DOI:
10.1039/D3RA02890A
(Paper)
RSC Adv., 2023,
13, 23158-23168
Efficacy of modified carbon molecular sieve with iron oxides or choline chloride-based deep eutectic solvent for the separation of CO2/CH4
Received
2nd May 2023
, Accepted 15th July 2023
First published on 1st August 2023
Abstract
It is necessary to separate CO2 from biogas to improve its quality for the production of biomethane. Herein, an improvement in the separation of CO2/CH4 via adsorption was achieved by modifying the surface of CMS. The surface modification of CMS was performed by impregnation with metal oxide (Fe3O4) and N-doping (DES–[ChCl:Gly]). Subsequently, the efficacy of the surface-modified CMS was investigated. This involved CMS modification, material characterization, and performance analysis. The uptake of CO2 by CMS–DES–[ChCl:Gly] and CMS–Fe3O4 was comparable; however, their performance for the separation of CO2/CH4 was different. Consequently, CMS–DES–[ChCl:Gly] and CMS–Fe3O4 exhibited ca. 1.6 times enhanced CO2 uptake capacity and ca. 1.70 times and 1.55 times enhanced CO2/CH4 separation, respectively. Also, both materials exhibited similar repeatability. However, CMS–DES–[ChCl:Gly] was more difficult to regenerate than CMS–Fe3O4, which is due to the higher adsorption heat value of the former (59.5 kJ).
1 Introduction
Presently, biogas and biomethane are considered to be alternative energy sources to fossil fuels and natural gas, which are derived from renewable resources. However, the composition of biogas is generally characterized by the presence of CH4 (50–70%) and CO2 (30–50%),1,2 and thus the CO2/CH4 gas mixture must be separated to obtain a more energy-dense product, given that biomethane is a very calorific gas. In contrast, CO2 has no calorific value.3 Currently, membrane separation, absorption, and cryogenic separation are accessible technologies for eliminating carbon dioxide.4,5 However, they are expensive and consume a large amount of energy. In the case of CO2/CH4 separation, adsorption-based separation has been proposed due to its high purity (>98% vol), ease of operation, and high energy efficiency.6 There is a difference in the affinities and diffusivities of CO2 and CH4, resulting in separation through adsorption.7 Molecular sieves, such as metal–organic frameworks (MOFs),8–12 silica-derived molecular sieves (ZMSs),13–17 and carbon-derived molecular sieves (CMSs)18–22 are frequently utilized for adsorption-based separation. Generally, carbon-derived molecular sieves are durable at low adsorption temperatures and may be synthesized using coal,23,24 polymers,25–27 and biomass.18,28–30 Furthermore, it can be readily regenerated.
CMS can be modified by altering their pores (pyrolysis, partial gasification, and pore constriction) and their surfaces (functionalization and metal impregnation). Furthermore, CMS can be homogeneous regarding their pore size by tuning their pores. Nevertheless, it is quite challenging to achieve a uniform pore size. In contrast, surface modification aims to enhance the affinity of CO2 for carbon surfaces. In addition to the uniformity of the pores in CMS, the diffusivity of molecules in their pores is also affected by their affinity with the surface of CMS. Increasing the affinity of a molecule for the surface of CMS inhibits the mobility (diffusion) of the molecule in the pores.
In CO2/CH4 separation with surface modification, functional groups such as nitrogen, amines, and oxygen are usually added to CMS to increase their affinity for CO2.31 Also, their surface can be modified by impregnating them with metal oxides. In this case, several metal oxides have been used as CO2 adsorbents, such as MgO, CaO, Fe3O4, CuO and NiO. The surface modification is based on the presence of CO2, which has an affinity for basic metal oxides, whereas CH4 does not. As a result of these differences, CO2 can be removed from gas mixtures by utilizing the interaction between the adsorbate and adsorbent. Thus, the presence of basicity may enhance the surface affinity of CMS for CO2.32 In general, the presence of metal oxides33 and functional groups such as oxygen and nitrogen31 is considered to increase the affinity of CMS for CO2. CO2 adsorption may also occur on materials with open metal oxide sites, specific functional groups, and charge species. As a result of the higher polarizability of CO2, higher amounts of CO2 are adsorbed than CH4 at ambient temperatures. Therefore, it can be separated from gas mixtures at this temperature.34
Recent research has focused on enhancing activated carbon via surface modification to increase its affinity for CO2. Given that CO2 is a weak Lewis acidic gas, modifying the surface of activated carbon with basic groups or reacting it with N-containing compounds can generate more active functional sites for CO2, enhancing the affinity for CO2. For instance, Masruroh et al.21 suggested that functionalizing the surface of activated carbon with amine groups such as monoethanolamine (MEA), 2-amino-2-methyl-1-propanol (AMP), and diethanolamine (DEA) is advantageous for improving the surface chemistry, which provides a higher affinity, thereby enhancing its CO2/CH4 separation performance. The results indicated that CMS–MEA exhibited the best performance for the separation of CO2 from CH4. In the investigation by Mukti et al.,22 an improved iron oxide dispersion on oxidized carbon surfaces could separate CO2/CH4. In this respect, Fe3O4 is more desirable for separating CO2/CH4 than Fe2O3. An additional study was conducted by Schott et al.35 on activated mesoporous carbon (AMC), which was modified with metal oxides (CeO2, CuO, Mn3O4, and NiO) to improve its CO2/N2 selectivity. Compared to the parent AMC, the selectivity values of the AMC modified with metal oxides were generally higher, with a 72% improvement in the selectivity of the 30% NiO-modified AMC. In addition, Shahkarami et al.36 found that MgO-impregnated activated carbon improved both the CO2 adsorption and CO2/N2 selectivity.
In addition, numerous studies investigated the possibility of transforming activating agents from chemical solvents to green solvents to increase the affinity of carbon for CO2, such as adding a deep eutectic solvent (DES) to its surface. For example, a choline chloride-based DES exhibited a strong electrostatic interaction with CO2, contributing to an increase in the affinity for it. Ariyanto et al.20 investigated the possibility of modifying carbon surfaces using a choline chloride-based DES to separate CO2/CH4. In their study, three types of alcohol were, i.e., 1-butanol (-ol), ethylene glycol (-diol), and glycerol (-triol), were combined with choline chloride to form a DES as a surface modifier. Among them, the DES containing the triol of glycerol displayed the best performance. Hussin et al.37 compared the impregnation of a deep eutectic solvent (DES) from a mixture of choline hydroxide
:
urea and choline hydroxide
:
glycerol on carbon to enhance the CO2 adsorption capacity. The highest CO2 adsorption efficiency was observed for the DES-based activated carbon obtained using a mixture of choline hydroxide
:
urea at a temperature of 25 °C. The modified adsorbent still demonstrated an excellent performance after 11 adsorption cycles and desorption.
