DOI:
10.1039/D3RA03381C
(Paper)
RSC Adv., 2023,
13, 19164-19172
Psidium guajava leaf extract mediated green synthesis of silver nanoparticles and its application in antibacterial coatings
Received
21st May 2023
, Accepted 20th June 2023
First published on 23rd June 2023
Abstract
In this study, Psidium guajava (P. guajava) leaf extract-assisted silver nanoparticles (AgNPs) were synthesized and their antibacterial activities were investigated. The synthesized green AgNPs were characterized by various analytical techniques including UV-Vis spectroscopy, Fourier transform infrared spectroscopy (FTIR), X-ray diffractometry (XRD), field emission scanning electron microscopy (FE-SEM), energy dispersive X-ray (EDX) spectroscopy, etc. From the UV-Vis spectroscopic analysis, the formation of nanoparticles has been confirmed by the color change from light yellow to reddish brown of the solution due to the excitation of the surface plasmon resonance peak at 430 nm. In addition, the FTIR study showed the reduction of Ag ions owing to the presence of biomolecules in the leaf extract, which acted as reducing as well as capping agents. Furthermore, XRD analysis reveals the identified 2θ peaks of AgNPs at ∼39° with cubic structure. The FE-SEM micrograph illustrated the material was formed in nano-dimensions, with an average particle size of ∼12 nm and almost spherical in shape. Moreover, P. guajava-mediated AgNPs demonstrated good antibacterial activity against both Gram-positive (S. aureus) and Gram-negative (E. coli) bacterial strains. The synthesis was performed by a bio-reduction process where a bioactive agent is responsible for reducing metallic ions to metallic nanoparticles as an eco-friendly, cost-effective, non-toxic, one-step, and sustainable method. Therefore, this study may create an imperative synthetic route for the fabrication of green-AgNPs and their application in antibacterial coatings in cotton textiles.
1. Introduction
Nanotechnology is the most significant approach in modern scientific practice in the world. Researchers have made extensive development of electronics, environment and human health in medical science through nanotechnology.1 The application of nanoparticles (NPs) in nanotechnology is increasing steadily.2 To meet the demand, different synthesis methods are being created over time. Conventional (chemical or physical) methods are used as a complex method of making NPs which is harmful to the environment and human health due to chemical hazards and reaction contamination.3,4 At the same time, conventional methods for synthesis of NPs are time-consuming, expensive, and toxic when handling.5 On the other hand, green synthesis is eco-friendly and sustainable innovative method because various elements used here which come from organic materials.6 Green synthesis of NPs is time saving and cost-effective bio-synthesis, with less chemical hazard. Metallic or oxide NPs, both are highly potential. This synthesis technique uses various elements which come from organic materials.7–9
Generally, wood and bones are used to produce NPs but in the green approach plants are popular more than others. Plants element likes bulks, roots, fruits and leaves are used typically to synthesize NPs.10 Moreover, extract of green leaves of various medicinal plants are used as they have significant reducing and capping agents to convert metallic ions to form NPs. Azadirachta indica (neem), Moringa oleifera, Justicia adhatoda,11 Aloe vera and Psidium guajava (P. guajava) etc. are common medicinal plants used for green synthesis of NPs. Among them, extract of P. guajava (guava) is used mostly because it contains glycosides and polyphenolics (flavonoids and tennies) which act as reducing and capping agents to convert metallic ions to NPs.12–14 P. guajava, commonly known as guava, a popular fruit in Bangladesh. This evergreen tree is found throughout Asia and is widely cultivated in India, Bangladesh, and Malaysia.
Synthesis of various NPs such as Au, Cu, Ni, Al including Ag can be attained from guava leaf extract.10,15,16 Several researchers have taken attempts to synthesize metallic nanoparticles exhausting aqueous extract of P. guajava leaves, especially silver nanoparticles considering wide range of advantages of green synthesis. Silver nitrate (AgNO3) was used as a precursor and most of the research used (1–30) mM solution of silver ions reduced by different percentages of aqueous extract of P. guajava leaves. In the synthesis process silver salts, Ag+ converts to metallic silver, Ag0 through biological molecules of the leaf extract which may contain reducing agents as well as metal salt reducers. P. guajava leaf extract contains biochemicals such as polyphenols, carotenoids, terpenoids, flavonoids, tannins and triterpenes could be responsible for the reduction of metal ions to NPs.9,10 Bose et al. prepared AgNPs using P. guajava and investigate their anti-bacterial activity against Gram negative bacterial strain.9 Sharmila et al.17 demonstrates result for both Gram-negative and Gram-positive bacteria with zone of inhibition less than 10 mm. Geetha et al. also get <10 mm inhibition zone by analyzing five pathogens for AgNP solutions.18 Besides, Dama et al. prepared spherical AgNPs using methanol solvent and analyzed anti-microbial efficacy against E. coli, S. aureus, and P. aeruginosa microbes.19 AgNPs has been applied in various fields such as engineering, communication, photochemical, bio-application, packaging antibacterial protection, etc. In addition, it has applications in biomedical devices, environmental remediation, medicine, food packaging, cosmetics, and other industrial purposes.15
AgNPs have distinct composition and structure, crystallinity, shape and size compared to the bulk forms. Consequently, it exhibited excellent physicochemical properties for instance chemical stability, surface-enhanced Raman scattering, high conductivity both thermal and electrical, catalytic activity, non-linear optical behavior, and biological properties compare to others NPs.16 AgNPs possess a wide-ranging spectrum of anti-bacterial and anti-fungal activities14 generally applied to various consumer products for example cosmetics, toiletries, plastics, food, and textiles.20
The objective of this research is to synthesize eco-friendly AgNPs from P. guajava (myrtle family)12 leaf extract and to examine its antibacterial activity. Here, synthesized AgNPs solutions with different concentrations of AgNO3 were observed against Gram-negative bacteria to examine antibacterial activity. Finally, textile cotton and N95 mask fibers were coated with leaf extract-mediated Ag nanoparticles and analyzed the anti-bacterial activity by applying Gram-positive and Gram-negative bacteria separately for application in cotton textiles as an antibacterial coating. Moreover there is a lake of availability of textile industries based on AgNPs base research.
