DOI:
10.1039/D3RA03168C
(Paper)
RSC Adv., 2023,
13, 18651-18657
Epsilon-poly-L-lysine conjugated erythromycin for enhanced antibiotic therapy†
Received
12th May 2023
, Accepted 15th June 2023
First published on 20th June 2023
Abstract
Antibiotic resistance is a big threat to public health. How to improve the therapeutic efficacy of conventional antibiotics is an effective way to address this issue. In order to enhance the antibacterial activity of conventional antibiotic erythromycin (EM), EM is conjugated to positively charged ε-poly-L-lysine (EPL) to obtain EPL modified EM (EPL-EM). The grafting ratio of EM can be calculated from the 1H NMR spectrum. EPL-EM is stable in physiological environment, while EM can be readily released from EPL-EM upon incubating with esterase which can be secreted by most bacteria. Because of the presence of cationic EPL, EPL-EM showed much stronger antibacterial activity than free EM, with much lower minimum inhibitory concentration (MIC) and minimum bactericidal concentration (MBC). Moreover, compared to free EM, the development of drug resistance can be slowed down if EPL-EM is used, which can be ascribed to the reduction of EM dosage. Meanwhile, EPL-EM cannot induce hemolysis and cytotoxicity, which indicates that EPL-EM exhibits excellent biocompatibility. The design of EPL-EM with enhanced antibacterial activity and excellent biocompatibility provides an innovative way to combat antibiotic resistance.
1. Introduction
Antibiotic resistance is one of the biggest threats to humans' life and health, which can affect anyone of all ages in any country.1–3 There are already some “superbugs” that are resistant to most of the antibiotics and other medications in the world, such as carbapenem-resistant Enterobacteriaceae, multidrug-resistant Acinetobacter baumannii, multidrug-resistant Klebsiella pneumoniae, and methicillin-resistant Staphylococcus aureus (MRSA). The World Health Organization (WHO) announced that we were in the post-antibiotic era in 2013.4 Based on a report from the WHO in 2017, about 700
000 people will be killed by antibiotic resistance, which will increase to 10 million by 2050 if new medical interventions are not developed.5 The overcome of antibiotic resistance was selected as one of the 125 scientific questions of greatest concern in the world by Science Magazine in 2021. It is urgently needed to develop new strategies to inhibit or decelerate the development of antibiotic resistance.6–9
There are already many strategies in combating antibiotic resistance.10–13 For example, we can use non-antibiotic strategies to treat bacterial infections.14,15 Photothermal therapy and photodynamic therapy are very effective in eliminating bacterial cells.16–20 Some transition metal (Ag, Cu, Zn, etc.) exhibit strong bacteriocidal capability and are very promising in sterilization.21–23 Antimicrobial peptides (AMPs) which have broad activity to directly kill bacterial cells are widely used in antibacterial applications.24–27 However, it is still very difficult for these non-antibiotic strategies in treating bacterial infections in vivo. On the other hand, the reduction of the dosage of antibiotics is also effective in inhibiting the development of antibiotic resistance.28,29 In order to achieve this goal, it is necessary to enhance the therapeutic efficacy of conventional antibiotics. Various antibiotic loaded nanoparticles were designed, which can enhance the therapeutic efficacy of conventional antibiotics by inhibiting degradation, improving biodistribution, enhancing biofilm penetration, enhancing drug–bacteria interaction, and so on.30–33 Chemical modification of conventional antibiotics is another effective way to improve the therapeutic efficacy. As a typical example, C-terminus guanidine modification of vancomycin was reported for enhanced antibacterial activity.34 Polyamidoamine (PAMAM) dendrimer conjugated azithromycin was much more effective in preventing productive infections than free azithromycin.35
Erythromycin (EM) is a widely used macrolide antibiotic, which exhibits strong inhibition ability against Gram-positive bacteria, including Staphylococcus aureus (S. aureus), Streptococcus pyogenes, Streptococcus viridans, Enterococcus faecalis (E. faecalis), and so on. However, the therapeutic efficacy of EM is always relatively poor in clinic. In order to improve the antibacterial activity of EM, ε-poly-L-lysine (EPL) conjugated EM (EPL-EM) was designed in this research. EPL is a cationic polypeptide with antibacterial activity. EPL can interact with the outer membrane of bacteria and lead to a permeabilization of bacterial membranes. Due to the presence of EPL, EPL-EM is expected to adhere to bacterial cells by electrostatic interaction and enhance the permeabilization of bacterial membranes, which might improve the antibacterial activity of EM. The dosage of EM can hence be reduced, which is beneficial for the deceleration of the development of antibiotic resistance.