In the present study, we synthesized molecular sieves to separate CO2/CH4 by impregnating porous carbon surfaces with Fe3O4 or DES–[ChCl:Gly]. The procedure for the preparation of CMS involved an oxidation process, followed by an impregnation process using Fe3O4 or DES–[ChCl:Gly]. In this study, we investigated different surface modifications and the corresponding separation performance. To the best of our knowledge, this has not been reported to date. The efficacy of DES–[ChCl:Gly] and Fe3O4 impregnation was assessed by examining the adsorption isotherms, breakthrough analysis, repeatability performance, and regeneration of the samples. Furthermore, their surface morphology, surface properties, elemental composition, and chemical properties were evaluated using SEM-EDX mapping, FTIR, and nitrogen sorption analysis.
2 Experimental
2.1. Materials
The materials used included porous carbon with a mesh size of 20–25, ferrous(III) nitrate (Merck), H2O2 (Merck), choline chloride (Sigma-Aldrich), glycerol (Sigma-Aldrich), methanol (Merck), HP-nitrogen, UHP-carbon dioxide and UHP-methane from PT Aneka Gas Industri Indonesia. A biogas representative comprised of CH4 and CO2 (55/45 v/v) was obtained from PT Aneka Gas Industri Indonesia.
2.2. Modification of CMS
Pristine carbon with a mesh size of 20–25 was pre-treated with 10% H2O2 to increase its surface wettability. In the next step, pre-treated carbon was impregnated with Fe3O4 and DES–[ChCl:Gly]. The metal oxides (Fe3O4) were dispersed on pre-treated carbon using the incipient wetness method and calcined. Before impregnation, the metal salt (Fe(NO3)3·9H2O) was dissolved in methanol. The metal salt solution in methanol was gradually added to the carbon pores in the following stages. The use of carbon and methanol in a w/v ratio of 1
:
1 was proposed to achieve a metal oxide content of 5 wt% (Fe). In the subsequent step, the carbon was impregnated with metal salt, and then calcined at 773 K for 6 h. Information regarding the impregnation procedures can be found in the literature.22
DES–[ChCl:Gly] impregnation on pretreated carbon was performed in accordance with ref. 20. Choline chloride and glycerol were mixed in distilled water in a mol ratio of 0.5
:
0.5 to prepare a 5 wt% DES–[ChCl
:
Gly] solution. In the following step, the DES–[ChCl:Gly] solution was gradually added to the carbon pores, and then dried at 105 °C overnight. Consequently, the carbon that was impregnated with the metal oxide and DES–[ChCl:Gly] was labeled CMS–Fe3O4 and CMS–DES–[ChCl:Gly], respectively. The modification of CMS is schematically illustrated in Fig. 1.
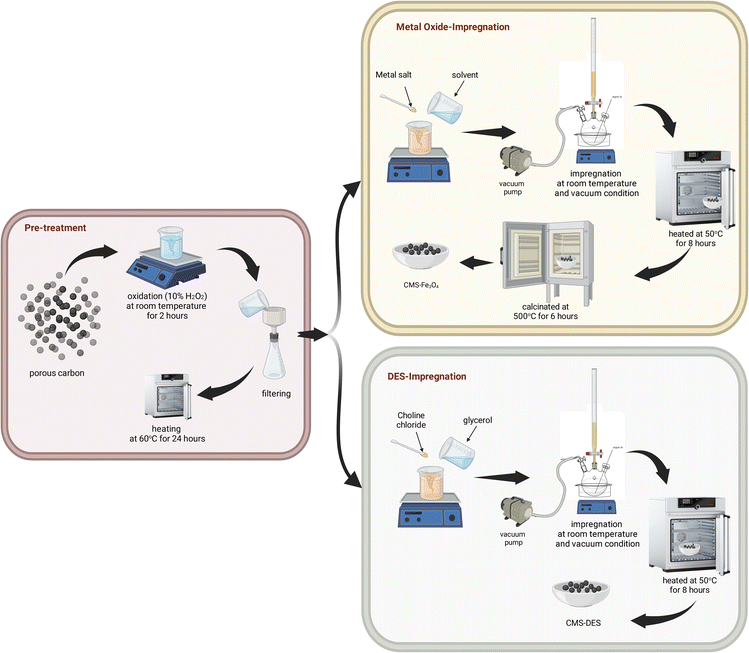 |
| Fig. 1 Schematic of CMS modification procedure. | |
2.3. Characterization of CMS
The functional groups present in the CMS materials were characterized by infrared Fourier transform analysis (FTIR) using a Nicolet Avatar 360 IR in the wavelength range of 400–4000 cm−1. The morphology of CMS was examined using SEM-EDX imaging with a JEOL JSM6510 LA at 10 kV. A Quantachrome NOVA 2000 was used to record the nitrogen sorption isotherms at 77 K. The Brunauer–Emmett–Teller (BET) method was utilized to determine the surface area in the relative pressure range of 0.05 to 0.25. The total pore volume, denoted by Vt, was computed with the help of the nitrogen sorption isotherm at a relative pressure of P/P0 = 0.99. The volume of micropores (Vm) and volume of the total pore system (Vt) were calculated using the T-plots. The pore size distributions (PSDs) in this study were determined by density functional theory (DFT) analysis.