2. Experimental methods
2.1 Materials
In this research work, healthy and fresh leaves of P. guajava plant were collected from the tree, grown in front of BCSIR Dhaka Laboratories, Bangladesh. The tree was medium-sized; has leaves of 7–10 cm in length and 4–5 cm in width and remains green throughout the year. Analytical grade silver nitrate (AgNO3) and sodium hydroxide (NaOH) were purchased from Merck, Germany (98% purity). Textile cotton and N95 mask fibers were obtained from local market of Bangladesh. For the preparation of leaf extract deionized water and Whatman no. 1 filter papers were used for erosion and filtration purpose. Moreover, for cleaning glassware (pipette, beaker, etc.) ethanol and distilled water were applied. Bacterial clinical isolations were generated by the Institute of National Analytical Research and Service (INARS), BCSIR.
2.2 Preparation of P. guajava leaf extract
For the preparation of leaf extract, fresh green leaves of P. guajava were taken, separated from the petiole and midrib, and washed with tap water thoroughly. The leaves were then washed further for several times to remove dust and surface contaminants using deionized water and hung for drying at room temperature (28 °C) for 1 hour. The dried leaves were sliced and cut into fine pieces using scissors. For preparing each batch of extract, 10 g of fine pieces of leaves were taken in a beaker with 100 mL of deionized water. The beaker was placed in a hot plate and stirred constantly at 400 rpm at 90 °C for 25 minutes to get leaf extract. This extract was filtered through Whatman filter paper for two times and kept at room temperature. Finally, the prepared aqueous leaf extract was poured into AgNO3 solution in different concentrations. The rest of the extract was kept in the refrigerator at 4 °C covered by aluminum foil for further use.
2.3 Biosynthesis of silver nanoparticles
To synthesize silver nanoparticles, aqueous leaf extracts (1 mL of P. guajava) and 10 mL of AgNO3 solution in different concentrations (10, 15, 20 and 25 mM) were taken in a beaker. The solution was placed in a hot plate; the temperature of the solution/mixture was raised to ∼60 °C maintaining constant magnetic stirring for 10 minutes. Afterward, it was kept at room temperature for 2 hours where the reduction of Ag+ ions to metallic Ag conversion was taken place. Finally, the solution was observed carefully, and without any pulsation, the color of the solution changed from light yellow to reddish brown which specifies the formation of AgNPs initially. A small portion of colored suspension was centrifuged at 10
000 rpm for 15 minutes to get powder material. Collected materials were washed with deionized water for several times to remove any impurities. It was then taken in a Petri dish and kept in an electric oven at 60 °C for 2 hours for drying. The dried powders were then annelid at 500 °C for 2 hours and stored in a desiccator for further use. Fig. 1 shows the whole procedure of synthesis of P. guajava leaf extract-mediated AgNPs.
