS PubMed.
- F. Biedermann, E. A. Appel, J. del Barrio, T. Gruendling, C. Barner-Kowollik and O. A. Scherman, Postpolymerization Modification of Hydroxyl-Functionalized Polymers with Isocyanates, Macromolecules, 2011, 44(12), 4828–4835 CrossRef CAS.
- O. Ivasenko and D. F. Perepichka, Mastering fundamentals of supramolecular design with carboxylic acids. Common lessons from X-ray crystallography and scanning tunneling microscopy, Chem. Soc. Rev., 2011, 40(1), 191–206 RSC.
- T. N. Gevrek and A. Sanyal, Furan-containing polymeric Materials: Harnessing the Diels-Alder chemistry for biomedical applications, Eur. Polym. J., 2021, 153, 110514 CrossRef CAS.
- D. Chen, C. Cui, N. Tong, H. Zhou, X. Wang and R. Wang, Water-Soluble and Low-Toxic Ionic Polymer Dots as Invisible Security Ink for MultiStage Information Encryption, ACS Appl. Mater. Interfaces, 2019, 11(1), 1480–1486 CrossRef CAS PubMed.
- Y. Lin, J.-W. Gao, H.-W. Liu and Y.-S. Li, Synthesis and Characterization of Hyperbranched Poly(ether amide)s with Thermoresponsive Property and Unexpected Strong Blue Photoluminescence, Macromolecules, 2009, 42(9), 3237–3246 CrossRef CAS.
- X. Pang, J. Wu, C. Reinhart-King and C.-C. Chu, Synthesis and characterization of functionalized water soluble cationic poly(ester amide)s, J. Polym. Sci., Part A: Polym. Chem., 2010, 48(17), 3758–3766 CrossRef CAS.
- E. Esen, P. Hädinger and M. A. R. Meier, Sustainable Fatty Acid Modification of Cellulose in a CO2-Based Switchable Solvent and Subsequent Thiol-Ene Modification, Biomacromolecules, 2021, 22(2), 586–593 CrossRef CAS PubMed.
- E. A. Campo, 3 - Thermal Properties of Polymeric Materials, in Selection of Polymeric Materials, ed. Campo, E. A., William Andrew Publishing, Norwich, NY, 2008, pp 103–140 Search PubMed.
- J. Antoniou, F. Liu, H. Majeed, H. J. Qazi and F. Zhong, Physicochemical and thermomechanical characterization of tara gum edible films: Effect of polyols as plasticizers, Carbohydr. Polym., 2014, 111, 359–365 CrossRef CAS PubMed.
- M. Rico, S. Rodríguez-Llamazares, L. Barral, R. Bouza and B. Montero, Processing and characterization of polyols plasticized-starch reinforced with microcrystalline cellulose, Carbohydr. Polym., 2016, 149, 83–93 CrossRef CAS PubMed.
- X. Ma, C. Qiao, X. Wang, J. Yao and J. Xu, Structural characterization and properties of polyols plasticized chitosan films, Int. J. Biol. Macromol., 2019, 135, 240–245 CrossRef CAS PubMed.
- G. L. Baker, E. B. Vogel and M. R. Smith, Glass Transitions in Polylactides, Polym. Rev., 2008, 48(1), 64–84 CrossRef CAS.
- T. Dispinar, R. Sanyal and A. Sanyal, A Diels-Alder/retro Diels-Alder strategy to synthesize polymers bearing maleimide side chains, J. Polym. Sci., Part A: Polym. Chem., 2007, 45(20), 4545–4551 CrossRef CAS.
- J. M. Rinehart, J. R. Reynolds and S. K. Yee, Limitations of Diels–Alder Dynamic Covalent Networks as Thermal Conductivity Switches, ACS Appl. Polym. Mater., 2022, 4(2), 1218–1224 CrossRef CAS.
- Y. Yu and Y. Chau, One-Step “Click” Method for Generating Vinyl Sulfone Groups on Hydroxyl-Containing Water-Soluble Polymers, Biomacromolecules, 2012, 13(3), 937–942 CrossRef CAS PubMed.
- Y. Xia, D. Zhang, Z. Li, H. Lin, X. Chen, S. Oliver, S. Shi and L. Lei, Toughness modification of cationic UV-cured cycloaliphatic epoxy resin by hydroxyl polymers with different structures, Eur. Polym. J., 2020, 127, 109594 CrossRef CAS.
- H. Zhou, Y. Chen, C. M. Plummer, H. Huang and Y. Chen, Facile and efficient bromination of hydroxyl-containing polymers to synthesize well-defined brominated polymers, Polym. Chem., 2017, 8(14), 2189–2196 RSC.
- P. Alagi, Y. J. Choi and S. C. Hong, Preparation of vegetable oil-based polyols with controlled hydroxyl functionalities for thermoplastic polyurethane, Eur. Polym. J., 2016, 78, 46–60 CrossRef CAS.
|
This journal is © The Royal Society of Chemistry 2023 |
Click here to see how this site uses Cookies. View our privacy policy here.