2.4. Measurement of adsorption isotherms
The adsorption capacity measurements for CO2 and CH4 were conducted at 303 K using the volumetric method. A Swagelok® VCR valve and fitting were used to assemble an ultrahigh vacuum adsorption apparatus. As a precondition for the adsorption measurements, the CMS sample was degassed at a temperature of 423 K for 6 h under vacuum. This was conducted to eliminate any remaining water and surface species. The adsorption isotherms were measured in the range of 0 to 5.0 atm and the adsorption isotherm curves were depicted using the obtained information. Detailed schematic diagrams of the adsorption isotherms and procedures for their measurements are described in the literature.38
2.5. Evaluation of breakthrough curves
Gas mixtures of CH4 and CO2 were detected using a portable biogas analyzer (Gas Board 3200 plus, Hubei Cubic Ruiyi Instrument Co., Ltd.). The CMS material was loaded in a fixed bed column (d = 0.95 cm and length = 30 cm), and 0.2 L min−1 of nitrogen gas was injected to remove CH4, CO2, and O2 from the bed. The CH4–CO2 gas mixture entered the system at a rate of 0.05 L min−1 at a temperature of 303 K and pressure of 1.2 bar. After completing the separation process, the gas composition returned to its initial concentration. The detailed procedure and breakthrough system for the separation of CO2/CH4 are described in the literature.20,22
3 Results and discussion
3.1. Structural and surface characterization of CMSs
Surface morphologies and functional groups. Fig. 2(a, b and d) display the SEM images of C, CMS–DES–[ChCl:Gly], and CMS–Fe3O4, and Fig. 2(c and e) show the elemental mappings of CMS–DES–[ChCl:Gly] and CMS–Fe3O4, respectively, where differences in the surface morphology between the pristine carbon and CMSs can be observed. According to Fig. 2(a, b, and d), CMSs have more cavities than pristine carbon. It is also evident from the elemental mapping displayed in Fig. 2(c and e) that Fe3O4 and DES–[ChCl:Gly] were evenly distributed on the surface of the carbon. An analysis of the SEM-EDX results was performed to assess the composition of the CMSs. Table 1 provides a detailed analysis of the results.
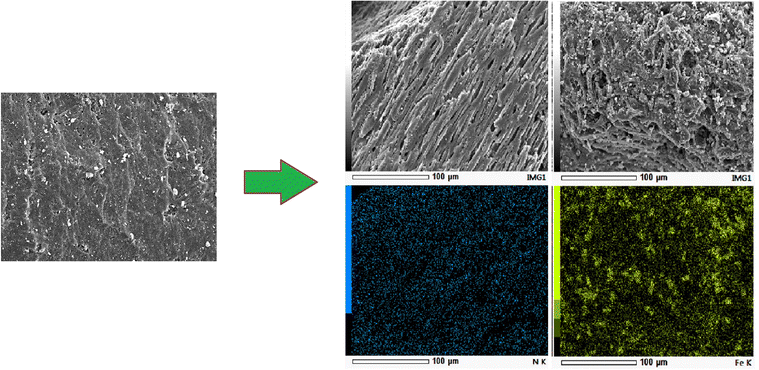 |
| Fig. 2 SEM images and their elemental mapping of C (a), CMS–DES–[ChCl]:Gly (b and c) and CMS–Fe3O4 (d and e). | |
Table 1 The elements detected by EDX analysis on CMSs
Material |
Element, % |
pHPZC |
C |
O |
N |
Cl |
Fe |
C |
93.06 |
6.94 |
— |
— |
— |
6.93 |
CMS–DES–[ChCl:Gly] |
77.88 |
16.55 |
5.25 |
0.32 |
— |
7.21 |
CMS–Fe3O4 |
83.47 |
10.57 |
— |
— |
5.96 |
7.29 |
The modification process involving iron oxide impregnation and DES–[ChCl:Gly] could alter the surface chemistry of CMS, where the addition of DES–[ChCl:Gly] and iron oxide to CMS increased its basicity. This was evidenced by the increase in the pHPZC value, as shown in Table 1. The pHPZC value also increased for the composite containing iron oxide and activated carbon nanoparticles.39,40 This basicity favours CO2 adsorption. As the basicity increased, the CO2 affinity increased, while the CH4 affinity decreased. In Table 1, it is also important to note that N, O, and Cl functional groups were added in the case of DES–[ChCl:Gly] impregnation. The presence of N, O, H, and Cl will increase the affinity for CO2.
The incorporation of Fe3O4 and DES–[ChCl:Gly] in the porous carbon was confirmed by FTIR spectroscopy (Fig. 3). In the pristine carbon and CMSs, the peaks at 1080 cm−1, 1590 cm−1, and 3460 cm−1 are associated with the C–O groups (stretching),41 carboxyl groups (C
O stretching),41,42 and hydroxyl groups (O–H stretching),42,43 respectively. In the spectrum of CMS–DES–[ChCl:Gly], the sharp peak observed at 3440 cm−1 is a result of the overlap in the O–H stretch band and the N–H stretch band.44 The FTIR spectrum of chitosan also revealed the overlap of the O–H and N–H peaks.45 Also, DES–[ChCl:Gly] was detected on the surface of carbon based on the N–H bending peak at 1640 cm−1.20 CMS–Fe3O4 exhibited an additional peak at 590 cm−1, indicating the presence of Fe–O bonds.22
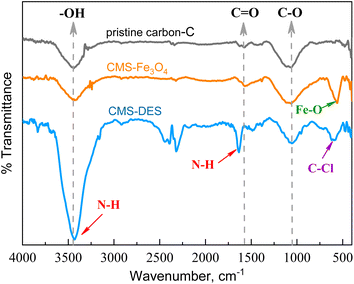 |
| Fig. 3 FTIR spectra of C, CMS–Fe3O4 and CMS–DES–[ChCl]:Gly. | |
Nitrogen sorption analysis. A nitrogen sorption analysis was conducted at 77 K to characterize the textural properties of pristine carbon and CMSs, and their comparison is illustrated in Fig. 4. Based on the IUPAC classification, carbon and CMS are classed as microporous materials with a type I isotherm (Fig. 4(a)). At 0.1 P/P0, the pristine carbon exhibited a high nitrogen adsorption volume (ca. 140 cm3 g−1 STP). The volume of nitrogen adsorbed decreased significantly with CMS–DES–[ChCl:Gly], whereas it decreased modestly with CMS–Fe3O4. Interestingly, the adsorption–desorption isotherm curve for CMS–DES–[ChCl:Gly] is unclosed, which is probably due to the presence of ink bottle-shaped pores following impregnation.20 Fig. 4(b) shows the results of the pore size distribution evaluated using the QSDFT model based solely on the adsorption data. The pristine carbon content was estimated to be 92 vol% in the micropore region (<2 nm), with a small tail of 4–5 nm. There was a decrease in porosity in the micropore region of CMS–DES–[ChCl:Gly] and CMS–Fe3O4. CMS–Fe3O4 exhibited a shift to <2 nm with a small tail of 2–3 nm, while CMS–DES–[ChCl:Gly] exhibited a shift to <2 nm region. A few of the small pores appeared to be blocked by DES–[ChCl:Gly] and Fe3O4. The pores smaller than 1 nm may become blocked completely, while that wider than 2 nm may experience a reduction in size. This can be attributed to the attachment of DES–[ChCl:Gly] and iron oxide to the carbon surface. Therefore, in the PSDs, it can be seen that the size of <2 nm was still present, while the size of >3 nm was absent.