2. Experimental section
2.1 Materials
Erythromycin (EM, Dalian Meilun Biotechnology Co., Ltd), ε-poly-L-lysine (EPL, Mw 3500–5000, Dalian Meilun Biotechnology Co., Ltd), succinic anhydride (Aladdin), esterase from porcine liver (Sigma-Aldrich), 1-ethyl-3-(-3′-dimethylaminopropyl) carbodiimide hydrochloride (EDC, Sigma-Aldrich), and N-hydroxysuccinimide (NHS, Energy Chemical) were used as received. Milli-Qwater (18.2 MX cm−1) was obtained using a Millipore Milli-Q Academic Water Purification System. Cell counting kit 8 (CCK-8) was purchased from Beyotime Biotechnology. S. aureus and E. faecalis were purchased from Guangdong culture collection center for all bacterial experiments. All other reagents were used as received without further purification.
2.2 Synthesis of succinic acid modified EM (EM-COOH)
EM-COOH was synthesized according to a reference with small modification by the reaction of EM and succinic anhydride.36 Briefly, 1 g (1.362 mmol) of AZM was added dissolved in 20 mL of N,N-dimethylformamide (DMF). 136.2 mg (1.362 mmol) of succinic anhydride and 300 μL of triethylamine (TEA) were added. The reaction mixture was stirred for 24 h. DMF was then removed under reduced pressure. The resulting crude product was purified by column chromatography using methanol: ethyl acetate: petroleum ether (1
:
2
:
1) as eluent. Yield: 42%.
2.3 Synthesis of EPL conjugated EM (EPL-EM)
To a stirred solution of EM-COOH (83.3 mg, 0.1 mmol) in 8 mL of DMSO and 2 mL of H2O, EDC (15.5 mg, 0.1 mmol) and NHS (11.05 mg, 0.1 mmol) were added to the reaction mixture. After 1 hour of stirring, 128 mg of EPL was added. The mixture was stirred at room temperature for 48 h. The reaction mixture was purified by dialysis using dialysis membrane (MWCO 1000 Da) with DMSO/H2O (8
:
2) for 48 h and Milli-Q water for another 48 h. The product was then freeze-dried and stored at 4 °C. Yield: 53%. The grafting ratio of EM in EPL-EM could be calculated based on the 1H NMR spectrum of EPL-EM. The molecular weight of EPL was already known, which could be used to calculate the polymerization degree of EPL. Therefore, the number of EM molecules conjugated to one EPL chain could be easily calculated.
2.4 In vitro drug release
In order to study esterase-responsive release of EM, 2 mL of EPL-EM (1 mg mL−1) in phosphate-buffered saline (PBS) containing esterase was added into a dialysis bag. EPL-EM in PBS without esterase was used as a control. The dialysis bag was then immersed in a prepared tube. The tube was put in a thermostated incubator at 37 °C with constant shaking (100 rpm). 0.5 mL of solution was taken out at different time intervals and replaced with fresh PBS. The concentration of released EM was detected by HPLC.
2.5 Determination of minimum inhibitory concentration (MIC) and minimum bactericidal concentration (MBC)
The MIC values of EPL-EM and free EM were determined by a standard broth microdilution method. S. aureus or E. faecalis at a concentration of 5 × 105 CFU mL−1 was incubated with different concentrations of EPL-EM and free EM (2-fold dilution, from 0.5 to 32 μg mL−1) in 96-well plates for 8 hours. After incubation, the MIC was read as the lowest antibiotic concentration completely inhibiting bacterial growth upon absorbance measurement by OD value, which was judged by lack of turbidity. The minimum bactericidal concentration (MBC) is the minimum concentration of an antibacterial agent that leads to 99.9% reduction of bacterial cells from initial bacterial inoculum. Culture wells with no visible turbidity were further sub-cultured for MBC determination. The solution from these wells were dropped on agar plates and incubated to determine MBC values. The MIC and MBC of EPL was measured similar to that of EPL-EM. The highest concentration of EPL used in this experiment was 256 μg mL−1.
