DOI:
10.1039/D3RA02927A
(Paper)
RSC Adv., 2023,
13, 22630-22638
Nanoengineering TiO2 for evaluating performance in dye sensitized solar cells with natural dyes†
Received
3rd May 2023
, Accepted 17th July 2023
First published on 26th July 2023
Abstract
The current study employs nanoengineering diatom and TiO2 NPs to form diatom-Si–TiO2 nanoengineered structures to fabricate a dye sensitized solar cell (DSSC) (DsTnas-DSSC). This was characterized and spin coated on a Fluorine-doped Tin Oxide (FTO) anode plate. The counter cathode was prepared by spin coating graphene oxide on a FTO glass plate and using Lugol's iodine as an electrolyte. The power density of DsTnas-DSSC was estimated with different natural dyes in comparison to conventional photosensitive ruthenium dye. It was found that the natural dyes extracted from plants and microalgae show significant power efficiencies in DSSC. The percentage efficiency of maximum power densities (PDmax) of DsTnas-DSSC obtained with photosensitive dyes were 9.4% with synthetic ruthenium dye (control) and 7.19% > 4.08% > 0.72% > 0.58% > 0.061% from natural dyes found in Haematococcus pluvialis (astaxanthin) > Syzygium cumini (anthocyanin) > Rosa indica (anthocyanin) > Hibiscus rosa-sinensis (anthocyanin) > Beta vulgaris (betalains), respectively. Among all the natural dyes used, the PDmax for the control ruthenium dye was 6.164 mW m−2 followed by the highest in astaxanthin natural dye from Haematococcus pluvialis (5.872 mW m−2). Overall, the use of natural dye DsTnas-DSSC makes the fuel cell low cost and an alternative to conventional expensive, metal and synthetic dyes.
1. Introduction
The global energy crisis is an immense problem that needs an economical and facile solution for energy generation as well as storage. A huge increase in fossil fuel consumption results in global warming and environmental instability. To reduce our dependence on fossil fuels, much attention has been paid to renewable energy sources.1 Development of photovoltaic technology is an important way to transform the Sun's energy into electrical energy which is the alternative source of energy on Earth.2,3 Photovoltaic cells (PVCs) play a significant role in energy harvesting as they efficiently harvest clean and renewable solar energy to produce electricity without exploiting harmful substrates and rigorous production approaches.4–7 Based on performance and cost effectiveness, the first-generation solar cells, specifically PVC silicon solar cells, currently dominate the market. Though they are effective since the energy conversion is high, on the other hand, they are very expensive. One such type is dye sensitized solar cell (DSSCs) which is a third generation photovoltaic device invented in 1991 by Grätzel et al.8 It is a promising technology with increasing interest as it has several advantages over conventional silicon solar cells. Fabrication process of DSSC is simple, inexpensive and can produce power even under low lighting conditions.9,10 Generally, a DSSC consists of photo-anode which is made up of fluorine doped tin-oxide (FTO) or indium doped tin-oxide, (ITO) and a porous monocrystalline semiconductor TiO2, a photosensitive dye (commonly ruthenium dye), an electrolyte and counter electrode which also have FTO/ITO covered glass coated usually with a thin film of platinum or carbon.11,12 Although DSSC has evolved in the past two decades but cost has remained a major barrier in commercialization. Due to this, scientist have been constantly working to lower down the cost of DSSC by incorporating nanomaterials and dyes which are cheap and photosensitive.
In nature diatoms are cheapest available biomaterials which have been demonstrated for their use in fuel cells viz.; DSSC, perovskite solar cells, microbial fuel cells.13–18 They are photosynthetic microalgae with 3-dimensional porous cell wall called frustules which is made of hydrogenated amorphous silica.19,20 It has nanoporous architecture which shows a vast diversity in shape and size and displays strong light scattering and trapping capacity.21–23 So the efficiency of DSSC can be enhanced by the integration of diatoms frustules onto anodes.24
In the present study, DsTnas-DSSC were synthesized by hydrothermal and co-precipitation method using diatom frustule rich in Si and TiO2 to prepare photoanode in a DSSC which was characterised via various state of art instruments. Transition metals like ruthenium have been reported as sensitizers with high stability and absorption spectra. They give good efficiency in a DSSC, but due to their high cost and upscaling, it is a cause for concern. On the offset, natural pigments are photosensitisers which are cheap and nontoxic but face un-stability and short shelf life.25 Among various natural dye tested in the present study astaxanthin (3,3′-dihydroxy-b,b′-carotene-4,4′-dione) biosynthesized from Haematococcus pluvialis has unique antioxidant properties.26,27 On the other hand, anthocyanin (2-phenyl-1-benzopyrlium) and betalanins (betaxanthins and betacyanins) pigment from various flowers, fruits and roots are tested as source of natural photosenitizer dye in DSSC.
These natural pigment have hydroxyl groups which allow them to assemble on the TiO2 film in a DSSC. Although these pigments are light and heat stable; a comparison of their use in DSSC will help to know the power efficiencies produced for the cheaper and efficient way of producing DSSC's.28
2. Materials and methods
Titanium(IV) oxide (TiO2), titania paste, ruthenium cis-bis(isothiocynato) bis(2,2′-bipyridyl-4,4′-dicarboxylato), ruthenium(II), iodine solution was purchased from Sigma-Aldrich (St. Louis, MO, USA). FTO glass slides (25 × 25 × 2.2 mm) with sheet resistance 15 U sq−1 were obtained from Techinstro, Maharashtra, India.
2.1. Synthesis of diatom (Si) doped TiO2 nanostructures
Diatom frustules of Nitzschia palea were harvested from pure culture which was grown in f/2 media under standard laboratory conditions.29,30 The live diatoms were acid washed and the obtained empty frustules were cleaned and dried.18 The diatom rich in Si were then doped with TiO2 nanoparticles (NPs) via hydrothermal and co-precipitation method31 with some modifications. Clean diatom frustules were washed, sieved and thermally annealed. Thereafter, diatom (Si) and TiO2 NPs at ratio of 20
:
80 g% respectively were assorted individually in water. The, pH of TiO2 NPs solution was calibrated to pH 11 while adding diatom rich Si dropwise. During preparation of DsTnas, solution was continuously stirred and agitated for 30–40 min. Once the reaction was completed a uniform suspension solution was formed which was cleaned and dried at temperature of near 24 h to form DsTnas.
