DOI:
10.1039/D3RA02018E
(Review Article)
RSC Adv., 2023,
13, 14914-14929
A review on arsenic in the environment: bio-accumulation, remediation, and disposal
Received
28th March 2023
, Accepted 1st May 2023
First published on 16th May 2023
Abstract
Arsenic is a widespread serious environmental pollutant as a food chain contaminant and non-threshold carcinogen. Arsenic transfer through the crops-soil-water system and animals is one of the most important pathways of human exposure and a measure of phytoremediation. Exposure occurs primarily from the consumption of contaminated water and foods. Various chemical technologies are utilized for As removal from contaminated water and soil, but they are very costly and difficult for large-scale cleaning of water and soil. In contrast, phytoremediation utilizes green plants to remove As from a contaminated environment. A large number of terrestrial and aquatic weed flora have been identified so far for their hyper metal removal capacity. In the panorama presented herein, the latest state of the art on methods of bioaccumulation, transfer mechanism of As through plants and animals, and remediation that encompass the use of physicochemical and biological processes, i.e., microbes, mosses, lichens, ferns, algae, and macrophytes have been assessed. Since these bioremediation approaches for the clean-up of this contaminant are still at the initial experimental stages, some have not been recognized at full scale. Nonetheless, extensive research on these primitive plants as bio-accumulators can be instrumental in controlling arsenic exposure and rehabilitation and may result in major progress to solve the problem on a worldwide scale.
Introduction
Arsenic (As) is a major source of environmental threat due to its high toxicity, contamination, many different pollution sources, non-biodegradability, and high concentrations in the environment and accumulation; it is also an element that is very costly and difficult to recover and dispose of from its environment.1 Arsenic is present in the environment in various inorganic and organic chemical forms: arsenite (As III), arsenate (As V), monomethylarsonic acid (MMA), dimethylarsinic acid (DMA), trimethyl arsine oxide (TMAO), and arsenobetaine (AsB), etc.2 The toxicity, mobility, and solubility differ among species in such a way that inorganic As(III) is more toxic than As(V), and in turn, organic As species are less toxic.3 The polluting and toxicity levels of the inorganic form of arsenic within these species are a concern because the metalloid does not degrade and cannot be destroyed in the environment. Today, it is reported that the arsenic concentration in drinking water in more than 42 countries of the world exceeds the limit value (10 μg L−1) for drinking water determined by the World Health Organization and included in the standard guidelines.4,5
Arsenic is widely distributed in air, water, soil, and plants. Air is a potential source of As exposure in industrial areas mainly due to the emission of airborne particulate matter from the smelting of ores and coal combustion. Related to this subject, the potential pathways of arsenic exposure and their reduction were reviewed before by Chung et al. 2014 and Raju 2022.6,7 Also, the arsenic chemistry and factors controlling the sorption/desorption, mobility, uptake of As by plants and reduction of translocation in plant tissues and release of As from sediments into groundwater have been reviewed by Aftabtalab et al. 2022, Dadwal and Mishra 2017, and Pigna et al. 2015.8–10
Exposure of arsenic to mammals, aquatic biota, and wildlife is of major concern to human health.11,12 Arsenic contamination of the environment (i.e., water soil, sediment, and biomass) is a great concern for widespread problems due to increased awareness of the health risks. Various methods, i.e., immobilization, flash pyrolysis, adsorption-pyrolysis, etc. for the treatment of As-contaminated samples have been reported.13
For this reason, it is important to investigate and disinfect arsenic pollution in all kinds of environments. Conventional techniques, including oxidation, coagulation–flocculation, and membrane techniques are frequently used for the removal of arsenic from water.14 Several methods, i.e., chemical, physical, and biological including membrane and nanoparticles for the removal of As from water and soil were reviewed.14–17 However, some of the options are financially infeasible in developing countries, where natural adsorbents (in particular, waste materials/by-products and microalgal and fungal biomass) can offer sustainable and cost-effective remediation solutions.18 Arsenic biotransformation in the environment occurs via three major modes: redox transformation between As(III) and As(V), reduction and methylation of As, and bioproduction of organic arsenic compounds.19–22
The aim of the review is to provide up-to-date information on arsenic contamination of plants and animals, transfer mechanism, bioaccumulation measurement, remediation technologies, and disposal of contaminated materials. The important scientific knowledge gaps and critical areas are figured out for future research.