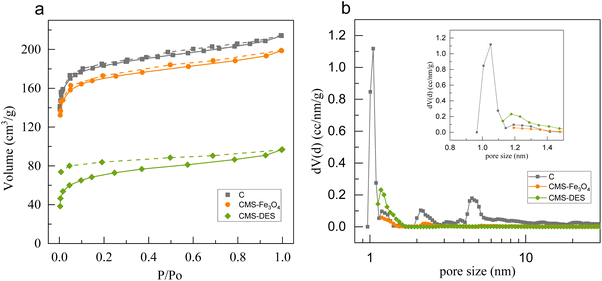 |
| Fig. 4 Isotherms for nitrogen sorption (a) and distribution of pore sizes (b) for CMSs. | |
The textural properties of CMSs are presented in Table 2. In comparison to pristine carbon, CMS–DES–[ChCl:Gly] has a lower specific surface area by approximately 64%, whereas that of CMS–Fe3O4 decreased by approximately 8%. The impregnation of DES–[ChCl:Gly] resulted in the blockage of the pores and the collapse of the pore walls.20,46,47 However, regardless of their surface area or pore volume, all the materials are microporous.
Table 2 Textural properties of CMSs based on nitrogen sorption
Material |
SBET, m2 g−1 |
% Smic |
V, cm3 g−1 |
Davg, nm |
C |
708 |
92.0 |
0.33 |
1.88 |
CMS–Fe3O4 |
649 |
92.9 |
0.31 |
1.90 |
CMS–DES–[ChCl:Gly] |
258 |
86.8 |
0.15 |
2.33 |
3.2. Carbon dioxide and methane adsorption isotherms
As shown in Fig. 5(a and b), CO2 and CH4 adsorption isotherm curves were measured at 30 °C. As shown in Fig. 5(a and b), the CO2 uptake significantly exceeded CH4 uptake. Similar results were obtained in a previous study.20,22 Moreover, the impregnation of Fe3O4 and DES–[ChCl:Gly] increased the CO2 uptake, while reducing CH4 uptake. In Fe3O4 and DES–[ChCl:Gly], the active sites are more favourable for the adsorption of CO2, resulting in an increase in CO2 capacity. In contrast, the carbon impregnated with Fe3O4 and DES–[ChCl:Gly] reduced the affinity for CH4. Thus, the selectivity for CO2/CH4 was enhanced, resulting in the better removal of CO2 from CO2–CH4 mixed gases. The CO2 uptake by pristine carbon was 1.7 mol kg−1 at 1 atm, whereas that by CMS–Fe3O4 and CMS–DES–[ChCl:Gly] was 2.4 and 2.5 mol kg−1, respectively. This drastically improved the CO2 uptake, which can be attributed to factors, i.e., CO2 binding Fe3O4 or DES–[ChCl:Gly] functional groups in CMS and well-dispersed Fe3O4 and DES–[ChCl:Gly] throughout the porous carbon. Meanwhile, the uptake of pristine carbon, CMS–Fe3O4, and CMS–DES–[ChCl:Gly] for CH4 was 1.1 mol kg−1, 0.75 mol kg−1, and 0.76 mol kg−1, respectively.
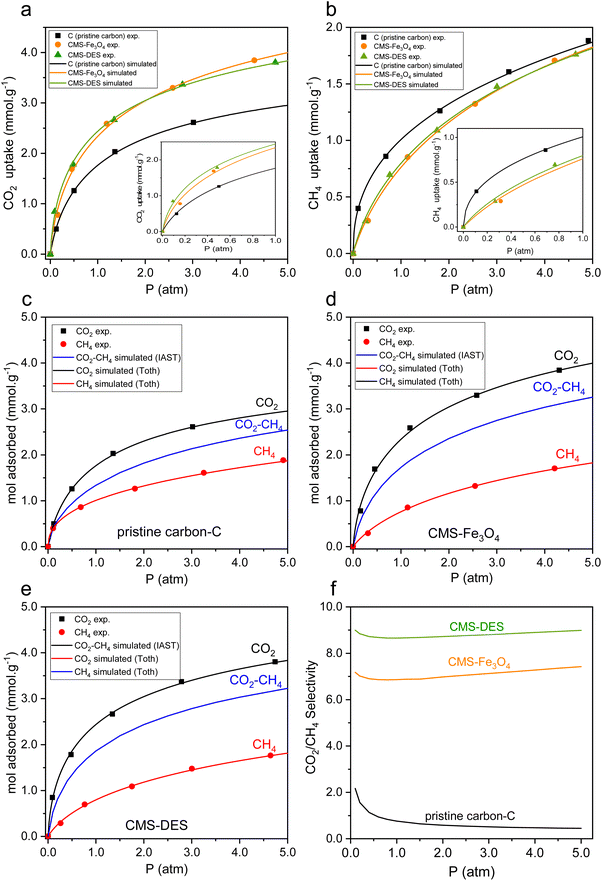 |
| Fig. 5 Isotherm adsorption of CO2 (a) and CH4 (b) on pristine carbon–C, CMS–Fe3O4 and CMS–DES–[ChCl]:Gly, adsorption isotherm of CO2 and CH4 on pristine carbon–C (c), CMS–Fe3O4 (d) and CMS–DES–[ChCl]:Gly (e) (isotherm data fitted with the Toth equilibrium model and IAST model used in simulative adsorption of CO2–CH4 (55 : 45) on pristine carbon–C, CMS–Fe3O4 and CMS–DES–[ChCl]:Gly), sorption selectivity of CO2/CH4 at a temperature of 303 K (f). | |
The equilibrium parameters were determined using the Toth adsorption model (eqn (1)).
|
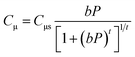 | (1) |
where
Cμ is the uptake capacity,
Cμs is the maximum uptake capacity, and
b and
t are the equilibrium constants.