2.2. Characterization of diatom (Si)–TiO2 nanoarray structures (DsTnas)
The nanocomposite mixture DsTnas was characterized by spectroscopy and microscopic studies. FT-IR spectra of commercial TiO2 NPs and DsTnas was done to determine the type of functional groups present in the samples. FT-IR spectra (Model: Bruker ATR Alfa II FT-IR spectrometer (USA)) of the DsTnas was recorded as per protocol mentioned elsewhere32 at the range of 400 cm−1 to 4000 cm−1.
2.3. Extraction of natural dyes
Natural dyes rich in pigments anthocyanin, astaxanthin and betalanins were extracted from different plant materials namely Beet root (Beta vulgaris), Rose (Rosa indica), Hibiscus (Hibiscus rosa-sinensis), Indian blackberry (Syzygium cumini) and microalgae Haematococcus pluvialis (red stage). Each plant material (1 g) was washed properly with distilled water, dried and chopped into small pieces and kept in 10 mL of acetone. After extraction in acetone, solution was centrifuged at 3000 × g for 5 min, filtered and stored in dark at 4 °C. The pH of the different natural dyes was analysed. All dye solutions were further analysed for their respective UV-vis absorption spectra using UV-vis spectrophotometer (Lab India 3000 double beam).
Furthermore, thin layer chromatography was done for all the natural dyes. The pigment was extracted from all plant-based cells using 100% acetone and incubated at room temperature for overnight using hexane
:
acetone (7
:
3). The sample extracts were analysed by thin-layer chromatography (TLC) on silica gel plates (Himedia). Samples were spotted and air dried whereas the methanol
:
acetic acid
:
water (4
:
1
:
3) was taken as the mobile phase.
The natural dye which showed best performance in DsTnas was processed for LCMS as per protocol described in our earlier study.33
2.4. Fabrication of DSSCs anode and cathode
The six number of FTO glass slides with conductive side were selected as cathode/anode in a DSSCs. The FTO plates were rinsed with a mixture of ethanol and isopropanol for about 40 min in an ultrasonic bath. The rutile anatase was spin coated on anode of FTO plates so as to make a uniform homogenous layer (1000 rpm; 15 seconds). Further, a thin film of DsTnas was spin coated on the anatase layer. The thickness of the DsTnas film was measured using Ambios XP-2 Profilometer. The spin coated FTO plates were thereafter annealed for 2 hours at 400 °C so as to remove the organic particles and enhance film compactness and crystallinity. After cooling at room temperature, FTO control plate was immersed into standard ruthenium dye (3 × 10−4 M) whereas the FTO test plates were dipped into five different natural pigment dyes and left for 2 h so that dyes gets properly adsorbed into films.8 Finally, it was dried in air and ready to assemble with counter cathode for DsTnas-DSSC device preparation. The counter cathode was fabricated by spin coating of graphene oxide (GO) over conductive side of FTO glass plate and annealed (400 °C for 2 hour).
2.5. Assembling of diatom (Si)–TiO2 dye sensitized solar cell (DsTnas-DSSC)
DsTnas coated anode was put over GO coated cathode in closed sandwich cell assembly so that conductive side of both the electrodes faced each other forming DsTnas-DSSC. The electrolyte, Lugol's iodine (50 mM) was introduced in between both the electrodes and kept in a vacuum chamber to bubble out any air if present in between the electrodes. The edges of plates were then sealed. The closed sandwich assembly was thus circuited with wires and their connections were connected thereafter with the digital multimeter and characterised by SEM and its current voltage (I–V) curve plotted (ESI Fig. 1†).
2.5.1. Measurement of voltage, current and power density of a DsTnas-DSSC. The prepared DsTnas-DSSC assembly was irradiated with the white LED at the intensity of 20.2 Klux. Thereafter the open circuit voltage, operating voltage, current and maximum power density (PDmax) of the fabricated DSSCs with ruthenium dye and different natural extracted pigment dyes were determined by digital multimeter (Model: MECO, 171B, India) individually using external resistance (1 Ω to 100 kΩ).34
3. Results and discussion
3.1. Characterization of diatom (Si)–TiO2 nanostructures by SEM and EDX
To fabricate anode of DSSC, TiO2 NPs (Fig. 1A) are doped on diatom frustules (Fig. 1B). The TiO2 NPs are doped inside the nanopores of diatom to form DsTnas nanocomposites (Fig. 1C). The anatase (Fig. 1D) is spin coated on FTO followed up by layering of DsTnas nanocomposite on it (Fig. 1E). It was found that SEM image of anatase appeared as cubes like single crystal structures and TiO2 NPs as nanoclusters. When the TiO2 NPs were functionalised within diatoms they formed diatom (Si)–TiO2 nanoarray structures with TiO2 NPs well-arranged inside nanopores of diatom frustules well in coherence to earlier reported studies.20
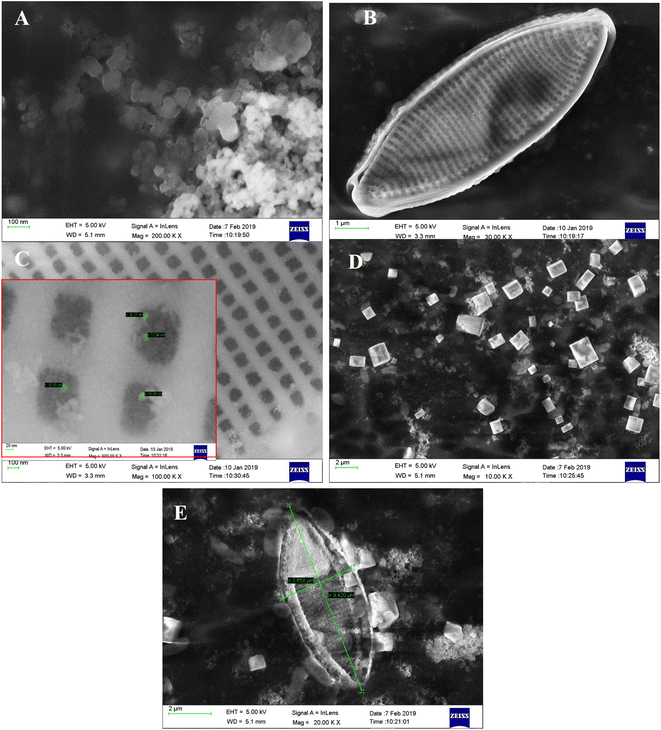 |
| Fig. 1 Morphological transformation shown via SEM images (A) TiO2 NPs; (B) diatom; (C) diatom frustules showing pores embedded with TiO2 NPs; (D) titania single crystal and (E) nanocomposite of diatom Si–TiO2 NPs. | |
The elemental composition of diatom and diatom (Si)–TiO2 nanoarray structures DsTnas were determined by energy-dispersive X-ray spectrometer (EDX) (Fig. 2A and B). The results showed that the diatoms had chemical compositions rich in C, O, Na, Si and Cl while diatom (Si)–TiO2 nanoarray structures displayed additional Ti, thus revealing that the diatoms were successfully doped with TiO2 NPs. Further, EDX of the assembled DsTnas-DSSC using standard ruthenium before its operation and without electrolyte (KI) and during operation in the presence of elelctrolyte potassium iodide (KI) showed altered chemical composition as well as incorporation of iodine ions due to the KI as electrolyte (ESI Fig. 2A and B†).