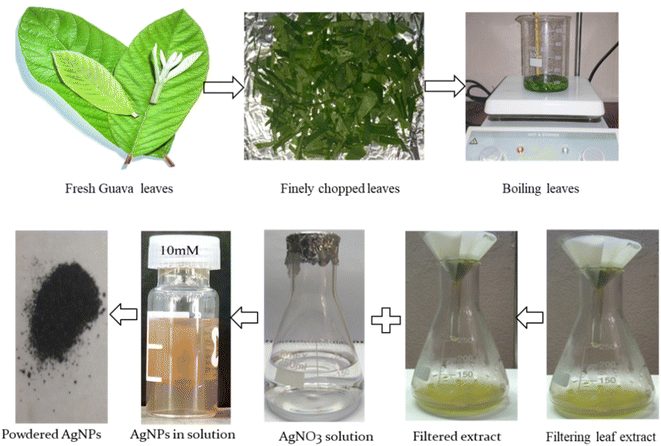 |
| Fig. 1 Schematic representation for the synthesis of P. guajava leaf extract mediated AgNPs. | |
2.4 Treatment of cotton and mask fibers
Cotton fiber from the local market may have dust, oil, hemicellulose and organic acids on its surface, so treatment is necessary to get anti-microbial cotton. At first, the cotton was washed with detergent to remove dust. Afterward, it was soaked in a solution of ammonia and ethanol (4
:
6) for 10 hours in a dark room for oil removal. For alkali treatment, the fibers were washed thoroughly and dried in a vacuum oven at 40 °C for 24 hours. A 1 g cotton fiber was taken in a beaker and 30 mL NaOH solution was added so that the fiber emerged in the solution completely. The beaker was placed in a magnetic stirrer at 60 °C for 1 hour maintaining continuous stirring. Later, the cotton fiber was pulled out from the solution and washed three times before drying in the oven at 40 °C. However, fresh/new N95 mask fibers do not require any treatment and they were used as it is. Both cotton and N95 mask fibers were then treated by low-temperature RF oxygen plasma (10 min, 0.13 Torr, power input 100 W, RF-13.5 MHz) which was necessary to incorporate AgNPs into the fiber. Plasma modification generates active species on fiber surfaces thus it may used as a medium of antimicrobial agents.11
2.5 Preparation of AgNPs/cotton and AgNPs/N95 mask coating
A 1 g of plasma treated cotton fiber was dipped in 30 mL of aqueous solutions of synthesized P. guajava leaf extract-mediated AgNPs solution (10 mM). Similarly, 1 g of N95 mask fiber was soaked in 30 mL of aqueous solutions of synthesized AgNPs taken in another beaker. The beakers are then placed in a magnetic stirrer and stirred for 24 hours continually to get coated AgNPs/cotton and AgNPs/N95 mask. AgNPs can be incorporated homogeneously into the surface of the fibers because it becomes hydrophilic after plasma treatment. Finally, coated fibers were washed out with distilled water thoroughly to take out any residue and dried at 40 °C. The dried AgNPs/cotton and AgNPs/N95 masks were then reserved in a desecrator.
2.6 Instrumentation
The characteristic of synthesized AgNPs and coated fibers (cotton and mask) were investigated by different characterization techniques. The bioreduction of AgNO3 in aqueous solution was observed with mixed plant extracts studies by using ultraviolet-visible near-infrared (UV-Vis-NIR) spectrophotometer (UV-2600, Shimadzu, Japan) ranging 200–800 nm. X-ray diffraction (XRD) (Rigaku Smart Lab, Japan) with Cu-Kα radiation (λ = 1.5406 Å) was used to confirm the crystallinity of AgNPs at room temperature. The scanning rate was 10° min−1; power 40 kV at 40 mA and 0.02° step. A field emission scanning electron microscope (FE-SEM) (JSM-7610F, Japan) and energy dispersive X-ray (EDX) were used to study the surface morphology of AgNPs, AgNPs/cotton and AgNPs/mask composite and to identify the elemental composition of materials. Highly pure KBr powder was mixed with 1% (w/w) samples and then pressed into pellets to identify the bioactive functional group of AgNPs by Fourier transform infrared spectroscopy (FTIR, PerkinElmer, USA) recorded within 4000–350 cm−1.
3. Results and discussion
3.1 Characterization of AgNPs
AgNPs have unique optical properties that help them to interact strongly with specific wavelengths of light.21 To identify the formation of silver nanoparticles primarily the color of the solution was observed. After mixing the leaf extract with AgNO3 for 2 two hours, due to surface plasmon resonance (SPR) the soft yellowish color of the solution has changed to brownish color which confirms the presence of AgNPs. Fig. 2 shows the color change observation of AgNPs solutions, the colors gave similar result with other previous research.15 For further confirmation of the formation of NPs UV-Vis spectroscopic analysis was accomplished.
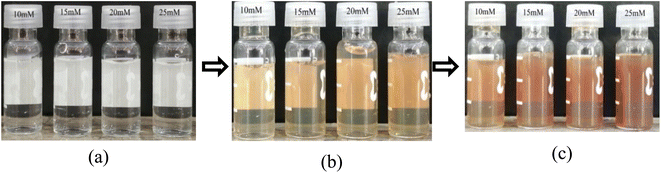 |
| Fig. 2 Observation of color changes during synthesis of Ag NPs at different time intervals: (a) at the beginning (0.0 minutes), (b) after 60 minutes, and (c) after 120 minutes. | |
3.2 UV-vis spectroscopic analysis
UV-Vis spectroscopy is a firm, modest, sensitive and selective method used to identify different types of NPs initially. Moreover, no calibration is required to determine the particulate properties of the colloidal suspension.22,23 Fig. 3(a) shows the UV-Vis absorption spectra of P. guajava leaf extract mediated AgNPs solution at wavelength 200–800 nm. The baseline data was taken using deionized water which is also referred as sample blank in this examination. The colored samples were diluted with deionized water and taken in the quartz cell of UV spectroscopy and the absorption peaks were found in the ultraviolet region at ∼350–530 nm. The absorption peaks were determined at different concentrations of AgNO3 salt and showed in Fig. 3(a). The intensity of absorption peaks increased with concentration however no shift change compared to leaf extract was observed.