Table 3 presents the parameters of the Toth model for CO
2 and CH
4 adsorption in CMSs. Based on the
b value (adsorption affinity), it is clear that CO
2 is more favorably adsorbed on the CMSs than CH
4. Further, based on the
b value, it can be concluded that the modified CMS exhibited an increased affinity for CO
2. In contrast, the affinity of CH
4 decreased. As shown in
Table 3, the
b values of CO
2 for pristine carbon, CMS–Fe
3O
4, and CMS–DES–[ChCl:Gly] are 1.53, 2.10, and 3.99, respectively.
Table 3 Equilibrium parameters obtained from the fitting isotherm data using the Toth model
Sample |
Adsorbate |
Cμs, (mmol g−1) |
b (atm) |
t |
C |
CO2 |
4.47 |
1.53 |
0.62 |
CH4 |
3.96 |
0.81 |
0.08 |
CO2–CH4 |
9.09 |
1.61 |
0.32 |
CMS–Fe3O4 |
CO2 |
7.70 |
2.10 |
0.45 |
CH4 |
4.71 |
0.33 |
0.59 |
CO2–CH4 |
7.13 |
1.13 |
0.47 |
CMS–DES–[ChCl:Gly] |
CO2 |
6.73 |
3.99 |
0.43 |
CH4 |
5.09 |
0.41 |
0.51 |
CO2–CH4 |
6.51 |
2.02 |
0.43 |
Subsequently, it was possible to predict the simultaneous adsorption of CO2 and CH4 on CMSs using the equilibrium adsorption of the single components of CO2 and CH4 on CMSs (written as CO2–CH4). The ideal adsorption solution theory (IAST) approximates the equilibrium equations for multicomponent systems by applying eqn (3) and (4), where the total amount absorbed can be determined. Fig. 5(c–e) present the calculation results for CO2–CH4 multicomponent adsorption based on the IAST model. The composition of the CO2–CH4 adsorption is 45%
:
55%. Based on the IAST equation for CO2–CH4 adsorption, the maximum adsorption capacity was smaller than that for single CO2 components. The results indicate competition for the interaction between CH4 and CO2 on CMSs. CO2 is more readily adsorbable than CH4, depending on the b values of the single components in each CMS. This is also evident from the CO2–CH4 curve, which tends to be more closely related to CO2.
|
 | (3) |
The separation of CO2/CH4 depends heavily on the selectivity of CO2/CH4. Fig. 5(f) illustrates the selectivity of CO2/CH4. In accordance with the studies in the literature, the selectivity of pristine carbon decreased with an increase in pressure, as evidenced by the data.20,48 CO2/CH4 had a selectivity of ca. 2.3–0.5 on the pristine carbon in the pressure range studied. Compared to pristine carbon, CMS DES–[ChCl:Gly] and CMS–Fe3O4 exhibited improved selectivity for CO2/CH4. The CO2/CH4 selectivity increased by 3.3 times on CMS–Fe3O4, while that on CMS–DES–[ChCl:Gly] increased by 3.9 times. CMS–DES–[ChCl:Gly] provides almost as much CO2 uptake as CMS–Fe3O4 despite its lower surface area. Additionally, CMS–DES–[ChCl:Gly] also provided higher CO2/CH4 selectivity. In addition to surface area, it appears that the affinity for the active site (DES–[ChCl:Gly] or Fe3O4) influenced the CO2 uptake. A more favorable affinity is the basis for improving the separation of CO2/CH4, given that it will inhibit CO2 diffusion in the pores, but not CH4 diffusion. Thus, as the diffusivity ratio increases, the separation of CO2/CH4 will be improved.
Adsorption studies on gases consider the heat of adsorption (ΔHads) as a significant variable. It explains how adsorbents interact with their adsorbates. Employing the Van't Hoff equation (eqn (4)), it is possible to determine the heat of adsorption (ΔHads) of CO2 and CH4 on pristine carbon and both CMSs. The heat of adsorption was calculated in Henry's region, provided at a zero-coverage limit.
|
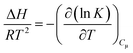 | (4) |
where Δ
Hads is the heat of adsorption (J mol
−1),
K is the Henry constant (dimensionless),
T is the temperature (K), and
R is the ideal gas constant (J (mol K)
−1). A comparison of the CO
2 and CH
4 heat adsorption on pristine carbon, CMS–DES–[ChCl:Gly], and CMS–Fe
3O
4 is shown in
Table 4. The results show a lower CO
2 adsorption energy in pristine carbon than in CMS–DES–[ChCl:Gly] and CMS–Fe
3O
4. In terms of heat of adsorption, the mechanism on pristine carbon and CMS–Fe
3O
4 is physisorption, while that on CMS–DES–[ChCl:Gly] is chemisorption. Hence, the CO
2 interactions with CMS–DES–[ChCl:Gly] are stronger than that between the pristine carbon and CMS–Fe
3O
4. This may be attributed to the fact that electrostatic interactions are responsible for the strong interactions between CMS–DES–[ChCl:Gly] and CO
2. The interactions that may occur include those involving oxygen in CO
2 with N
+ in choline, oxygen in CO
2 with H bonds from glycerol, and carbon in CO
2 with Cl
−. The presence of
n-functionalization on carbon has also been shown to interact strongly with CO
2 in other studies.