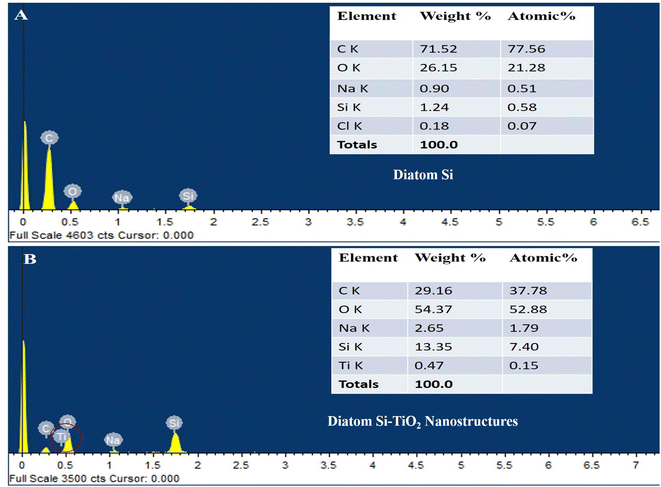 |
| Fig. 2 Scanning electron microscopy-energy dispersive X-ray analysis (SEM-EDX) of elemental composition of (A) diatom Si and (B) diatom Si–TiO2 NPs. | |
3.2. TEM analysis
It has been reported that diatoms frustules are rich in silica.18,35 Doping of diatoms (Si) with TiO2 NPs resulted in diatom (Si)–TiO2 nanoarray structures (DsTnas) which was also studied via TEM for high resolution study of nanoparticles nanoarray structures (Fig. 3 and ESI Fig. 3†). The TEM study showed an accurate deposition of TiO2 in diatom forming DsTnas. Further, the formation of these complex nanostructures was very well concordance with the XRD results. The TEM images of the TiO2 NPs showed that these NPs were not only spherical but also irregular in size and its distribution (Fig. 3A). Some NPs are large and spherical while others are small in size with different shapes.36 Further Fig. 3B shows selected area electron diffraction (SAED) pattern which is in accordance to the crystal plane structure of the nanostructures (Si–TiO2 NPs) (Fig. 3B). The HR-TEM further showed the well-arranged lattice fringes of the nanostructures (Si–TiO2 NPs) (Fig. 3C). The ‘d’ spacing of the lattice fringe was in the range of 0.45 nm (Fig. 3C). However, the average size of TiO2 NPs was ∼19.01 nm (Fig. 3D).37
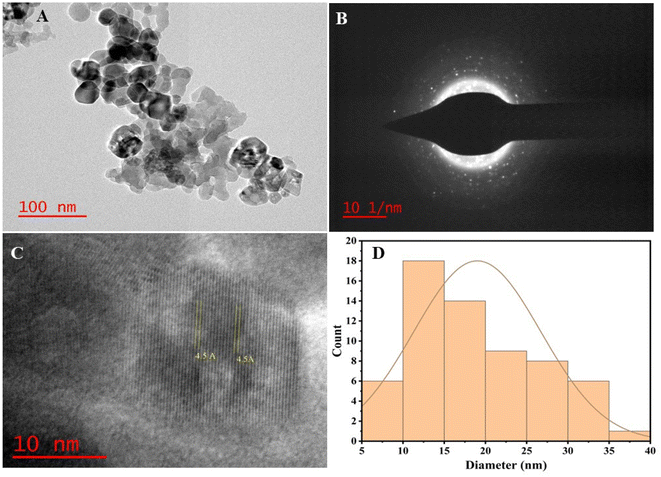 |
| Fig. 3 (A) TEM image of diatom-Si–TiO2 NP; (B) HR-TEM image of diatom-Si–TiO2 NP (C) selected area electron diffraction (SAED) pattern of diatom-Si–TiO2 NP for HR-TEM showing d spacing of 0.45 nm and (D) average size of TiO2 NPs. | |
3.3. Characterisation of functional groups and crystallographic study
3.3.1. FT-IR characterization. The FT-IR spectra of TiO2 and DsTnas was done at wavenumbers range of 500–4000 cm−1 (Fig. 4A and B). IR spectrum classify the chemical bonds as well as functional group present in the samples. The broadest band was seen at 3500 cm−1 which is due to O–H stretching of Ti–OH bonds. Strong absorption peak at 500–1100 cm−1 were seen which represented the Ti–O and Ti–O–Ti bonds. This was probably due to the vibrations arising due to bending and stretching between Ti–OH and Ti–O–Ti (Fig. 4A). There are sharp characteristic absorption bands around 600 cm−1 which corresponds to the rutile phase of TiO2 (Fig. 4A).38,39 The FT-IR spectrum of DsTnas showed that position 810 cm−1 corresponded to the Si bands which was due to the Si–O–Si vibrations.40 It was observed that siloxane vibrations at 1112 cm−1 were formed due to (SiO)n groups41,42 revealing that the silica content in diatom formed DsTnas (Fig. 4B).