2.6 In vitro bactericidal activity of EPL-EM
The bactericidal activity of EPL-EM and free EM was evaluated by standard plate counting assays. At first, 100 μL PBS, EPL, free EM, or EPL-EM with 2 μg mL−1, 4 μg mL−1, 8 μg mL−1, 16 μg mL−1 (2-fold dilution, equivalent to PMB) were added into the 100 μL S. aureus or E. faecalis in 96-well plates and incubated for 8 h. The suspensions were then serially diluted with sterile PBS and living bacteria were counted by plate counting method on the tryptone soy agar (TSA) plates.
2.7 Antibiotic resistance evaluation
The occurrence of antibiotic resistance was evaluated by determining MIC after successive incubating of S. aureus with EM. After incubating S. aureus with EPL-EM or EM, the MIC value against S. aureus (passage 0) was determined by a standard MIC test method. S. aureus bacterial cells were then treated with EPL-EM or EM at the concentration of 1/2 MIC. The survived bacterial cells were collected and designated as passage 1. The MIC of EPL-EM or EM against S. aureus (passage 1) was determined again. S. aureus bacterial cells were again treated with EPL-EM or EM at the concentration of 1/2 MIC (passage 1). The survived bacterial cells were collected and designated as passage 2. These operations were repeated for 10 successive passages. The MIC values of EPL-EM or EM against S. aureus at each passage were recorded, which was used to evaluate the development of antibiotic resistance.
2.8 Hemolysis evaluation
Rabbit red blood cells (RBCs) were used for hemolysis assay of EPL-EM. 100 μL whole blood was centrifuged at 1500 rpm for 5 min to collect RBCs. The obtained RBCs were washed and re-suspended in 2 mL PBS to prepare 5% v/v RBCs suspension. 100 μL RBCs suspension was mixed with EPL-EM (5, 10, 25, 50 μg mL−1). After 1 h incubation at 37 °C, the mixture was centrifuged at 1500 rpm for 5 min. The absorbance at 576 nm was detected using a microplate reader. PBS was used as negative control. 0.5% Triton X-100 was taken as full hemolysis. The percentage of hemolysis was calculated through eqn (1) |
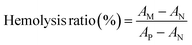 | (1) |
where AM is the absorbance of EPL-EM group, AN is the absorbance of PBS group, and AP is the absorbance of Triton X-100 group.
2.9 In vitro cytotoxicity evaluation
The cytotoxicity of EPL-EM was evaluated by a standard CCK-8 assay. Human corneal epithelial cells (HCE-T) or mouse fibroblast cells (L929) were cultured in 96-well plates at a density of 10
000 cells per well. After 24 h incubation, the culture medium was replaced with EPL-EM (5, 10, 25, 50 μg mL−1) in fresh medium and incubated for another 24 h. The medium was then replaced with fresh medium containing CCK-8 (10%) for 1 h. Finally, the viability of cells was detected by a microplate Bio-Rad reader at 450 nm. Data were presented as mean ± standard deviation (SD) (n = 4).
2.10 Characterizations
1H NMR and 13C NMR spectra were recorded on a Bruker DMX500 spectrometer. The mass spectra were recorded on a Bruker Esquire 3000 plus ion trap mass spectrometer equipped with an ESI source. The concentration of EM was determined by HPLC using Agilent 1200. The mobile phase was: acetonitrile: 0.4 M NH4H2PO4 = 1
:
2. The microplate reader we used was Bio-Rad PR 4100 microplate reader.
2.11 Statistical analysis
Data were expressed as mean ± SD and the significant difference was determined by Student's t-test. no significance: n.s, *P < 0.05, **P < 0.01, ***P < 0.001.