49 Table 5 provides a comparison of the adsorption heat values from various sources.
Table 4 Heat of adsorption and Henry constants for CH4 and CO2 in CMSs
Adsorbate |
Material |
ΔHads (kJ mol−1) |
T (K) |
Henry constant, Kpa |
Henry constant, Kb |
gmol (g atm)−1. Dimensionless, K = (KpρpRT)/(1 − ε). |
CO2 |
C |
10.9 |
303 |
3.4 × 10−3 |
259 |
313 |
8.4 × 10−4 |
65 |
323 |
1.9 × 10−4 |
16 |
CMS–Fe3O4 |
16.4 |
303 |
3.7 × 10−3 |
283 |
313 |
1.8 × 10−3 |
140 |
323 |
6.5 × 10−4 |
52 |
CMS–DES–[ChCl:Gly] |
59.5 |
303 |
5.2 × 10−3 |
394 |
313 |
3.8 × 10−3 |
293 |
323 |
2.1 × 10−3 |
172 |
CH4 |
C |
9.8 |
303 |
8.0 × 10−4 |
60 |
313 |
4.1 × 10−4 |
33 |
323 |
3.3 × 10−4 |
29 |
CMS–Fe3O4 |
10.6 |
303 |
9.5 × 10−4 |
72 |
313 |
7.9 × 10−4 |
62 |
323 |
6.1 × 10−4 |
49 |
CMS–DES–[ChCl:Gly] |
10.3 |
303 |
7.7 × 10−4 |
58 |
313 |
6.8 × 10−4 |
53 |
323 |
5.9 × 10−4 |
48 |
Table 5 The heat of adsorption and amount of CH4 and CO2 adsorbed in other CMSs
Adsorbate |
Material |
SSABET, (m2 g−1) |
Mol adsorbed (mmol g−1) (1 atm, 303 K) |
ΔHads (kJ mol−1) |
Ref. |
CO2 |
C |
708 |
1.85 |
10.9 |
This study |
CMS–Fe3O4 |
649 |
2.94 |
16.4 |
This study |
CMS–DES–[ChCl:Gly] |
258 |
2.85 |
59.5 |
This study |
AC–Fe2O3 |
212 |
1.10 |
33.2 |
50 |
AC–Co3O4 |
185 |
1.60 |
28.1 |
50 |
Porous carbon–La2O3 |
715 |
1.47 |
33.4 |
51 |
S-doped microporous carbon |
729 |
2.46 |
22.0 |
52 |
N-doped microporous carbon |
614 |
4.04 |
59.3 |
31 |
AC–MgO |
615 |
2.19 |
— |
36 |
CH4 |
C |
708 |
1.08 |
9.8 |
This study |
CMS–Fe3O4 |
649 |
0.85 |
10.6 |
This study |
CMS–DES–[ChCl:Gly] |
258 |
0.64 |
10.3 |
This study |
AC–Fe2O3 |
212 |
0.30 |
20.2 |
50 |
AC–Co3O4 |
185 |
0.40 |
22.0 |
50 |
3.3. CO2/CH4 separation: a breakthrough curve analysis
The performance of the materials in separating CO2/CH4 was evaluated through breakthrough analysis. Fig. 6(a) depicts the CO2 breakthrough curves for pristine carbon and CMSs. The breakthrough curves demonstrate the ratio of the outlet concentration at a given time (Ct) to the initial inlet concentration (C0) over time. It is evident from the data that the CO2 breakthrough time for CMS–DES–[ChCl:Gly] and CMS–Fe3O4 increased compared to pristine carbon. CMSs exhibited an increase in breakthrough time by 40% compared to the pristine carbon. Due to this strong interaction between CO2 and CMSs, the CO2 diffusion is slower. Both CMS–DES–[ChCl:Gly] and CMS–Fe3O4 showed similar breakthrough times. Interestingly, CMS–Fe3O4 had a steeper breakthrough curve than CMS–DES–[ChCl:Gly]. This suggests that CMS–DES–[ChCl:Gly] has a stronger interaction with CO2 than CMS–Fe3O4. Furthermore, other studies suggest that nitrogen-doped carbon exhibits slow adsorption kinetics.33 In contrast, studies on pristine carbon and CMS–Fe3O4 indicated that mass transfer occurs instantaneously.20,22
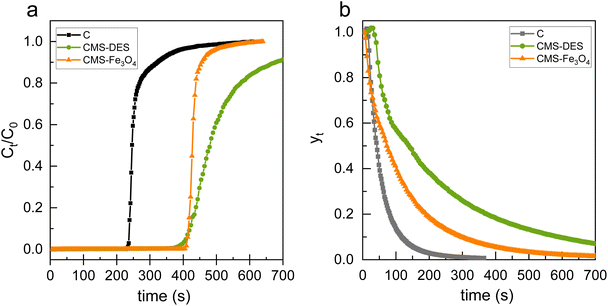 |
| Fig. 6 CO2 breakthrough curves of mixed gas CO2/CH4 for CMS (a). Desorption curves for CMS (b) at a temperature of 303 K and atmospheric pressure. | |
During the separation of CO2/CH4, the ability to regenerate the absorbent is an imperative factor. Fig. 6(b) illustrates a regeneration graph for pristine carbon and CMSs. The data indicate that the regeneration time of CMS–DES–[ChCl:Gly] is longer than that of CMS–Fe3O4 and pristine carbon. Therefore, CMS–DES–[ChCl:Gly] will be more difficult to regenerate due to its strong interaction with CO2. Furthermore, this conclusion is supported by the results of the adsorption heat value of CMS–DES–[ChCl:Gly], which is quite high (59.5 kJ), as explained in Section 3.2.
Fig. 7 depicts the CO2 and CH4 breakthrough curves for the different CMS materials. Initially, neither CH4 nor CO2 gas was present, as shown in Fig. 6. CH4 with a purity of higher than 98% appeared after 200 s. This was followed by CO2 until the concentration of CO2 in the outlet equalled the concentration in the inlet. Each material had a similar CH4 breakthrough time, but a different CO2 breakthrough time. The CMS surface and gas molecules may interact strongly, causing a delay in CO2 flow at the outlet. Consequently, CO2 gas diffused more slowly within the bed. Furthermore, the difference between the CH4 signal and CO2 signal appears to be larger, indicating that CH4 and CO2 were separated more effectively.
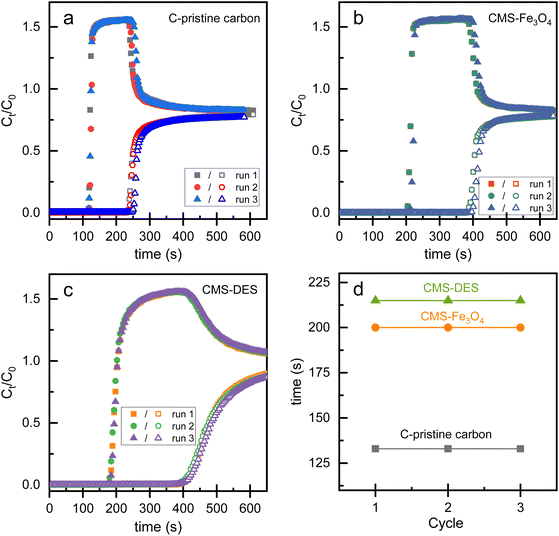 |
| Fig. 7 Breakthrough curves of mixed gas CO2/CH4 for pristine carbon (a), CMS–Fe3O4 (b), CMS–DES–[ChCl]:Gly (c),20 and repeatability of CO2/CH4 separation of CMSs (d). | |
According to Fig. 7(a), the CO2–CH4 breakthrough time difference for pristine carbon is 130 s. CMS–Fe3O4 exhibited an approximately 1.5-fold increase in CO2–CH4 breakthrough time compared to the pristine carbon (see Fig. 7(b)). Meanwhile, CMS–DES–[ChCl:Gly] experienced a 1.7-fold increase (Fig. 7(c)). In the repeatability study, the sequence of CO2/CH4 separation was cycled and the bed was regenerated once it was full of CO2. Interestingly, CMS–DES–[ChCl:Gly] and CMS–Fe3O4 maintained their breakthrough time in the three cycles. These materials performed similarly to the pristine carbon but had a much higher capacity for uptake. The cycling tests indicate that CMS–DES–[ChCl:Gly] and CMS–Fe3O4 can be repeatably used in terms of separation. Thus, it is evident that CMS–DES–[ChCl:Gly] has a wider CO2–CH4 breakthrough time difference and reasonable repeatability. Nevertheless, it requires a longer period for regeneration. Meanwhile, CMS–Fe3O4 has a shorter CO2–CH4 breakthrough time than CMS–DES–[ChCl:Gly]. Despite this, it is repeatable and regenerates quickly.