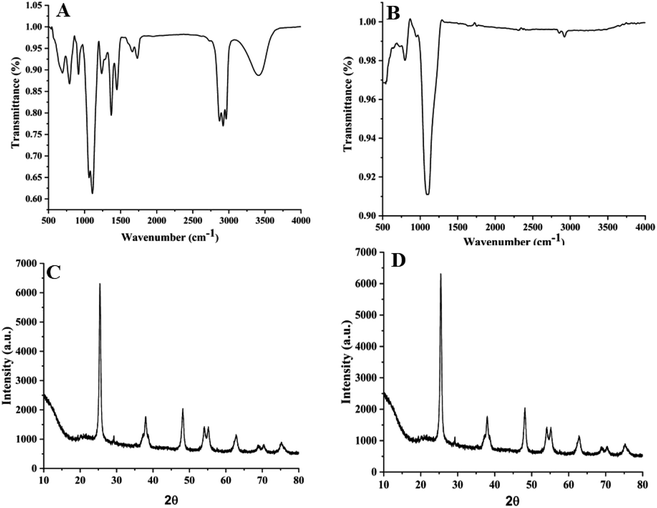 |
| Fig. 4 FTIR spectra of (A) TiO2 NPs and (B) diatom-Si doped TiO2 NPs and X-ray diffraction patterns of (C) TiO2 NPs and (D) diatom-Si doped TiO2 NPs. | |
3.3.2. XRD characterization. The TiO2 NPs and DsTnas were further evaluated by XRD technique (Fig. 4C and D). The XRD pattern of commercial TiO2 NPs is depicted in Fig. 4C. The broad diffraction line of the sample is related to the nanocrystalline anatase phase. The results of formation of strong peaks at about 2θ = 25.2°, 37.8°, 47.7°, 54.7°, 63.0° and 68.9° were well in accordance with indices at (101), (004), (200), (211), (204) and (116) which were part of anatase phase of titania (Fig. 4C).43 The XRD patterns in both TiO2 NPs and Si doped TiO2 NPs showed sharp and strong peak at 2θ = 25° of anatase phase. XRD phase identification patterns showed some minor peaks due to rutile phase. The XRD patterns of Si–TiO2 NPs are essentially crystalline structure which can be accomplished from the broad characteristic diffraction peak between 2θ value of 20–80° (Fig. 4D).44 The results are well in concordance with earlier reported from the literature which show that the absence of Si, does not change the crystalline structure of TiO2, therefore the XRD spectra of TiO2 and diatom-Si doped TiO2 NPs was almost similar. The optical, material and mechanical properties of diatom thus makes them as naturally available nanomaterial besides being rich in value added compounds mainly crude oil and fucoxanthin.45,46
3.4. UV-vis spectroscopy
Once the DsTnas was synthesized and characterised to serve as anode of the DSSC, it was necessary to test different natural dyes which would be acting as photosensitive dyes in the DSSC. The natural dyes extracted from Beet root (Beta vulgaris), Rose (Rosa indica), hibiscus (Hibiscus rosa-sinensis), Indian blackberry (Syzygium cumini) and microalgae H. pluvialis (red stage) were analysed for their pH which was 4, 3.5, 6.4, 4.1, 4.2 respectively and 2.9 for synthetic ruthenium dye (standard) (ESI Fig. 4†). Further, the absorbance peaks of each natural dye and standard ruthenium dye were analysed via UV-vis spectrophotometer. The absorption maxima of Rosa indica, Syzygium cumini, Hibiscus rosa-sinensis, H. pluvialis, Beta vulgaris and ruthenium was 534 nm, 522 nm, 480 nm, 490 nm, 474 nm and 505 nm, respectively (Fig. 5A) which is in coherence with the existing studies.47–51 The appearance of absorption spectra in the range between 454 and 540 nm indicated high concentration of anthocyanin isomers present in these extracted natural dyes.52,53 It was found that the Hibiscus rosa-sinensis displayed two wide absorption peaks at 430 nm and 540 nm.54 Similarly astaxanthin from H. pluvialis shows one peak at 490 nm which is in concordance with the reported studies.55,56 The shifts in the absorption peaks of the different pigment dyes were probably due to varied chemical structure of these natural pigment dyes. Further, the TLC studies have shown diverse separation of anthocyanins, astaxanthin and betalains in different TLC plates (Fig. 5B). TLC fraction of Hibiscus rosa-sinensis showed a single spot with Rf vale 0.40 identified as anthocyanin as per earlier reported work where Rf value of 0.32 was obtained using solvent mixture HCl
:
acetic acid
:
water.57 Similarly, TLC of Syzygium cumini fruit showed a single spot with Rf value of 0.31 as anthocyanin in consistency with reported literature wherein Rf value of 0.30 was obtained using methanol
:
formic acid
:
water was used as mobile phase.58 On the offset, TLC of H. pluvialis separated pigments such as primary xanthophylls astaxanthin monoester, astaxanthin diester, adonirubin ester, echinenone, adonixanthin diester, β-carotene with Rf value of 0.46, 0.51, 0.70, 0.72, 0.80, 0.84, 0.85 respectively. In a similar study conducted on microalga Bracteacoccus aggregatus BM5/15 using mobile phase hexane
:
chloroform
:
benzene, the separated pigments such as primary xanthophylls, astaxanthin monoester, astaxanthin diester, adonirubin ester, echinenone, adonixanthin diester, β-carotene displayed Rf value of 0.04, 0.24, 0.56, 0.44, 0.59, 0.80, 0.98, respectively.59
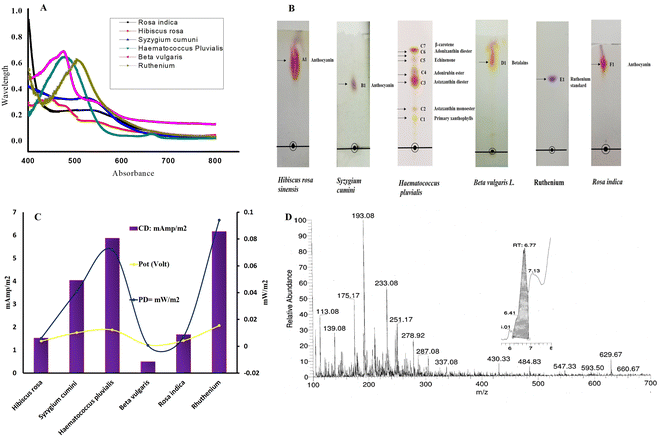 |
| Fig. 5 Characterisation of natural dyes Hibiscus rosa-sinensis; Syzygium cumini; Haematococcus pluvialis; Beta vulgaris; ruthenium and Rosa indica by (A) UV-vis spectroscopy, (B) thin layer chromatography (C) collective maximum current and PDmax of diatom rich Si–TiO2 nanoarray structures-dye sensitized solar cells using different plant dyes and their comparison with control (ruthenium) and (D) LCMS of dye (astaxanthin) from H. pluvialis. | |
TLC fraction of Beta vulgaris L. showed a single spot with Rf value of 0.5, identified as betacyanin. In a similar study on Beta vulgaris using a mixture of isopropanol
:
ethanol
:
water
:
acetic acid as mobile phase, betacyanin displayed Rf value of 0.48.60 The TLC fraction of ruthenium standard (Sigma Aldrich) showed single spot with Rf value of 0.50 in support with earlier reported studies.61 Additionally, the TLC fraction of Rosa indica showed a single spot of anthocyanin with Rf value of 0.42 in concordance with similar pigment with Rf value of 0.46 when 0.1% formic acid in aqueous solution and 0.1% formic acid acetonitrile was used as mobile phase.62
3.5. Current and power density of DsTnas-DSSC
The current density of DsTnas-DSSC using different dyes was analysed to evaluate its performance using range of resistance. The best changes in potential of DsTnas-DSSC with respect resistance (10 Ω to 100 kΩ) using dyes are shown Fig. 5C and that from resistance 10 kΩ to 120 kΩ in ESI Fig. 5† followed a similar pattern. The open circuit voltage was highest for H. pluvialis (0.92 mV) and lowest for Beta vulgaris (0.237 mV) compared to 0.732 mV of standard ruthenium (ESI Fig. 6†). The obtained potential was used to calculate current density using Ohm's law. The electrochemical data showed that the percentage efficiency of PDmax of DsTnas-DSSCs was 9.4% with control ruthenium dye. Among the natural pigment dyes that were used as photosensitive dyes in a DsTnas-DSSC for generating electric current, it was seen that percentage efficiency of PDmax from astaxanthin dye extracted from H. pluvialis was 7.19%. Further, natural dyes rich in anthocyanin from Syzygium cumini showed percentage efficiency of PDmax 4.08%. The natural dyes from other plant parts viz.; Beta vulgaris, Rosa indica and Hibiscus rosa-sinensis showed percentage efficiency of PDmax < 1%. The PDmax of DsTnas-DSSCs with different natural dyes were 6.164, 5.872, 4.04 1.676, 1.524, 0.492 mW m−2 at 50 kΩ for ruthenium dye (standard as control) and extracted dyes from H. pluvialis, Syzygium cumini, Rosa indica and Hibiscus rosa-sinensis and Beta vulgaris, respectively (Fig. 5C). These data showed that the ruthenium is most efficient dye followed by extracts of astaxanthin from H. pluvialis and anthocyanin from Syzygium cumini dye in DsTnas-DSSC. On the offset, anthocyanin natural dyes extracted from Rosa indica and Hibiscus rosa-sinensis and betalains from Beta vulgaris displayed least performance. In this study, an efficient and stable DSSC in which diatom DsTnas serves as an advantages material can be applied in the preparation of various cheap and facile DSSCs.
Since astaxanthin from H. pluvialis shows promising photosenitizer properties in the present fabricated DsTnas-DSSC, ITS LCMS was done. Fig. 5D represents the LCMS of astaxanthin from H. pluvialis as only pigment present in its mono and diester form and thus LCMS showed its diverse ester compounds. The chromatogram shows detection of m/z values at 287.92–284.3 denoting astaxanthin, which was comparative to studies in Chlorella vulgaris.63 Similarly other compounds at m/z like 233.08–233 for presence of halocynthiaxanthin, at m/z of 251.17–251 denoting (3S,4R,30R)-4-hydroxyalloxanthin as seen in Isochrysis galbana.64 Furthermore, m/z of 593.50–539 was identified as 53-7,8,7′,8′-tetradrhydroastaxanthin as in other studies on H. pluvialis.65 The DsTnas enhanced the electron extraction due to photonic property of diatom nanopores which promoted the formation of compact uniform and homogenous layer. The DSSCs performance significantly improved within the nanoarray diatom-Si structure embedded with TiO2. The large active surface area of diatom Si–TiO2 nanoarray structure helps in providing resonance in visible spectral range, thus increased possibility of photon induced electron excitation. Additionally, more electrolyte and dye bound to the material increased photon adsorptions from solar energy thus enhanced the DSSC efficiency.66
Thus, from these studies, it is evident that diatoms are being used in solar cells, batteries and electroluminescent technology.66 The solar-current conversion efficiency of the DSSC, a low-cost photovoltaic device, is approximately 12%. Ruthenium-based dye particles serve an important function when they are attached to crystalline anatase TiO2 films that serve as photoanodes and are immersed in electrolyte. The dye in the DSSC transfers electrons to the TiO2 film when exposed to sunlight thus creating electron hole pairs. Electrons pass through the TiO2 layer to conductive FTO glass to the appliance or load, and back to the electrolyte. To fill the dye's electron hole, the electrolyte releases electrons.67 Diatom frustule was employed by Jeffryes et al. in DSSC.68 They connected the composite frustules to the dye–TiO2 layer after culturing TiO2 into the frustules. The transparent frustule pores allows the penetration of photons thus allowing light scattering, light reflection and light focusing so as to direct photons to the dye.69,70 A thinner photoanode layer produced by improved photonic capture lowers the electron diffusion distance, boosts efficiency, and lowers the cost of production. Because the titanium put into the frustule is of poor quality and the TiO2 is primarily dispersed along the pores, the effectiveness of the TiO2-frustule layer can be increased. In a later work, Jeffryes et al.,66 created composite frustules with a homogeneous TiO2 coating layer using a deposition process. The effectiveness of the photoanode is increased by 3.7% with the addition of the composite frustule.66,68 Thus, it is clear that innovative diatom processing techniques can enhance the functionality of diatom devices. Gautam et al.,34 has further demonstrated that TiO2 NPs were doped on diatom by two-way cell culturing methods and direct doping methods using sol–gel method with additional direct incubation with Ag nanoparticles (Ag NPs) so as to obtain diatom (Si)–TiO2 nanotubes doped on DSSC.71 The DSSC doped with diatoms TiO2 nanotubes via direct doping with Ag NPs has shown facile power efficiency of 8.45% which was nearly close to that from two way culturing method (9.45%).34,71