Results and discussion
Biota accumulation
Accumulation potential, mechanism, and biotransformation of As in biota, i.e., terrestrial and aquatic plants and animals, and primitive plants, are presented as follows.
Terrestrial plant. Arsenic is a non-essential element accumulated in plants via oxidative and/or genotoxic mechanisms depending upon several factors. It can enter plants through soil, water, and air, entering the food chain, where accumulation and biomagnification occur.23 In fact, plants – particularly rice, wheat, maize, and vegetables – are the main source of exposure for the general population.24 Moreover, as a non-essential element, arsenic also causes adverse effects in crops, such as interference with various metabolic pathways by inactivation of enzymes or competitive inhibitors of phosphate (Pi), a burst of reactive oxygen species (ROS), photosynthetic efficiency, damage of lipid, protein, carbohydrate and nucleic acids, decrease in biomass and yield productivity, and the appearance of toxicity symptoms,25,26 Typical toxicity of As in plants is illustrated in Fig. 1. The oxidative stress is mitigated either by higher activities of the enzymes or exogenous uses of proline.27–29 This toxicity is responded to by plants via several mechanisms: hyperaccumulation, antioxidant defense system, and phyto-chelation.30,31 Arsenate reduction to As(III) and its subsequent sequestration into the vacuole in plant roots is considered a major As detoxification mechanism.32–34 In addition to As reductase enzymes, the enzymes from other systems also could reduce As(V), e.g. glyceraldehyde-3-phosphate dehydrogenase, and F1Fo ATP-synthase in the mitochondria. Moreover, instead of inorganic phosphate, these enzymes incorporate As(V) into the biological molecules and form arseno-esters.35–37
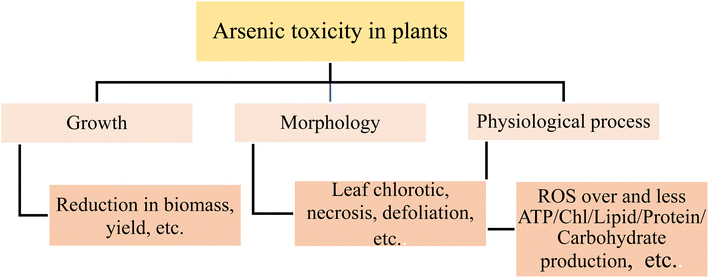 |
| Fig. 1 Arsenic toxicity in plants. | |
Regarding root uptake from the soil, various factors control the dynamics of As in the rhizosphere, such as the formation of iron plaque, phosphate status, soil oxidation/reduction status, and inter-conversion between organic and inorganic As species.35 Furthermore, the soil parent material and clay contents/type plays a vital role in the regulation of As in soil solution. The uptake ability of As by plants varies from species to species.
The speciation pattern of As also affects its accumulation by plants. Arsenate is a chemical analog to inorganic phosphate and is efficiently taken up by plant roots through inorganic phosphate transport. Arsenate is uploaded to the xylem vessels through an inorganic phosphate transport protein, but As non-hyperaccumulators generally retain the majority of As(V) in the plant's root. On the other hand, in rice plants, nodulin-26 like plasma membrane proteins (LSi1 and LSi2) are also responsible for As(III) uptake and transport.38
Cereal grasses, bamboos, and natural grasses that belong to the Poaceae family are some of the main sources of food for both humans and animals.39 For instance, rice (Oryza sativa), an aquatic grass, is considered the main food for ≈50% of the population of the world,40 and rice husk (seed coat) and straw (vegetative part) are used as fodder for livestock. Nonetheless, grasses can be heavily polluted with arsenic: the shoots and roots of red fescue (Festuca rubra), barnyard, and bermudagrass (Cynodon dactylon) were contained As up to 824 mg kg−1.41–44 In the particular case of rice, it accumulated more As than other crops due to its uptake from both contaminated water and soil.45 Moreover, studies on arsenic speciation in rice have shown that the major fraction corresponded to inorganic As.46 Examples of arsenic contamination in different types of plants (grasses, herbs, shrubs, and trees)47–62 are presented in Table 1.