3. Results and discussion
3.1 Synthesis of EPL conjugated EM (EPL-EM)
In order to synthesize EPL-EM, carboxylated EM (EM-COOH) was firstly obtained by reacting EM with succinic anhydride (Scheme 1). The successful synthesis of EM-COOH was confirmed by 1H NMR, 13C NMR, and ESI-MS. The appearance of a peak at δ 2.73–2.80 in Fig. S1† could be ascribed to –CH2− next to the carboxyl group of EM-COOH in 1H NMR. Meanwhile, two peaks (δ 174.0, δ 175.4) assigned to the carbonyl group were observed in 13C NMR of EM-COOH (Fig. S2†). The ESI-MS result in Fig. S3† was consistent with the theoretical molecular weight of EM-COOH. All these results proved the successful synthesis of EM-COOH. EPL-EM was then prepared after conjugating EM-COOH to EPL by the amidation reaction. The 1H NMR spectrum of EPL-EM was shown in Fig. 1. The grafting ratio of EM in EPL-EM was calculated to be 8.1% based on the 1H NMR spectrum of EPL-EM. Therefore, the loading content of EM in EPL-EM was 31.5%. The molecular weight of EPL we used was in the range of 3500 to 5000. Due to the relatively high molecular weight of EM (Mw 734), 2 to 3 EM molecules in average were conjugated to one EPL chain. It should be noted that EM cannot be dissolved in water. High grafting ratio of EM might induce the precipitation of EPL-EM in aqueous solution. Therefore, the grafting ratio of EM was kept relatively low in this research.
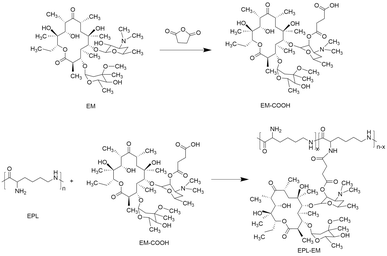 |
| Scheme 1 Schematic illustration of the synthetic route of EPL-EM. | |
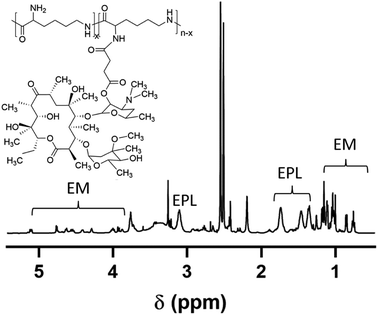 |
| Fig. 1 The 1H NMR spectrum of EPL-EM. DMSO-d6 was used as the solvent. | |
The effective drug release is a critical step for prodrugs. EM was conjugated to EPL-EM via an esterase-sensitive ester bond (Scheme 2). The esterase can be secreted by most of bacterial strains. Therefore, the release of EM from EPL-EM was investigated in the presence of esterase by high performance liquid chromatography (HPLC). As shown in Fig. 2, EPL-EM exhibited outstanding stability in PBS without drug leakage. However, EM could be effectively released from EPL-EM in the presence of esterase. More than 60% of EM was released after incubating with esterase for 6 h, which could further increase to 90% upon prolonging incubating time to 24 h. Therefore, EPL-EM was stable in physiological environment, while EM could be readily released in bacterial infected sites.
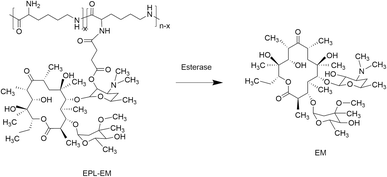 |
| Scheme 2 Schematic illustration of esterase-responsive release of EM from EPL-EM. | |
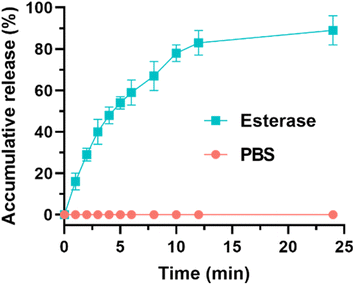 |
| Fig. 2 The release of EM from EPL-EM in PBS with or without esterase (n = 3). | |
3.2 In vitro antibacterial activity evaluation
After successful synthesis of EPL-EM, the antibacterial activity of EPL-EM was evaluated using free EM as control. Since EM is mainly used to treat Gram-positive bacterial infections in clinic, the Gram-positive bacterial strains including S. aureus and E. faecalis were used as model strains. Firstly, the minimal inhibitory concentration (MIC) and minimal bactericidal concentration (MBC) of EPL-EM (EM equivalent) were determined. As shown in Table 1, the MIC of EPL-EM against S. aureus was 1 μg mL−1 (EM equivalent), which was lower than that of free EM (2 μg mL−1). Meanwhile, the MIC of EPL-EM (1 μg mL−1) against E. faecalis was also lower than free EM (4 μg mL−1). Similarly, the MBC of EPL-EM against S. aureus and E. faecalis were 4 μg mL−1 and 8 μg mL−1, respectively, which were lower than that of free EM. However, the MIC and MBC of EPL against S. aureus and E. faecalis were higher than 256 μg mL−1, which implied relatively low antibacterial activity.