However, in this work, we show how dye-sensitized solar cells using diatom-Si–TiO2 have better light trapping. According to the electrochemical data, the percentage efiiceincy of maximum power density (PDmax) of the DsTnas-DSSCs was with synthetic dye ruthenium dye (9.4%) and 7.19% from organic dye astaxanthin from H. pluvialis which is equally good in terms of its cost effectiveness. A difficult but possible commercial objective for the current DSSC technology is to get efficiency exceeding 15%. By increasing the light responsiveness of the sensitizers in the near IR spectral region, future advancements will concentrate on the short circuit current (JSC). Thus an easy and flexible new method is necessary to attain high efficiency to create novel ligands that result in the creation of new dyes or ruthenium sensitizers.72
4. Conclusion
In this study diatom DsTnas were synthesized, characterized via SEM, TEM, XRD, FTIR, UV-vis and assembled into DSSC. The astaxanthin, anthocyanin and betalains natural dyes were treated as photosensitizer dyes and compared with standard ruthenium dye. It was seen that astaxanthin from Haematococcus pluvialis displayed highest power density (5.872 mW m−2) among other anthocyanins and betalains natural dyes from different plant sources after standard synthetic ruthenium dye (6.164 mW m−2) used conventionally in DSSCs. This definitely can lead towards economical fabrication of DSSC thus depleting the cost of expensive ruthenium dye. This is probably because of enhanced charge transfer from the natural dye molecule and the diatom DsTnas surfaces. Pigments are produced in large quantities in plants. These photosensitive natural dyes possess hydroxyl groups which allow the binding to the TiO2 layer. Thus, from an economic aspect, natural dyes can be a good choice to fabricate DSSCs provided the shelf life of these dyes are increased as they are very sensitive to degradation. On the offset, among these natural dyes astaxanthin in esterified form shows more stability compared to anthocyanin or any other plant pigment thus can be a better alternate after ruthenium to be used in DSSC. This study is important as it opens the use of low-cost natural pigment dyes as photosensitizers to run DSSCs for electricity production.
Data availability
Data would be available on request.
Author contributions
MJK: literature review, writing-original draft, data curation; AA: data curation; VS: data curation; AR: data curation; SV: review; VV: conceptualization; supervision; writing – original draft; review & editing; Funding acquisition.
Conflicts of interest
The authors declare that they have no known competing financial interests or personal relationships that could have appeared to influence the work reported in this paper.
Acknowledgements
MJK thanks DST-Nanomission for Postdoc Fellowship. VV gratefully acknowledge to DST-Nanomission (Government of India) project no. (SR/NM/NT-1090/2014(G)) and CEFIPRA Indo French project no. 7133/2020 for funding support.
References
- M. Mourya, M. J. Khan, A. Ahirwar, B. Schoefs, J. Marchand, A. Rai, S. Varjani, K. Rajendran, J. R. Banu and V. Vinayak, Fuel, 2022, 314, 122738 CrossRef CAS.
- G. K. Singh, Energy, 2013, 53, 1–13 CrossRef.
- A. Hagfeldt, G. Boschloo, L. Sun, L. Kloo and H. Pettersson, Chem. Rev., 2010, 110, 6595–6663 CrossRef CAS PubMed.
- J. R. Benemann, Energy Convers. Manage., 1997, 38, S475–S479 CrossRef CAS.
- Y. Watanabe and D. O. Hall, Energy Convers. Manage., 1996, 37, 1321–1326 CrossRef CAS.
- S. Ashina, J. Fujino, T. Masui, T. Ehara and G. Hibino, Energy Policy, 2012, 41, 584–598 CrossRef.
- M. Wang, N. Chamberland, L. Breau, J. E. Moser, R. Humphry-Baker, B. Marsan, S. M. Zakeeruddin and M. Gratzel, Nat. Chem., 2010, 2, 385–389 CrossRef CAS PubMed.
- B. O'Regan and M. Grätzel, Nature, 1991, 353, 737–740 CrossRef.
- S. Mathew, A. Yella, P. Gao, R. Humphry-Baker, B. F. E. Curchod, N. Ashari-Astani, I. Tavernelli, U. Rothlisberger, M. K. Nazeeruddin and M. Grätzel, Nat. Chem., 2014, 6, 242 CrossRef CAS PubMed.
- D. Joly, L. Pellejà, S. Narbey, F. Oswald, J. Chiron, J. N. Clifford, E. Palomares and R. Demadrille, Sci. Rep., 2014, 4, 4033 CrossRef CAS.
- Y. Gao, J. Zhu, H. An, P. Yan, B. Huang, R. Chen, F. Fan and C. Li, J. Phys. Chem. Lett., 2017, 8, 1419–1423 CrossRef CAS.