4 Conclusions
A feasibility study was conducted regarding the modification of CMS by impregnating DES–[ChCl:Gly] and Fe3O4 in the surface of porous carbon for CO2/CH4 separation. Several aspects of CO2/CH4 separation were evaluated, including CO2 breakthrough time, CO2–CH4 breakthrough time difference, regeneration capability, and repetition cycle. Additionally, the uptake of CO2 and CH4 was examined. The CO2–CH4 breakthrough time difference for CMS–DES–[ChCl:Gly] was higher than that for CMS–Fe3O4. Consequently, CMS–DES–[ChCl:Gly] and CMS–Fe3O4 exhibited an enhanced CO2 uptake capacity by ca. 60% at 30 °C and 1 atm, while enhancing the separation of CO2/CH4 by ca. 69% and 54%, respectively. The adsorption energy of CO2 in CMS–DES–[ChCl:Gly] (59.5 kJ) was higher than that of CMS–Fe3O4 (16.4 kJ), and thus a longer regeneration time was required. According to the cycling tests, CMS–DES–[ChCl:Gly] and CMS–Fe3O4 could be repeatably used. Thus, CMS–Fe3O4 is more favourable for CO2/CH4 separation than CMS–DES–[ChCl:Gly]. This is due to its repeatability and ability to be regenerated quickly.
Author contributions
Nur Indah Fajar Mukti: conceptualization, investigation, methodology, formal analysis, funding acquisition, writing-original draft. Teguh Ariyanto: supervision, funding acquisition, writing-review and editing. Wahyudi Budi Sediawan: supervision, writing-review and editing. Imam Prasetyo: conceptualization, supervision, funding acquisition, writing-review and editing.
Conflicts of interest
There are no conflicts to declare.
Acknowledgements
This research was supported by World Class Research Grant, Ministry of Education, Culture, Research, and Technology of the Republic of Indonesia, grant number 1786/UN1/DITLIT/Dit-Lit/PT.01.03/2022. N. I. F. M. acknowledges doctoral scholarship (Beasiswa Unggulan Dosen Indonesia) through Indonesia Endowment Fund for Education.
References
- I. U. Khan, M. H. D. Othman, H. Hashim, T. Matsuura, A. F. Ismail, M. Rezaei-DashtArzhandi and I. W. Azelee, Energy Convers. Manage., 2017, 150, 277–294 CrossRef
. - R. Zhang, Biogas and Fuel Cell Workshop, 2012, pp. 1–8 Search PubMed.
- M. T. Kallo and M. J. Lennox, Langmuir, 2020, 36, 13591–13600 CrossRef CAS PubMed
. - O. W. Awe, Y. Zhao, A. Nzihou, D. P. Minh and N. Lyczko, Waste Biomass Valorization, 2017, 8, 267–283 CrossRef CAS
. - X. Y. Chen, H. Vinh-Thang, A. A. Ramirez, D. Rodrigue and S. Kaliaguine, RSC Adv., 2015, 5, 24399–24448 RSC
. - I. Durán, N. Álvarez-Gutiérrez, F. Rubiera and C. Pevida, Chem. Eng. J., 2018, 353, 197–207 CrossRef
. - I. Prasetyo, R. Rochmadi and E. Wahyono, Reaktor, 2010, 13, 24–30 Search PubMed
. - W. Lou, J. Yang, L. Li and J. Li, J. Solid State Chem., 2014, 213, 224–228 CrossRef CAS
. - D. Britt, H. Furukawa, B. Wang, T. G. Glover and O. M. Yaghi, Proc. Natl. Acad. Sci. U. S. A., 2009, 106, 20637–20640 CrossRef CAS
. - W. L. Queen, M. R. Hudson, E. D. Bloch, J. A. Mason, M. I. Gonzalez, J. S. Lee, D. Gygi, J. D. Howe, K. Lee, T. A. Darwish, M. James, V. K. Peterson, S. J. Teat, B. Smit, J. B. Neaton, J. R. Long and C. M. Brown, Chem. Sci., 2014, 5, 4569–4581 RSC
. - Z. Li, P. Liu, C. Ou and X. Dong, ACS Sustainable Chem. Eng., 2020, 8, 15378–15404 CrossRef CAS
. - A. Guo, Y. Ban, K. Yang and W. Yang, J. Membr. Sci., 2018, 562, 76–84 CrossRef CAS
. - N. K. Jensen, T. E. Rufford, G. Watson, D. K. Zhang, K. I. Chan and E. F. May, J. Chem. Eng. Data, 2012, 57, 106–113 CrossRef CAS
. - M. Younas, M. Sohail, L. L. Kong, M. J. K. Bashir and S. Sethupathi, Int. J. Environ. Sci. Technol., 2016, 13, 1839–1860 CrossRef CAS
. - A. I. Sarker, A. Aroonwilas and A. Veawab, Energy Procedia, 2017, 114, 2450–2459 CrossRef CAS
. - R. Chanajaree, T. Chokbunpiam, J. Kärger, S. Hannongbua and S. Fritzsche, Microporous Mesoporous Mater., 2019, 274, 266–276 CrossRef CAS
. - W. Li, K. Goh, C. Y. Chuah and T. H. Bae, J. Membr. Sci., 2019, 588, 117220 CrossRef CAS