Table 1 The MIC and MBC of EM, EPL-EM, and EPL against different bacterial stains, including S. aureus and E. faecalis
Bactericidal agents |
MIC (μg mL−1) |
MBC (μg mL−1) |
S. aureus |
E. faecalis |
S. aureus |
E. faecalis |
EM |
2 |
4 |
16 |
16 |
EPL-EM (EM equivalent) |
1 |
1 |
4 |
8 |
EPL |
>256 |
>256 |
>256 |
>256 |
The bactericidal activity of EPL-EM was further evaluated by the standard plates counting assay. EPL-EM with different concentrations (2, 4, 8, and 16 μg mL−1 EM equivalent) were incubated with ∼108 colony forming units (CFU) S. aureus or E. faecalis for 8 h. The residual live bacterial cells were evaluated by the plates counting assay. As depicted in Fig. 3, EPL-EM exhibited stronger antibacterial activity than free EM against S. aureus. Specifically, EPL-EM and free EM at 16 μg mL−1 (EM equivalent) could lead to 3.8 and 2.7 orders of magnitude reduction of S. aureus colonies, respectively. EPL didn't exhibit obvious antibacterial activity due to the very low incubation concentration (35 μg mL−1). Similarly, EPL-EM and free EM at 16 μg mL−1 (EM equivalent) resulted in 4.6 and 3.6 orders of magnitude reduction of E. faecalis colonies, respectively (Fig. 4). Therefore, the conjugation of EM to EPL could significantly increase the antibacterial activity of EM. EPL-EM is a positively charged polymer and can adhere to negatively charged bacterial cells, which can lead to the accumulation of EM around bacterial cells. In this case, the local concentration of EM might be very high compared with free EM. Meanwhile, EPL itself is a cationic polypeptide with antibacterial activity. Therefore, EPL-EM showed much stronger antibacterial activity than free EM.
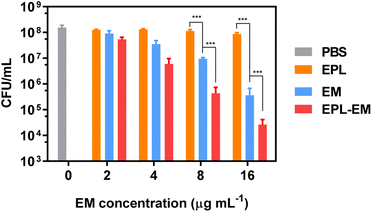 |
| Fig. 3 The in vitro antibacterial capability against S. aureus was evaluated the standard plates counting assay. S. aureus was incubated with different concentrations of EPL, EM, or EPL-EM for 8 h (n = 4). Statistical analysis was conducted by the t-test; p value: p < 0.05, *; p < 0.01, **; p < 0.001, ***. | |
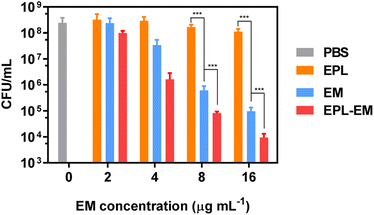 |
| Fig. 4 The in vitro antibacterial capability against E. faecalis was evaluated the standard plates counting assay. E. faecalis was incubated with different concentrations of EPL, EM, or EPL-EM for 8 h (n = 4). Statistical analysis was conducted by the t-test; p value: p < 0.05, *; p < 0.01, **; p < 0.001, ***. | |
As discussed above, EPL-EM showed much stronger antibacterial activity than free EM against planktonic bacteria. The biofilm-associated infection is a great challenge in clinic. Since EM was conjugated to positively charged EPL, the relatively large molecular and positive charge may inhibit the penetration of EPL-EM into bacterial biofilms, which may influence the therapeutic efficacy of biofilm-associated infections. The antibiofilm effect of EPL-EM will be investigated in future.