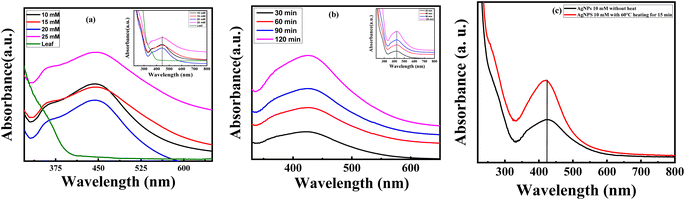 |
| Fig. 3 UV-Vis absorption spectra of AgNPs (a) at different concentrations of AgNO3 solution (b) at different time intervals for 10 mM AgNO3 solution and (c) at different temperatures for 10 mM AgNO3 solution. | |
In Fig. 3(b) and (c), the effect of time and temperature variation for 10 mM AgNO3 solution was illustrated. It revealed that the absorption in the visible region is gradually increased with respect to time and temperature. From Fig. 3(b) the absorption intensity was red-shifted due to an increase in particle size with time interval which was confirmed by SPR intensity with help of the % reduction of Ag+ to Ag0. In addition, an increase in temperature favored the formation of leaf extract-mediated AgNPs and the wider peak of absorption indicates stable condition in Fig. 3(c). The free electron between the conduction band and the valence band moves and generate a range of absorption peak because of the mass oscillation of the electrons of the silver nanoparticles in the resonance with the optical wave at SPR.24,25 The particle sizes, and surrounding chemical and electrical insulation are the main criteria of absorption of AgNPs.26,27
3.3 X-ray diffraction analysis
The structural properties of particles were analyzed by a conversant technique, XRD to get information about crystallinity and phase formation. The XRD pattern with reflections at different angular positions of the synthesized AgNPs was shown in Fig. 4. The Bragg's peaks were observed at 2θ positions 32.99°, 39.21°, 44.39, 64.43° and 77.37° for (101), (111), (200), (220) and (311) crystal planes respectively. The formation of AgNPs were confirmed further by these characterization reflections. Also, the diffraction peaks of the XRD pattern correspond to the card (JCPDS No. 03-0921) which reflects those NPs are crystalline in nature.28 The XRD pattern has confirmed that the synthesized samples were AgNPs with face-centered cubic crystal structure.29
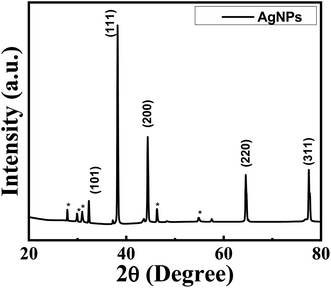 |
| Fig. 4 XRD patterns of synthesized leaf extract mediated AgNPs. | |
Along with the characteristic peaks in the XRD pattern some * marked reflections also exist at 2θ (27.90°, 30.01°, 31.06, 46.34 and 54.97°) which were not known precisely. It may be due to the crystallization of bioorganic phases on the AgNPs surface that originates from the leaf extract30 or due to AgNO3 that was not reduced and remained in the sample slightly.29 Many researchers also get similar patterns in XRD.16–18
The average crystallite size, D of the synthesized AgNPs were estimated from the diffractogram by using the Debye–Scherrer formula, D = 0.9λ/β
cos
θ, where λ and β are the wavelength of diffraction and full width at half maximum (FWHM) of a peak respectively.31 The interplanar spacing between the atoms, d, was calculated using Bragg's law, and lattice constant, a, was determined.32 The values of lattice parameters, d-spacing, FWHM, hkl value, and crystallite size at different angular position were demonstrated in Table 1.