Table 1 Arsenic reported in different plants
Location |
Samplea |
As concentrationb (mg kg−1) |
Source |
Ref. |
The number of samples is given in brackets. The number in brackets following a concentration range is the mean value, while the number after “±” is the standard deviation. The samples are as following: Cynodon dactylon (grass)/Oryza sativa (rice)/Amaranthus spinosus L./Amaranthus tricolor L./Coriandrum sativum/Foeniculum vulgare/Capsicum annuam/Solanum melongena/Solanum lycopersicon/Dioscorea bulbifera/Amorphophallus paeoniifolius/Acacia concinna/Momordica charantia/Cucurbita máxima/Trigonella foenum-graecum/Vigna radiate/Cajanus cajan/Vigna sinensis/Vigna unguiculata/Lablab purpureus/Tamarindus indica/Vachellia nilotica/Musa paradisiaca/Zingiber officinale L./Curcuma longa/Ficus racemose L./Artocarpus heterophyllus/Ziziphus mauritiana/Ficus religiosa/Carica papaya/Moringa oleifera/Tectona grandis/Ocimum tenuiflorum/Mentha spicata/Hibiscus sabdariffa/Abelmoschus esculentus/Citrus limon/Aegle marmelos/Psidium guvava/Syzygium cumini/Madhuca longifolia/Schleichera trijuga/Diospyros melanoxylon/Mangifera indica/Shorea robusta. |
Kaudikasa, Rajnandgaon, India |
Rice(20)/husk(20)/straw(20)/root (20) |
0.17–0.72 (0.47 ± 0.07)/0.40–1.58(0.83 ± 0.15)/2.5–5.9(4.2 ± 0.5)/204–354 (276 ± 21) |
Atmospheric deposition and soil |
47 |
Bangladesh |
Grain/husk/traw |
(0.5 ± 0.02)/(1.6 ± 0.20)/(2.37 ± 0.44) |
Irrigation water |
48 |
Bangladesh |
Grain/husk/straw(17) |
0.08–0.45(0.23 ± 0.08)/0.14–1.87(0.75 ± 0.38)/0.46–9.50 (2.89 ± 2.01) |
Soil and irrigation water |
49 |
Bangladesh |
Grain/husk/straw/root(20) |
0.19–1.17/0.45–3.42/0.64–16.3/18 – 478 |
Irrigation water |
50 |
Republic of Kazakhstan |
Rice (95) |
0.25–0.45(0.36 ± 0.02) |
Soil |
51 |
Bangkok, Thailand |
Rice (31) |
0.084–0.27 (0.17 ± 0.009) |
Environmental contamination |
52 |
Malaysia |
Rice (22) |
(0.091 ± 0.001) |
Environmental contamination |
53 |
Brazil |
Rice (Oryza sativa L.)(27) |
0.003–1.3 |
Pesticides |
54 |
Raipur, India |
Weeds(3) |
1.0–3.5 |
Atmospheric deposition |
55 |
Ambagrh Tehsil, Rajnandgaon, India |
Plant leaves(45)c |
6.2/5.6/12/3.1/8.2/7.1/3.9/4.6/9.3/5.7/3.9/7.1/13/1.7/3.7/2.2/5.9/0.8/27/1.3/0.7/3.2/9.1/1.9/2.2/3.3/4.1/6.2/9.5/1.4/6.4/5.2/7.1/7.4/1.8/0.3/8.1/3.8/3.3/4.7/0.8/6.2/17/4.6 |
Atmospheric deposition and soil |
56 |
West Bengal, India |
Pea/lentil/mustard seed/potato/cauliflower/onion bulb/brinjal/spinach/bitter guard/garlic/radish/green chili/arum/amarnath/papaya/cabbage/lady's finger/pumpkin/beans/tomato/lemon |
0.0003–1.02/0.141/0.096/0.198/0.654/0.293/0.162/0.279/0.257/0.021/0.126/0.344/0.085/0.407/0.372/0.258/0.209/0.301/0.184/0.090/0.084/0.012/0.209/0.301/0.184/0.090/0.084/0.012 |
Soil and water |
57 |
Korba, India |
Leafy vegetables(6) |
0.56–2.05 (1.55 ± 0.19) |
Atmospheric deposition and soil |