3.3 Reduced antibiotic resistance by EPL-EM
Although EM is a widely used antibiotic in clinic, the serious EM resistance is a critical issue. By conjugating EM to EPL, the antibacterial capability of EM was significantly improved. Therefore, compared to free EM, the dosage of EM could be remarkably reduced in the form of EPL-EM because of the enhanced antibacterial capability. In this case, it is very interesting to see if EPL-EM can reduce the occurrence of resistance compared with free EM. S. aureus bacterial cells were serially incubated with a sublethal concentration of EPL-EM or free EM. The MIC values were determined after each passage in the whole procedure. As shown in Fig. 5, the MIC of free EM at passage 10 was 10.6-fold as that of passage 0. In contrast, the MIC of EPL-EM at passage 10 was 4.7-fold as that of passage 0. Therefore, compared to free EM, EPL-EM could reduce the occurrence of EM resistance.
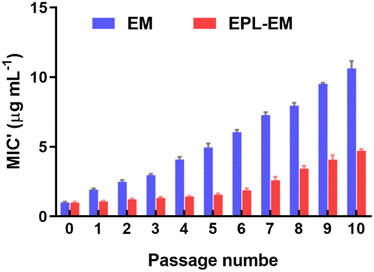 |
| Fig. 5 Resistance development of S. aureus after continuous exposure toward EPL-EM or free EM at the concentration of half MIC for 10 passages (n = 3). | |
3.4 Biocompatibility evaluation
After confirmed the excellent antibacterial activity of EPL-EM, the biocompatibility of EPL-EM was further evaluated. At First, the hemolytic toxicity of EPL-EM was investigated by incubating EPL-EM with RBCs. As sown in Fig. 6, EPL-EM cannot induce obvious hemolysis when the polymer concentration was 50 μg mL−1, which implied excellent hemocompatibility of EPL-EM. The cytotoxicity of EPL-EM was further studied by a CCK-8 assay. Human corneal epithelial cells (HCE-T) and mouse fibroblast cells (L929) were used as model cells. After incubating EPL-EM (5, 10, 25, 50 μg mL−1) with HCE-T cells or L929 cells for 24 h, the cell viability was higher than 95%, which implied that EPL-EM exhibited excellent cytocompatibility (Fig. 7). Therefore, EPL-EM maintained outstanding biocompatibility after EM was conjugated with EPL, which was extremely important for practical applications.
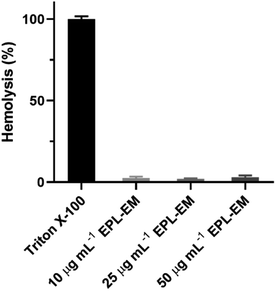 |
| Fig. 6 Hemolysis of mouse red blood cells after incubated with different concentrations of EPL-EM. Triton X-100 was used as a positive control (n = 3). | |
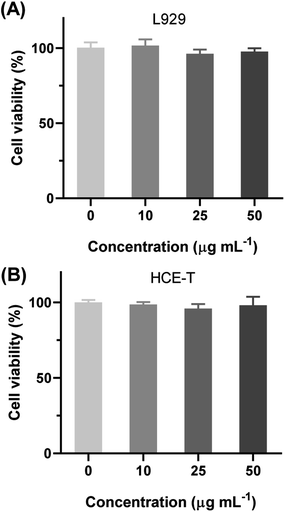 |
| Fig. 7 The cell viability of (A) L929 and (B) HCE-T after incubating with different concentration of EPL-EM for 24 h (n = 4). | |
4. Conclusions
In summary, EPL conjugated EM was successfully synthesized by the amidation reaction between EPL and EM-COOH. The grafting ratio of EM in EPL-EM was 8.1% determined by 1H NMR. EPL-EM maintained stable in physiological environment without drug leakage. However, the release of EM from EPL-EM was significantly accelerated in the presence of esterase, which was overexpressed by most bacterial strains. The MIC values of EPL-EM (EM equivalent) against S. aureus and E. faecalis were both 1 μg mL−1, which was much lower than that of free EM. Similarly, the MBC of EPL-EM against S. aureus and E. faecalis was also lower than that of free EM. EPL-EM exhibited much stronger antibacterial activity against Gram-positive bacteria than free EM. Due to the excellent antibacterial activity of EPL-EM, much fewer dosage of EPL-EM could be used in antibacterial applications. Compared to free EM, the development of resistance could be slowed down if EPL-EM was used. Meanwhile, EPL-EM could not induce hemolysis and cytotoxicity, exhibiting outstanding biocompatibility. This research provides an innovative strategy to improve the antibacterial activity of EM while not bring in hemolysis and cytotoxicity, showing great potential in antibacterial applications.