Table 1 Structural parameters of synthesized leaf extract-mediated AgNPs
2θ (degree) |
FWHM degree |
Interplaner spacing, ‘d’ Å |
h k l |
Crystallite size ‘D’ nm |
Lattice constant, ‘a’ Å |
32.99 |
0.1538 |
2.7693 |
1 0 1 |
19.84 |
4.0315 |
39.21 |
0.148 |
2.3533 |
1 1 1 |
20.54 |
4.0956 |
44.39 |
0.1622 |
2.0462 |
2 0 0 |
20.96 |
4.0924 |
64.43 |
0.3582 |
1.4449 |
2 2 0 |
26.23 |
4.0868 |
77.37 |
0.5117 |
1.2329 |
3 1 1 |
19.90 |
4.0891 |
From Table 1, it is seen that the D values are nearly identical for all the peaks and the average crystallite size obtained was ∼22 nm. Thus, the XRD analysis of silver nanoparticles showed well-defined dimensions has fair agreement with the standard value for silver. Moreover, the lattice parameters, d-spacing, and hkl value calculated from the characteristic peaks of the synthesized sample match with reports of other researchers.10,15
3.4 Field emission scanning electron microscopy (FE-SEM) analysis
The surface morphology of guava leaf extract-mediated AgNPs were investigated by FE-SEM and illustrated in Fig. 5(a)–(d). The FE-SEM images showed a spherical shape for all the concentrations of AgNO3 solutions. Fig. 5(d) showed distinct and homogeneous grain distribution; however, (10, 15, and 20 mM) AgNO3 solution contains some agglomeration in Fig. 5(a)–(c). Similar shape was found for ref. 33. The average grain size estimated in the range 12–19 nm for the variation of AgNO3 solution against 10
:
1 leaf extract. The grain size measurement and distribution curve was calculated using Image J software. EDX spectra confirmed the presence of silver and oxygen shown in Fig. 6(a)–(d) also indicates the high purity of the synthesized AgNPs.34
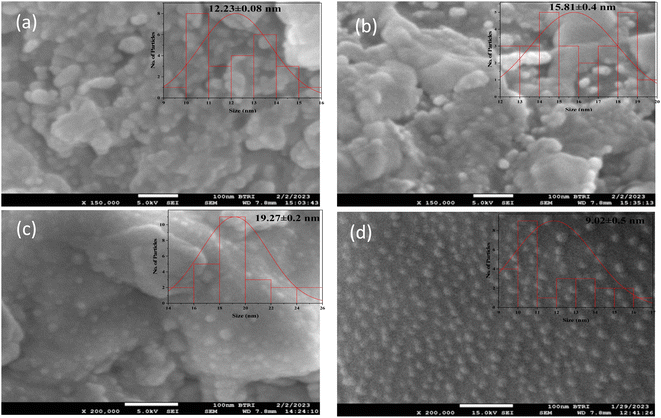 |
| Fig. 5 FE-SEM images with grain size distribution of AgNPs for (a) 10 mM (b) 15 mM (c) 20 mM and (d) 25 mM of AgNO3 solution. | |
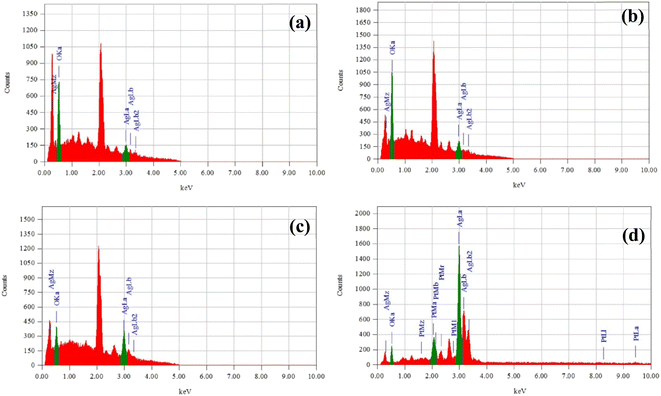 |
| Fig. 6 EDX spectra of AgNPs for (a) 10 mM (b) 15 mM (c) 20 mM and (d) 25 mM of AgNO3 solution. | |
The FE-SEM images of the treated cotton fiber and N95 mask surface are shown in Fig. 7(a) and (b) that sharper, clean, and pours free. Fig. 7(c) and (d) showed that the treated cotton fiber and N95 mask were coated homogeneously by AgNps. Oxygen plasma treatment creates more hydroxyl groups on the surface of the fibers that have stable conductive bonds with NPs, responsible for clinical isolation and antibiotic sensitivity.20
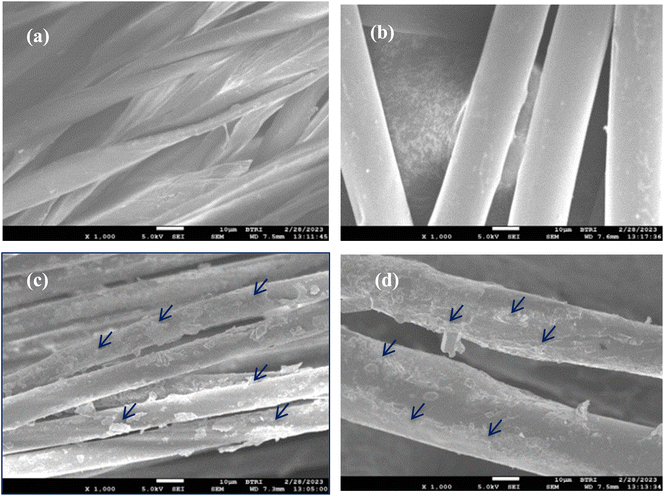 |
| Fig. 7 FE-SEM micrograph of (a) treated cotton (b) treated N95 mask (c) AgNPs/treated cotton and (d) AgNPs/treated N95 mask. | |
3.5 Fourier transforms infrared spectroscopy (FTIR) analysis