58 |
Korba, India |
Tree leaves(6) |
2.8–43.1 (10.38) |
Atmospheric deposition and soil |
59 |
West Bengal, India |
Coriander leaf/stem/root (5) |
0.54–0.80 (0.69)/0.44–61 (12.60)/0.99–2.01 (1.45) |
Groundwater |
60 |
West Bengal, India |
Cumin/turmeric powder/arum leaf/papaya |
0.04786–0.20975/0.29733–0.2809/0.331–0.341/0.1965–0.373 |
Irrigation water |
61 |
West Bengal, India |
Potato/onion/cauliflower/carrot/tomato |
0.24–0.30/0.10–0.29/0.19–0.20/0.24–0.29/0.15–0.18 |
Contaminated water |
62 |
Bangladesh |
Vegetables/crops(7) |
0.96–1.43 (1.7 ± 0.16) |
Industrial effluents |
63 |
Nepal |
Onion leaves/onion bulb/cauli flower/rice/brinjal/potato (6) |
<0.01–0.55 (0.27) |
Irrigation water |
64 |
Nigeria |
Guava/apple/pineapple/orange/pawpaw (5) |
20–96.84(53.79) |
Environmental contamination |
65 |
Serbia |
Black pepper powder(2)/ginger powder(2) |
0.33–0.51(0.42 ± 0.02)/0.11–0.18(0.145 ± 0.008) |
Environmental contamination |
66 |
South Africa |
C. Albumy, B. oleracea, S. nigrum, B. rapa(4) |
1.5–1.9 (1.7 ± 0.2) |
Industrial and commercial activities |
67 |
Thailand |
Zingiberaceous rhizomes(6) |
0.0425–0.1451 |
Soil |
68 |
Global |
Bertel leaves/slaked lime/zarda (flavored tobacco) betel quids(4) |
0.406/4.56/0.285/0.035 |
Environmental contamination |
69 |
Global |
Tobaco(8) |
0.144–3.9149 (1.4586) |
Environmental contamination |
70 |
Hainan Island, China |
Mango |
0.0006–0.05 (0.0086) |
Soil |
71 |
Bangladesh |
Coconut water(28) |
0.005–0.170 (0.070) |
Soil pollution |
72 |
The Codex Alimentarius Commission73 established a maximum limit of 0.2 mg kg−1 for inorganic As. However, overall, As levels in rice have been shown to vary widely from 0.020–2.05 mg kg−1 among various countries in Asia, Europe, and the USA. The highest level was reported in South Bangladesh. The As uptakes in rice depend on its concentration in water and soil, physicochemical factors of soil i.e. Fe, P, S, and Si concentrations, and environmental conditions controlling As availability and uptake in the soil–rhizosphere–plant system.74 As contents up to 1.09 mg kg−1 have been detected in paddy rice and rice products: rice milk, rice bran, baby rice, rice crackers, brown rice syrup, cereal bars, and breakfast cereals. The high-yield rice varieties, such as Kalinga, IR-64, G. Gurmatia, Shyamla, Ek Hazar Das, and M2, were found to be more sensitive to As accumulation.47,51–54 Arsenic concentration increases in the sequence: rice grain < husk < straw ≪ root.48–50 Similar arsenic accumulation patterns have been observed in wheat, pea, and bean grown in soil contained with As.75,76 Bamboo, an important plant in the tropical region, was found to accumulate As over the 0.03–0.09 mg kg−1 range.77,78 The fractions of MMA, DMA, and TMAO were in the 4.2–16.5, 13.9–44.9, and 11.8–18.4% intervals, respectively.