- N. T. R. N. Kumara, A. Lim, C. M. Lim, M. I. Petra and P. Ekanayake, Renewable Sustainable Energy Rev., 2017, 78, 301–317 CrossRef CAS.
- M. J. Khan, S. Das, V. Vinayak, D. Pant and M. Ghangrekar, Chemosphere, 2022, 291, 132841 CrossRef CAS PubMed.
- A. Rai, M. J. Khan, A. Ahirwar, R. Deka, N. Singh, B. Schoefs, J. Marchand, S. Varjani and V. Vinayak, Int. J. Hydrogen Energy, 2022, 47, 42099–42121 CrossRef CAS.
- M. J. Khan, V. J. Suryavanshi, K. B. Joshi, P. Gangadharan and V. Vinayak, in Handbook of Algal Biofuels, Elsevier, 2022, pp. 363–384 Search PubMed.
- V. Vinayak, M. J. Khan, S. Varjani, G. D. Saratale, R. G. Saratale and S. K. Bhatia, J. Biotechnol., 2021, 338, 5–19 CrossRef CAS PubMed.
- M. J. Khan, N. Singh, S. Mishra, A. Ahirwar, F. Bast, S. Varjani, B. Schoefs, J. Marchand, K. Rajendran and J. R. Banu, Chemosphere, 2022, 288, 132589 CrossRef CAS PubMed.
- M. J. Khan, A. Pugazhendhi, B. Schoefs, J. Marchand, A. Rai and V. Vinayak, Chemosphere, 2022, 291, 132692 CrossRef CAS.
- M. J. Khan, R. Singh, K. B. Joshi and V. Vinayak, RSC Adv., 2019, 9, 22410–22416 RSC.
- S. Gautam, M. Kashyap, S. Gupta, V. Kumar, B. Schoefs, R. Gordon, C. Jeffryes, K. B. Joshi and V. Vinayak, RSC Adv., 2016, 6, 97276–97284 RSC.
- T. Fuhrmann, S. Landwehr, M. El Rharbi-Kucki and M. Sumper, Appl. Phys. B: Lasers Opt., 2004, 78, 257–260 CrossRef CAS.
- J. Romann, J.-C. Valmalette, M. S. Chauton, G. Tranell, M.-A. Einarsrud and O. Vadstein, Sci. Rep., 2015, 5, 17403 CrossRef CAS.
- J. Toster, K. S. Iyer, W. Xiang, F. Rosei, L. Spiccia and C. L. Raston, Nanoscale, 2013, 5, 873–876 RSC.
- K. Shin, Y. Jun, J. H. Moon and J. H. Park, ACS Appl. Mater. Interfaces, 2010, 2, 288–291 CrossRef CAS.
- G. Richhariya, A. Kumar, P. Tekasakul and B. Gupta, Renewable Sustainable Energy Rev., 2017, 69, 705–718 CrossRef CAS.
- V. Sirotiya, A. Ahirwar, M. Mourya, M. J. Khan, A. Rai, R. Kwatra, A. K. Sharma, B. Schoefs, J. Marchand and S. Varjani, Phytochem. Rev., 2022, 1–26 Search PubMed.
- A. Ahirwar, K. Kesharwani, R. Deka, S. Muthukumar, M. J. Khan, A. Rai, V. Vinayak, S. Varjani, K. B. Joshi and S. Morjaria, J. Biotechnol., 2022, 349, 32–46 CrossRef CAS PubMed.
- N. Prabavathy, R. Balasundaraprabhu, A. S. Kristoffersen, G. Balaji, S. Prasanna, K. Sivakumaran, M. Kannan, S. R. Erga and D. Velauthapillai, Optik, 2021, 227, 166053 CrossRef.
- M. J. Khan, R. Singh, K. Shewani, P. Shukla, P. Bhaskar, K. B. Joshi and V. Vinayak, Sci. Rep., 2020, 10, 1–11 CrossRef PubMed.
- V. Vinayak, R. Gordon, S. Gautam and A. Rai, Adv. Sci. Lett., 2014, 20, 1256–1267 CrossRef.
- C. C. Kee, B. C. Ang and H. S. C. Metselaar, Ceram. Int., 2021, 47, 4803–4812 CrossRef CAS.
- A. Ahirwar, M. J. Khan, V. Sirotiya, M. Mourya, A. Rai, B. Schoefs, J. Marchand, S. Varjani and V. Vinayak, BioEnergy Res., 2022, 1–14 Search PubMed.
- M. Mourya, M. J. Khan, V. Sirotiya, A. Ahirwar, B. Schoefs, J. Marchand, S. Varjani and V. Vinayak, RSC Adv., 2023, 13, 17611–17620 RSC.
- S. Gautam, M. Kashyap, S. Gupta, V. Kumar, B. Schoefs, R. Gordon, C. Jeffryes, K. B. Joshi and V. Vinayak, RSC Adv., 2016, 6, 97276–97284 RSC.
- M. J. Khan, R. Gordon, S. Varjani and V. Vinayak, Sci. Total Environ., 2022, 823, 153667 CrossRef CAS PubMed.
- R. Tamayo, R. Espinoza-González, F. Gracia, U. P. Rodrigues-Filho, M. Flores and E. Sacari, Nanomaterials, 2019, 9, 1–16 CrossRef PubMed.
- D. Zhang and Z. J. Liu, Appl. Mech. Mater., 2010, 26–28, 835–838 CAS.
- V. G. Erkov, S. F. Devyatova, E. L. Molodstova, T. V. Malsteva and U. A. Yanovskii, Appl. Surf. Sci., 2000, 166, 51–56 CrossRef CAS.
- N. D. Abazović, M. I. Čomor, M. D. Dramićanin, D. J. Jovanović, S. P. Ahrenkiel and J. M. Nedeljković, J. Phys. Chem. B, 2006, 110, 25366–25370 CrossRef PubMed.
- Y.-K. Lu and X.-P. Yan, Anal. Chem., 2004, 76, 453–457 CrossRef CAS PubMed.