To detect functional group properties for NPs, FTIR is the most significant spectroscopic technique. FTIR spectra were employed to identify the photochemical elements in leaf extract-mediated AgNPs which are responsible for reducing and capping agents on Ag+ ions to AgNPs. Those bioactive different functional groups produce effective NPs. In our research, guavas leave extract contains bioactive high polyphenolics acid molecules whose presence was found in FTIR spectra. The FTIR spectra of AgNPs with different functional groups was shown in Fig. 8. It revealed that the characteristic peak at 3445 cm−1 can be attributed to the –OH stretching indicating the capping and reducing agent.8 In addition, –C–H stretching vibrations of alkane came from the carboxylic acid group at 2922 cm−1. Highly conjugated carboxylic functional groups were found at 1383 cm−1 absorption band and the materialization oxygen group –C–O vibrations were confirmed at 1113 cm−1 respectively. The peak 613 cm−1 confirmed the formation of AgNPs where the –OH phenolic bond was shown matches with.11 Thus, the fingerprint suggests that amid bonds belonging to aromatic, ethers and polyphenols are responsible bioactive elements that act as reducing and stabilizing agents for leaf extract-mediated AgNPs. The absorption peak was slightly shifted from guava leaf extract to AgNPs because of the formation of nanoparticles. Table 2 shows the different bioactive functional groups which confirm the formation of Ag+ ions to AgNPs.33,35
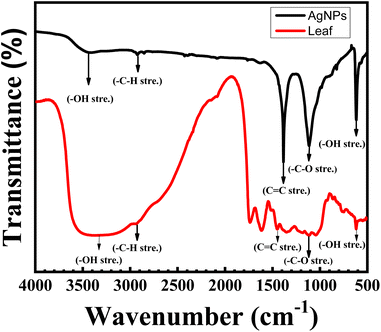 |
| Fig. 8 FT-IR spectra of P. guajava-mediated AgNPs and leaf extract. | |
Table 2 FT-IR data
Sample name |
Functional group at different characteristics absorptions (cm−1) |
O–H (stretching) |
C–H (alkane) |
C C (stretching) |
–C–O (stretching) |
O–H (stretching) |
AgNPs |
3445 |
2922 |
1383 |
1113 |
613 |
Leaf extract |
3433 |
2924 |
1444 |
1126 |
617 |
3.6 Antibacterial activity
The antibacterial screening of NPs is one of the important studies to find an antibacterial agent. NPs can be considered effective antibacterial agent when it exhibits a significant zone of inhibition (ZOI) against bacteria.36,37 A well disk diffusion method was applied for the measurement of zone of inhibition of P. guajava-assisted AgNPs.38 To attune the optical density (OD 0.1) of bacteria in culture UV-visible spectrophotometer (JASCO V-600, Japan) was used. The sterile wipe of cotton was used to give a layer of bacterial pathogens on the well-cultured agar plate. Clinical pathogenic bacteria Gram-negative Escherichia coli (E.coli) and Gram-positive Staphylococcus aureus (S. aureus) were placed on a properly sterilized nutrient agar plate and kept in incubator at 37 °C for the whole night. In the experiment, the agar plate was prepared for each bacterium following the same procedure.
Afterward, AgNPs in various AgNO3 concentration solutions were incorporated in the Gram-negative bacteria to confirm the activities of NPs. Also AgNPs/treated cotton and AgNPs/treated N95 masks were poured in Gram-negative bacteria (E. coli) and Gram positive (S. aureus) coated plate for testing its antibacterial activities in wet condition (Fig. 9). The samples were placed in oven for 2 hours so it may diffuse completely and then incubated for 24 hours at 37 °C. Finally, the diameters of restrictive zones were measured in mm.38 The measurement values of zone of inhibition for each bacterial strain were shown in Tables 3 and 4.