About arsenic concentration in fruits, vegetables, and plant leaves, the values ranging from 0.01 to 0.10, 1.06–1.43, and 2.8–43.1 mg kg−1, respectively, have been reported.59,63,65,71 Commonly consumed leafy and tuberous vegetables, as well as spices and coconut water, have also been identified as potential As intake sources.57,58,60–62,66–68,72 Tobacco leaves and weeds are also potential sources for As (0.538–3.5 mg kg−1) intake.55,69
Aquatic life. Arsenic is widespread in aquatic environments due to its natural and anthropogenic activities. It is a hazardous pollutant and its toxic effects on aquatic life are significantly dependent on various abiotic factors (e.g., temperature, pH, Eh, organic matter content, phosphate concentration, suspended solids, and presence of other substances and toxicants), as well as on As speciation, and duration of exposure. Continuous exposure of freshwater organisms, including fish, to low concentrations of As (10 μg L−1) results in bioaccumulation in the liver and kidney, inducing hyperglycemia, depletion of enzymatic activities, various acute and chronic toxicity, and immune system dysfunction.79Arsenic is found in estuarine and marine waters at concentrations in the 0.5–3 μg L−1 range with a mean value of 1.3 μg L−1.80 Marine organisms – including in macroalgae and invertebrates and fish – normally contain high organic As residues, such as arsenosugars and AsB.81,82 The inorganic As concentration is negligible, and methylated As compounds are identified in marine ecosystems from the enzymatic methylation of inorganic As.
Total and organic arsenic concentrations in representative marine organisms are presented in Table 2. It may be observed that arsenosugars are accumulated in marine algae and crustaceans at high concentrations of up to 100 mg kg−1.83–85 AsB, an amino acid derivative, is predominant in most finfish and shellfish, zooplankton, and some algae, while arsenolipids are present in seaweeds.
Table 2 Arsenic content in marine organisms
Location |
Sample |
Concentrationa (mg kg−1) |
Ref. |
The concentration is given for AsT, with additional information for specific species in the brackets. |
USA |
Seafood |
20–100 (arsenosugar, 80%; organolipids, arsenic hydrocarbons and arsenic glycophospholipids, 0.6–6.7%) |
82 |
Greek coastal areas |
Shrimp |
11.8–62.6 (AB, 8.6–58.8) |
83 |
Poland |
Hijiki algal species |
102.7 |
84 |
Gulf of Trieste, Italy |
Benthic (Pteromylaeus bovinus, Myliobatis aquila) and pelagic rays (Pteroplatytrygon violacea) |
32.4–362 |
85 |
Canada |
Phytoplankton |
154–894 (iAs, 38–98%) |
86 |
Canada |
Zooplankton |
7–340 (sulfate arseno-sugar, 47%) |
86 |
NY, USA |
Shrimp (Acetes sp.) |
5–30 (AB, <95%) |
87 |
Arabian gulf |
Shellfish and finfish |
11–134 (iAs, 0.03%; AB, 81%) |
88 |
In Canadian lake water, the zooplankton and phytoplankton organisms contained As concentrations of up to 340 and 894 mg kg−1 dry weight (DW), respectively. The main As compounds identified by HPLC-ICP-MS in all plankton were inorganic As (from 38% to 98% of total arsenic). No other As compounds were found in phytoplankton, but zooplankton organisms showed the presence of organoarsenic compounds accounting for up to 47% of the total As.86 Total As observed in various fishes of New York and the Arabian Gulf ranged from 5 to 134 mg kg−1.87,88