- L. Bois, A. Bonhommé, A. Ribes, B. Pais, G. Raffin and F. Tessier, Colloids Surf., A, 2003, 221, 221–230 CrossRef CAS.
- V. Kumar, M. Kashyap, S. Gautam, P. Shukla, K. B. Joshi and V. Vinayak, J. Biosci., 2018, 43, 717–729 CrossRef CAS PubMed.
- J. Lei, X. Li, W. Li, F. Sun, D. Lu and Y. Lin, J. Solid State Electrochem., 2012, 16, 625–632 CrossRef CAS.
- S. Azadi, A. Karimi-Jashni, S. Javadpour and L. Mahmoudian-Boroujerd, Environ. Dev. Sustainability, 2021, 23, 6047–6065 CrossRef.
- V. Vinayak, K. M. Manoylov, H. Gateau, V. Blanckaert, J. Hérault, G. H. Pencréac, J. Marchand, R. Gordon and B. Schoefs, Mar. Drugs, 2015, 13, 2629–2665 CrossRef CAS PubMed.
- M. J. Khan, N. Bawra, A. Verma, V. Kumar, A. Pugazhendhi, K. B. Joshi and V. Vinayak, Bioresour. Technol., 2021, 319, 124129 CrossRef CAS PubMed.
- K. Herbach, F. Stintzing and R. Carle, J. Food Sci., 2004, 69, C491–C498 CrossRef CAS.
- R. Ramanarayanan, P. Nijisha, C. V. Niveditha and S. Sindhu, Mater. Res. Bull., 2017, 90, 156–161 CrossRef CAS.
- N. I. Beedri, S. A. A. R. Sayyed, S. R. Jadkar and H. M. Pathan, AIP Conf. Proc., 2017, 1832, 040022 CrossRef.
- Z. Hu, Y. Li, M. Sommerfeld, F. Chen and Q. Hu, Eur. J. Phycol., 2008, 43, 365–376 CrossRef CAS.
- D. V. Pogozhev, M. J. Bezdek, P. A. Schauer and C. P. Berlinguette, Inorg. Chem., 2013, 52, 3001–3006 CrossRef CAS PubMed.
- V. Hong and R. E. Wrolstad, J. Agric. Food Chem., 1990, 38, 708–715 CrossRef CAS.
- J. B. Harborne, Biochem. J., 1958, 70, 22–28 CrossRef CAS.
- D. L. Macuvele, G. Z. Sithole, K. Cesca, S. L. Macuvele and J. V. Matsinhe, Environ. Sci. Pollut. Res. Int., 2016, 23, 11639–11644 CrossRef CAS PubMed.
- A. Ahirwar, G. Meignen, M. J. Khan, V. Sirotiya, M. Scarsini, S. Roux, J. Marchand, B. Schoefs and V. Vinayak, Bioresour. Technol., 2021, 340, 125707 CrossRef CAS.
- H. Sun, Q. Kong, Z. Geng, L. Duan, M. Yang and B. Guan, Bioresource technology, 2015, 186, 67–73 CrossRef CAS PubMed.
- Y. Nakamura, M. Hidaka, H. Masaki, H. Seto and T. Uozumi, Agric. Biol. Chem., 1990, 54, 3345–3346 CAS.
- S. S. L. Priya, P. R. Devi, P. Eganathan and J. Kingsley, Afr. J. Pharm. Pharmacol., 2013, 7, 1719–1728 CrossRef.
- K. Chekanov, D. Litvinov, T. Fedorenko, O. Chivkunova and E. Lobakova, Biology, 2021, 10, 643 CrossRef CAS PubMed.
- P. Suganyadevi, M. Saravanakumar, K. Aravinthan, A. Arunkumar, R. K. Krishna and S. Karthikeyani, J. Pharm. Res., 2010, 3, 2693–2696 CAS.
- D. Monchaud, J. Lacour, C. Coudret and S. Fraysse, J. Organomet. Chem., 2001, 624, 388–391 CrossRef CAS.
- A. Younas, S. Kiran, S. Abrar, S. Javed and T. Gulzar, Pol. J. Environ. Stud., 2022, 31, 5945–5954 CrossRef CAS.
- H. A. Pantami, M. S. Ahamad Bustamam, S. Y. Lee, I. S. Ismail, S. M. Mohd Faudzi, M. Nakakuni and K. Shaari, Mar. Drugs, 2020, 18, 367 CrossRef CAS.
- M. S. A. Bustamam, H. A. Pantami, A. Azizan, K. Shaari, C. C. Min, F. Abas, N. Nagao, M. Maulidiani, S. Banerjee and F. Sulaiman, Mar. Drugs, 2021, 19, 139 CrossRef CAS PubMed.
- R. Ranga, A. R. Sarada, V. Baskaran and G. A. Ravishankar, J. Microbiol. Biotechnol., 2009, 19, 1333–1341 CAS.
- C. Jeffryes, J. Campbell, H. Li, J. Jiao and G. Rorrer, Energy Environ. Sci., 2011, 4, 3930–3941 RSC.
- D. Zhang, Y. Wang, J. Cai, J. Pan, X. Jiang and Y. Jiang, Chin. Sci. Bull., 2012, 57, 3836–3849 CrossRef CAS.
- C. Jeffryes, T. Gutu, J. Jiao and G. L. Rorrer, J. Mater. Res., 2008, 23, 3255–3262 CrossRef CAS.
- S. Ito, T. N. Murakami, P. Comte, P. Liska, C. Grätzel, M. K. Nazeeruddin and M. Grätzel, Thin Solid Films, 2008, 516, 4613–4619 CrossRef CAS.
- K.-H. Park, H.-B. Gu, E. M. Jin and M. Dhayal, Electrochim. Acta, 2010, 55, 5499–5505 CrossRef CAS.
- S. Gupta, M. Kashyap, V. Kumar, P. Jain, V. Vinayak and K. B. Joshi, J. Mol. Liq., 2018, 249, 600–608 CrossRef CAS.
- Y. Qin and Q. Peng, Int. J. Photoenergy, 2012, 2012, 1–21 CrossRef.
|
This journal is © The Royal Society of Chemistry 2023 |