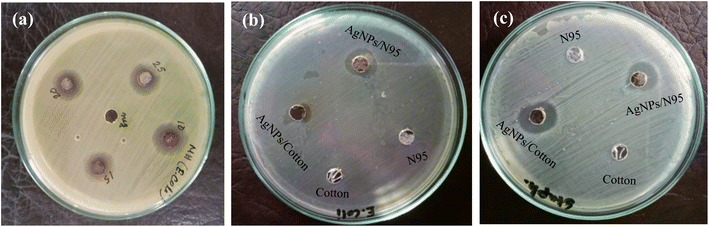 |
| Fig. 9 Antibacterial activity of AgNPs (a) 10 mM, 15 mM, 20 mM, and 25 mM AgNO3 solutions (b) AgNPs/treated cotton and AgNPs/treated N95 mask for E. coli (Gram-negative) bacteria and (c) AgNPs/treated cotton and AgNPs/treated N95 mask for S. aureus (Gram-positive) bacteria. | |
Table 3 Zone of inhibition (ZOI) for AgNPs solution against E.coli (Gram-negative) bacteria
AgNPs with different concentration of AgNO3 solution (mM) |
Zone of inhibition for Gram-negative (E. coli) (mm) |
10 |
13.00 |
15 |
13.50 |
20 |
13.50 |
25 |
14.00 |
Table 4 Zone of inhibition for the E. coli (Gram-negative) bacteria and S. aureus (Gram-positive) bacteria
Name of bacteria |
Zone of inhibition for AgNPs/treated cotton (mm) |
Zone of inhibition for AgNPs/treated N95 mask (mm) |
E. coli |
11.50 |
15.00 |
S. aureus |
18.00 |
14.50 |
In case of Gram-negative bacteria, the antimicrobial activity varied for each sample with different solution concentration. Due to the structure complexity of Gram-negative bacteria, the effective inhibition zone is greater in coated N95 mask than pure cotton. Cell wall thickness of Gram-negative has bigger width.34 Also the coated AgNPs/treated cotton and AgNPs/treated N95 mask showed higher ZOI with strong antibacterial activity.
4. Conclusions
Silver nanoparticles were fabricated using aqueous leaf extract of P. guajava following green synthesis route. The reduction of silver ions from AgNO3 to metallic Ag occurs due to leaf extract of P. guajava. The synthesized AgNPs were confirmed by observing the change of color initially and the absorbance peak at ∼430 nm in UV-Vis spectroscopic analysis. Moreover, the XRD data revealed that it corresponds to the face-centered cubic structure of crystalline silver. Thus, the present method leads to the formation of silver nanoparticles with well-defined dimensions. The average crystalline size was estimated ∼22 nm confirming the nanoparticle nature. However, FE-SEM analysis revealed that the material was formed in nano-dimension, with an average grain size ∼12 nm. The particles were almost spherical in shape though their occurs has some agglomeration. In addition, the FTIR study showed the reduction of Ag+ ions owing to the presence of biomolecules in the leaf extract, which acted as reducing as well as capping agents. Examination showed significant antibacterial activities of the synthesized AgNPs solution. The zone of inhibition in homogeneously coated treated cotton and N95 mask fiber showed a promising result against Gram-negative (E. coli) and Gram-positive (S. aureus) bacteria. The photo resistive properties of synthesized AgNPs signify their utility as an antibacterial coating in cotton textile applications.
Conflicts of interest
The authors declare that they have no conflict of interest.
Acknowledgements
Bangladesh Council of Scientific and Industrial Research (BCSIR), Dr Qudrat-I-Khuda Road, Dhanmondi, Dhaka-1205, Bangladesh.
References
- S. Piretarighat, M. Ghannadnia and S. Baghshahi, J. Nanostruct. Chem., 2019, 9(1), 1–9 CrossRef.
- I. Ijaz, E. Gilani, A. Nazir and A. Bukhari, Green Chem. Lett. Rev., 2020, 13(3), 223–245 CrossRef CAS.
- D. A. Kader, S. O. Rashid and K. M. Omer, RSC Adv., 2023, 13, 9963 RSC.
- R. Khatun, M. S. A. Mamun, S. Islam, N. Khatun, M. Hakim, M. S. Hossain, P. K. Dhar and H. R. Barai, Micromachines, 2022, 13(12), 2077 CrossRef PubMed.
- J. R. Vega-Baudrit, S. M. Gamboa, E. R. Rojas and V. V. Martinez, Biosens. Bioelectron., 2019, 5, 5 Search PubMed.
- P. Velmurugan, M. Iydroose, S. Lee, M. Cho, J. Park, V. Balachandar and B. T. Oh, Indian J. Microbiol., 2014, 54, 196–202 CrossRef CAS PubMed.
- J. D. S. Newman and G. J. Blanchard, Langmuri, 2006, 22(13), 5882–5887 CrossRef CAS PubMed.
- D. S. Sheny, J. Mathew and D. Philip, Spectrochim. Acta, Part A, 2011, 79(1), 254–262 CrossRef CAS PubMed.
- D. Bose and S. Chattrjee, Appl. Nanosci., 2016, 6(6), 895–901 CrossRef CAS.
- S. P. Patil and P. M. Rane, J. Basic Appl. Sci., 2020, 9, 60 Search PubMed.
- M. J. Islam, M. T. Khatun, M. R. Rahman and M. M. Alam, AIP Adv., 2021, 11, 125223 CrossRef CAS.
- P. Somchaidee and K. Tedsree, Adv. Nat. Sci.: Nanosci. Nanotechnol., 2018, 9, 035006 Search PubMed.