Terrestrial animal. Terrestrial animals, i.e., cows, buffalos, goats, sheeps, pigs, birds, rabbits, etc. are exposed to As generally via water and feed. They are used as food and frequently contaminated with As89 as shown in Table 3. This contamination also affects products of animal origin (POAO): for instance, poultry egg yolk, albumen, and poultry products retain arsenic, reaching concentrations of up to 0.465 mg kg−1, respectively.90,91 The levels of As in the liver, kidney, and meat of beef, mutton, caprine, and chicken varied from 0.01 to 0.34 ± 0.23 mg kg−1, and livers and kidneys were found to have the highest significant levels.92 The mean concentration of As in the meat was observed in the order: chicken > duck > beef > mutton.93 The highest concentration of As was observed in chicken meat (2.9 ± 3.6 mg kg−1) and the lowest was found in mutton (1.3 ± 1.2 mg kg−1). Quite high concentrations of arsenic (40.80–52.44 mg kg−1) in the meat samples from Pakistan were reported.94
Table 3 Arsenic contamination in animal samples
Location |
Samplea |
Concentrationb (mg kg−1) |
Source |
Ref. |
If available, the number of samples is given in brackets. The number in brackets is the mean value, while the number after “±” is the standard deviation. Angle brackets following a concentration range indicate the details for different sample types. Results are given based on wet weight. |
West Bengal, India |
Chicken egg albumen (22)/chicken egg yolk (22) |
0.028–0.062/0.048–0.111 |
Water and feed |
90 |
West Bengal, India |
Chicken and duck feathers (30)/chicken and duck litters (30)/chicken whole egg (30)/duck whole egg (30) |
(0.385 ± 0.125)/(0.535 ± 0.101)/(0.222 ± 0.120)/(0.155 ± 0.231) |
Ground water |
91 |
Nigeria |
Beef liver/mutton liver/caprine liver/chicken liver/beef kidney/mutton kidney/aprine kidney/chicken kidney |
(0.08 ± 0.01)/(0.34 ± 0.23)(0.15 ± 0.06)/(0.03 ± 0.01)/(0.14 ± 0.01)/(0.18 ± 0.06)/(0.05 ± 0.22)/(0.11 ± 0.01) |
Environment |
92 |
Bangladesh |
Chicken/duck/beef/mutton (100) |
0.08–3.3<(2.9 ± 3.6)/(2.4 ± 1.0)/(1.4 ± 0.90)/(1.3 ± 1.2)> |
Environment |
93 |
Pakistan |
Mutton/chicken |
42.4/44.09 |
Environment |
94 |
Canada |
Frog leg |
1.6–4.4 (2.7 ± 1.1) |
Food and environment |
95 |
USAc |
Pine snake: liver(17)/kidney(15)/muscle(20)/skin(20)/heart(15)/blood(29) |
(0.156 ± 0.0273)/(0.167 ± 0.0532)/(0.125 ± 0.0313)/(0.0914 ± 0.0217)/(0.145 ± 0.0473)/(0.0070 ± 0.0033) |
Food |
96 |
USAc |
Water snake from East Fork Poplar Creek: blood(20)/kidney(20)/liver(20)/muscle(20)/skin(20)/whole body(20) |
0.0028–0.027(0.0101 ± 0.0013)/0.0068–0.167(0.0513 ± 0.0103)/0.000424–1.151(0.171 ± 0.058)/0.0072–0.112(0.0379 ± 0.0084)/0.014–0.084(0.0369 ± 0.0044)/(0.129 ± 0.021) |
Food |
97 |
Water snake from Little River: blood(27)/kidney(27)/liver(27)/muscle(27)/skin(27)/whole body(27) |
0.0011–0.076(0.0184 ± 0.0030)/0.001–0.400(0.0669 ± 0.0166)/0.00098–0.178(0.0369 ± 0.0071)/0.0015–0.190(0.0328 ± 0.0087)/0.00015–0.140(0.0323 ± 0.0069)/(0.071 ± 0.008) |
Japan |