Author contributions
Jie Yu: methodology, investigation, validation, writing – original draft; Danwei Cen: validation, formal analysis, writing – review & editing; Yongcheng Chen: investigation, visualization; Hailan Zhao: software, visualization; Mengyue Xu: software; Sulan Wu: data curation; Shuo Wang: supervision, project administration, writing – review & editing; Qiao Jin: project administration, supervision; Ting Shen: conceptualization, supervision, project administration, funding acquisition, writing – review & editing.
Conflicts of interest
There are no conflicts to declare.
Acknowledgements
Financial support from General research program of Zhejiang Provincial Health Commission (2021KY076) is gratefully acknowledged.
References
- D. G. J. Larsson and C.-F. Flach, Nat. Rev. Microbiol., 2022, 20, 257–269 CrossRef CAS PubMed.
- C. Nathan and O. Cars, N. Engl. J. Med., 2014, 371, 1761–1763 CrossRef PubMed.
- R. Laxminarayan, D. Sridhar, M. Blaser, M. Wang and M. Woolhouse, Science, 2016, 353, 874–875 CrossRef CAS PubMed.
- M. Mckenna, Nature, 2013, 499, 394–396 CrossRef CAS PubMed.
- M. E. A. de Kraker, A. J. Stewardson and S. Harbarth, PLoS Med., 2016, 13, e1002184 CrossRef PubMed.
- Y. Chen, Y. Huang and Q. Jin, Macromol. Chem. Phys., 2022, 223, 2100440 CrossRef CAS.
- C. Liu, Z. Yang, X. Song, Y. Qian, H. Huo, J. He, J. Zhang, Z. Zhang, M. Shi, J. Pang, B. Zhang and W. Tian, Supramol. Mater., 2022, 1, 100014 Search PubMed.
- L. Zou, X. Li, Y. Huang, C. Wang, Y. Fang, J. Zhao, Q. Jin and J. Ji, Nano Today, 2023, 50, 101828 CrossRef CAS.
- D. C. Angst, B. Tepekule, L. Sun, B. Bogos and S. Bonhoeffer, Proc. Natl. Acad. Sci. U. S. A., 2021, 118, e2023467118 CrossRef CAS PubMed.
- D. Chinemerem Nwobodo, M. C. Ugwu, C. Oliseloke Anie, M. T. S. Al-Ouqaili, J. Chinedu Ikem, U. Victor Chigozie and M. Saki, J. Clin. Lab. Anal., 2022, 36, e24655 CrossRef PubMed.
- J. Murugaiyan, P. A. Kumar, G. S. Rao, K. Iskandar, S. Hawser, J. P. Hays, Y. Mohsen, S. Adukkadukkam, W. A. Awuah and R. A. M. Jose, et al., Antibiotics, 2022, 11, 200 CrossRef CAS PubMed.
- S. Chandra, P. Dutta and K. Biswas, ACS Nano, 2022, 16, 7–14 CrossRef CAS PubMed.
- Y. Jia, J. Zhang, Y. Zhao, R. Dong, H. Wang and X. Jiang, Chem. Commun., 2022, 58, 10544–10547 RSC.
- A. Mobed, M. Hasanzadeh and F. Seidi, RSC Adv., 2021, 11, 34688–34698 RSC.
- X. Chen, H. Han, Z. Tang, Q. Jin and J. Ji, Adv. Healthcare Mater., 2021, 10, 2100736 CrossRef CAS PubMed.
- Y. Wang, C. Zhang, H. Zhang, L. Feng and L. Liu, Chin. Chem. Lett., 2022, 33, 4605–4609 CrossRef CAS.
- X. Li, J. Man, H. Hu, J. Ye and Q. Jin, Colloid Interface Sci. Commun., 2022, 47, 100598 CrossRef CAS.