- R. Saha, S. Karthik, P. M. R. S. A. Kumar, R. Suriyaprabha and V. Rajendran, Prog. Org., 2018, 124, 80–91 CAS.
- J. Singh, V. Kumar, K. Kim and M. Rawat, Environ. Res., 2019, 177, 108569 CrossRef CAS PubMed.
- M. J. Islam, Kamaruzzaman, M. F. Hossain, M. A. Awal, M. R. Rahman and M. M. Alam, AIP Adv., 2022, 12(11), 115116 CrossRef CAS.
- M. Liu, D. Zhang, S. Chen and T. Wen, J. Solid State Chem., 2016, 237, 32–35 CrossRef CAS.
- C. Sharmila, R. Rajamani and C. S. Bellan, Asian J. Pharm. Clin. Res., 2018, 11(1), 341–345 CrossRef CAS.
- G. Venugopal, Int. J. Bioassays, 2017, 6(7), 5441–5443 CrossRef.
- L. B. Dama, P. P. Mane, A. V. Pathan, M. S. Chandarki, S. R. Sonawane, S. B. Dama, S. R. Chavan, R. P. Chondekar and A. S. Vinchurkar, Sci. Res. Rep., 2016, 6(2), 89–95 Search PubMed.
- A. Fakhri and M. Naji, J. Photochem. Photobiol., B, 2017, 167, 58–63 CrossRef CAS PubMed.
- R. S. Zamel, B. K. Mohammed, A. S. Yaseen, H. T. Hussein and U. M. Nayef, J. Res. Lepid., 2019, 50(3), 82–95 CrossRef.
- X. Huang, P. K. Jain, I. H. El-Sayed and M. A. El-Sayed, Nanomedicine, 2007, 2, 681–693 CrossRef CAS PubMed.
- E. Tomaszewska, K. Soliwoda, K. Kadziola, B. Tkacz-Szczesna, G. Celichowski, M. Cichomski and J. Grobelny, J. Nanomater., 2013, 2013, 1–10 CrossRef.
- M. Alam, J. King Saud Univ., Sci., 2022, 34, 102327 CrossRef.
- R. Das, S. S. Nath, D. Chakdar, G. Gope and R. Bhattacharjee, J. Nanotechnol., 2009, 5, 1–6 Search PubMed.
- A. Taleb, C. Petit and M. P. Pileni, J. Phys. Chem., 1998, 102(12), 2214–2220 CrossRef CAS.
- S. Link and M. A. El-Sayed, Annu. Rev. Phys. Chem., 2003, 54(1), 331–366 CrossRef CAS PubMed.
- L. N. Khanal, K. R. Sharma, H. Paudyal and K. Parajuli, J. Nanomater., 2022, 2022(1), 1–11 Search PubMed.
- A. M. Awwad, N. M. Salem and A. O. Abdeen, Int. J. Ind. Chem., 2013, 4(1), 29 CrossRef.
- A. Rautela, J. Rani and M. Debnath, J. Anal. Sci. Technol., 2019, 10, 5 CrossRef.
- S. Islam, S. A. Satter, N. Khatun, M. S. Hossain, S. F. U. Farhad, P. Bala, S. Tabassum and A. Siddika, J. Mol. Eng. Mater., 2019, 1–9 Search PubMed.
- S. Islam, N. Khatun, M. S. Habib, S. F. U. Farhad, N. I. Tanvir, M. A. A. Shaikh, S. Tabassum, D. Islam, M. S. Hossain and A. Siddika, Heliyon, 2022, 8, e10529 CrossRef CAS PubMed.
- A. Jamaluddin and C. K. M. Faizal, IIUM Eng. J., 2018, 19(1), 178–184 CrossRef.
- S. I. I. Abdel-Hafez, N. A. Nafady, I. R. Abdel-Rahim, A. M. Shaltout, J. Daròs and M. A. Mohamed, 3 Biotech, 2016, 6(2), 199 CrossRef PubMed.
- V. Sreenivasulu, N. S. Kumar, M. Suguna, M. Asif, E. H. Al-Ghurabi, Z. X. Huang and Z. Zhen, Int. J. Electrochem. Sci., 2016, 9959–9971 CrossRef CAS.
- S. Roy, I. Hasan and B. Guo, Coord. Chem. Rev., 2023, 482, 215075 CrossRef CAS.
- H. Huahg, A. Ali, Y. Liu, H. Xie, S. Ullah, S. Roy, Z. Song, B. Guo and J. Xu, Adv. Drug Delivery Rev., 2023, 192, 114634 CrossRef PubMed.
- S. Shamaila, N. Zafar, S. Riaz, R. Sharif, J. Nazir and S. Naseem, J. Nanomater., 2016, 6(4), 71 CrossRef PubMed.
Footnote |
† Contributed equally to this work. |
|
This journal is © The Royal Society of Chemistry 2023 |