. - I. Prasetyo, N. I. F. Mukti, R. B. Cahyono, A. Prasetya and T. Ariyanto, Waste Biomass Valorization, 2020, 11, 5599–5606 CrossRef CAS
. - I. Prasetyo and D. D. Do, Chem. Eng. Sci., 1998, 53, 3459–3467 CrossRef CAS
. - T. Ariyanto, K. Masruroh, G. Y. S. Pambayun, N. I. F. Mukti, R. B. Cahyono, A. Prasetya and I. Prasetyo, ACS Omega, 2021, 6, 19194–19201 CrossRef CAS PubMed
. - K. Masruroh, R. B. Cahyono, I. Prasetyo and T. Ariyanto, Int. J. Renewable Energy Dev., 2021, 10, 249–255 CAS
. - N. I. F. Mukti, T. Ariyanto, W. B. Sediawan and I. Prasetyo, RSC Adv., 2021, 11, 36782–36791 RSC
. - Z. Yang, D. Wang, Z. Meng and Y. Li, Sep. Purif. Technol., 2019, 218, 130–137 CrossRef CAS
. - H. Teng, J. A. Ho, Y. F. Hsu and C. T. Hsieh, Ind. Eng. Chem. Res., 1996, 35, 4043–4049 CrossRef CAS
. - I. Prasetyo, R. Rochmadi, E. Wahyono and T. Ariyanto, Eng. J., 2017, 21, 83–94 CrossRef CAS
. - S. J. Kim, Y. Kwon, D. Kim, H. Park, Y. H. Cho, S. E. Nam and Y. I. Park, Membranes, 2021, 11, 1–34 Search PubMed
. - C. P. Hu, C. K. Polintan, L. L. Tayo, S. C. Chou, H. A. Tsai, W. S. Hung, C. C. Hu, K. R. Lee and J. Y. Lai, Carbon, 2019, 143, 343–351 CrossRef CAS
. - N. Álvarez-Gutiérrez, M. v Gil, F. Rubiera and C. Pevida, Fuel Process. Technol., 2016, 142, 361–369 CrossRef
. - S. Sethupathi, M. J. Bashir, Z. A. Akbar and A. R. Mohamed, Waste Manage. Res., 2015, 33, 303–312 CrossRef CAS PubMed
. - H. Wei, J. Chen, N. Fu, H. Chen, H. Lin and S. Han, Electrochim. Acta, 2018, 266, 161–169 CrossRef CAS
. - Y. Zhao, X. Liu, K. X. Yao, L. Zhao and Y. Han, Chem. Mater., 2012, 24, 4725–4734 CrossRef CAS
. - W. N. R. W. Isahak, Z. A. C. Ramli, M. W. Ismail, K. Ismail, R. M. Yusop, M. W. M. Hisham and M. A. Yarmo, J. CO2 Util., 2013, 2, 8–15 CrossRef CAS
. - B. Petrovic, M. Gorbounov and S. M. Soltani, Microporous Mesoporous Mater., 2021, 312, 110751 CrossRef CAS
. - D. Saha and M. J. Kienbaum, Microporous Mesoporous Mater., 2019, 287, 29–55 CrossRef CAS
. - J. A. Schott, Z. Wu, S. Dai, M. Li, K. Huang and J. A. Schott, Microporous Mesoporous Mater., 2017, 249, 34–41 CrossRef
. - S. Shahkarami, A. K. Dalai and J. Soltan, Ind. Eng. Chem. Res., 2016, 55, 5955–5964 CrossRef CAS
. - F. Hussin, M. K. Aroua and R. Yusoff, J. Environ. Chem. Eng., 2021, 9(4), 105333 CrossRef CAS
. - I. Prasetyo, N. I. F. Mukti, M. Fahrurrozi and T. Ariyanto, ASEAN J. Chem. Eng., 2018, 18, 09–16 CrossRef
. - M. Jain, M. Yadav, T. Kohout, M. Lahtinen, V. K. Garg and M. Sillanpää, Water Resour. Ind., 2018, 20, 54–74 CrossRef
. - B. Buhani, S. Suharso, F. Luziana, M. Rilyanti and S. Sumadi, Desalin. Water Treat., 2019, 171, 281–293 CrossRef
. - W. Yang, Z. Du, Z. Ma, G. Wang, H. Bai and G. Shao, RSC Adv., 2016, 6, 3942–3950 RSC
. - B. Lesiak, N. Rangam, P. Jiricek, I. Gordeev, J. Tóth, L. Kövér, M. Mohai and P. Borowicz, Front. Chem., 2019, 7, 1–16 CrossRef PubMed
. - F. Larasati, Y. Kusumastuti, A. Mindaryani, R. Rochmadi and M. Handayani, ASEAN J. Chem. Eng., 2022, 22, 82–92 CrossRef CAS
. - M. M. Zainol, M. N. F. Roslan, M. Asmadi and N. A. S. Amin, ASEAN J. Chem. Eng., 2021, 21, 1–10 CrossRef CAS
. - A. Ayub, Z. A. Raza, M. I. Majeed, M. R. Tariq and A. Irfan, Int. J. Biol. Macromol., 2020, 163, 603–617 CrossRef CAS PubMed
. - S. H. Khalil, M. K. Aroua and W. M. A. W. Daud, Chem. Eng. J., 2012, 183, 15–20 CrossRef CAS
. - B. G. H. Briton, L. Duclaux, Y. Richardson, K. B. Yao, L. Reinert and Y. Soneda, Appl. Water Sci., 2019, 9, 1–14 CrossRef CAS
. - W. Liang, Z. Liu, J. Peng, X. Zhou, X. Wang and Z. Li, Energy Fuels, 2019, 33, 493–502 CrossRef CAS
. - D. Saha, S. E. van Bramer, G. Orkoulas, H. C. Ho, J. Chen and D. K. Henley, Carbon, 2017, 121, 257–266 CrossRef CAS
. - Ã. Kirbiyikkurukavak, B. Z. Buÿükbekar and M. Ersöz, Turk. J. Chem., 2021, 45, 914–926 CrossRef
. - M. Jonnalagadda, R. Anjum, H. Burri and S. Mutyala, J. Chem. Res., 2021, 45, 194–200 CrossRef CAS
. - Y. Xia, Y. Zhu and Y. Tang, Carbon, 2012, 50, 5543–5553 CrossRef CAS
.
|
This journal is © The Royal Society of Chemistry 2023 |