- Y. Huang, Q. Gao, X. Li, Y. Gao, H. Han, Q. Jin, K. Yao and J. Ji, Nano Res., 2020, 13, 2340–2350 CrossRef CAS.
- Y. Ren, H. Liu, X. Liu, Y. Zheng, Z. Li, C. Li, K. W. K. Yeung, S. Zhu, Y. Liang and Z. Cui, et al., Cell Rep. Phys. Sci., 2020, 1, 100245 CrossRef.
- O. A. Yeshchenko, N. V. Kutsevol, A. V. Tomchuk, P. S. Khort, P. A. Virych, V. A. Chumachenko, Y. I. Kuziv, A. P. Naumenko and A. I. Marinin, RSC Adv., 2022, 12, 11–23 RSC.
- Y. Huang, Q. Gao, C. Li, X. Chen, X. Li, Y. He, Q. Jin and J. Ji, Adv. Funct. Mater., 2022, 32, 2109011 CrossRef CAS.
- S. Chernousova and M. Epple, Angew. Chem., Int. Ed., 2013, 52, 1636–1653 CrossRef CAS PubMed.
- T. Bai, K. Zhao, Z. Lu, X. Liu, Z. Lin, M. Cheng, Z. Li, D. Zhu and L. Zhang, Chin. Chem. Lett., 2021, 32, 1051–1054 CrossRef CAS.
- S. Wang, Y. Yu, H. Li, Y. Huang, J. Wang, Q. Jin and J. Ji, J. Polym. Sci., 2022, 60, 2289–2297 CrossRef CAS.
- S. Nayab, M. A. Aslam, S. ur Rahman, Z. U. D. Sindhu, S. Sajid, N. Zafar, M. Razaq, R. Kanwar and Amanullah, Int. J. Pept. Res. Ther., 2022, 28, 46 CrossRef CAS.
- M. D. T. Torres, J. Cao, O. L. Franco, T. K. Lu and C. de la Fuente-Nunez, ACS Nano, 2021, 15, 2143–2164 CrossRef CAS PubMed.
- Q. Wang, Y. Xu and J. Hu, RSC Adv., 2022, 12, 14485–14491 RSC.
- Y. Gao, J. Wang, M. Chai, X. Li, Y. Deng, Q. Jin and J. Ji, ACS Nano, 2020, 14, 5686–5699 CrossRef CAS PubMed.
- N. Osman, N. Devnarain, C. A. Omolo, V. Fasiku, Y. Jaglal and T. Govender, Wiley Interdiscip. Rev. Nanomed. Nanobiotechnol., 2022, 14, e1754 Search PubMed.
- S. Wang, Y. Gao, Q. Jin and J. Ji, Biomater. Sci., 2020, 8, 6825–6839 RSC.
- W. Gao, Y. Chen, Y. Zhang, Q. Zhang and L. Zhang, Adv. Drug Delivery Rev., 2018, 127, 46–57 CrossRef CAS PubMed.
- E. Porges, D. Jenner, A. W. Taylor, J. S. P. Harrison, A. De Grazia, A. R. Hailes, K. M. Wright, A. O. Whelan, I. H. Norville and J. L. Prior, et al., ACS Nano, 2021, 15, 19284–19297 CrossRef CAS PubMed.
- K. Deng, Y. Li, X. Liang, C. Shen, Z. Zeng and X. Xu, Chin. Chem. Lett., 2022, 33, 1619–1622 CrossRef CAS.
- Z.-C. Wu, M. D. Cameron and D. L. Boger, ACS Infect. Dis., 2020, 6, 2169–2180 CrossRef CAS PubMed.
- M. K. Mishra, K. Kotta, M. Hali, S. Wykes, H. C. Gerard, A. P. Hudson, J. A. Whittum-Hudson and R. M. Kannan, Nanomedicine, 2011, 7, 935–944 CrossRef CAS PubMed.
- A. Bosnjakovic, M. K. Mishra, W. Ren, Y. E. Kurtoglu, T. Shi, D. Fan and R. M. Kannan, Nanomedicine, 2011, 7, 284–294 CrossRef CAS PubMed.
|
This journal is © The Royal Society of Chemistry 2023 |
Click here to see how this site uses Cookies. View our privacy policy here.