Green turtles: intestine(2)/kidney(6)/liver(5)/lung(2)/muscle(5)/spleen(2)/tomach(1) |
6.6/(17 ± 14)/(4.9 ± 3.3)/7.9/(69 ± 52)/7.6/6.148/28/(45 ± 38)/(25 ± 32)/33/(210 ± 140)/24/22 |
Food |
98 |
Hawksbill turtles: eyeball(1)/heart(1)/kidney(6)/liver(10)/lung(1)/muscle(9)/spleen(1)/stomach(1) |
The amphibians are exposed to As via soil, food, and water as inorganic arsenic, and they bio-transformed in the less toxic organo arsenicals, i.e., MMA, DMA, and TMAO. The total As concentration in frog legs observed was 1.6–4.4 mg kg−1 with a mean value of 2.7 ± 1.1 mg kg−1.95 The methylated organo arsenicals contributed major fractions: MMA (<6%), DMA(V) (up to 46%), and TMAO (<10%). High As of up to 0.171 mg kg−1 (wet weight) in snake liver was observed.96,97 In the muscle of Green turtles and Hawksbill turtles, high total As of up to 69 mg kg−1and 210 mg kg−1, respectively, were reported but present mostly in the organic form 66–84% as AsB.98
The ingestion of milk has been reported to be one of the most important pathways of exposure to As in humans.99 Rapid increase in urbanization and industrialization caused contamination of milk with toxic elements.100,101 For the case of arsenic, taking milk samples from cows, buffalos, sheep, goats, and camels as an example is shown in Table 4. It is worth noting that a positive correlation (0.926–0.974) between the As concentration in milk samples of cattle and that in the corresponding drinking water of farms/flocks has been confirmed in the literature.102 It should also be clarified that organic species, such as AsB and dimethylarsinate constitute most of the water-soluble arsenic present in milk.103 The As content in the human and animal milk samples ranged from 2.36 to 10.75 and from 4.93 to 72.0 μg L−1, respectively.90,103–106 At least 5-fold more As in the animal milk was detected due to feeding of As contaminated foods and water.
Table 4 Arsenic contamination in milk
Location |
Milk typea |
Concentrationb (μg L−1) |
Source |
Ref. |
The number of samples is given in brackets. The number in brackets is the mean value, while the number after “±” is the standard deviation. |
West Bengal |
Cattle (30) |
(13 ± 2)–(72 ± 12) |
Water and food |
90 |
Iran |
Human (20) |
(7.73 ± 4.01)–(10.75 ± 7.62) |
Water and food |
101 |
Pakistan |
Cow/buffalo/sheep/goat/camel (3) |
15.1–18.4/2.6–7.7/25.7–33.2/10.5–37.3/6.6–13.7 |
Water and food |
103 |
Lebanon |
Human milk (74) |
(2.36 ± 1.95) |
Food and smoking |
104 |
Turkey |
Human/cow (35) |
(4.219 ± 0.079)/(4.932 ± 0.38) |
Environment |
105 |
Bangladesh |
Cow milk (240) |
(26.2 ± 2.8) |
Water/paddy/straw |
106 |
Mosses and lichens. Mosses and lichens are non-vascular plants that clean polluted air and water by absorbing contaminants. The accumulation of As is carried out by the ion exchange process by the formation of complexes between the metal ions and the organic functional groups, i.e., phosphodiester, carboxyl, phosphoryl, polyphenols, sulfhydryl, and amine groups of surface cells. The adsorbed metal on the moss surface is uptaken by specific membrane transport proteins or via channels present in the cell membrane.107 Arsenical Compound Resistance (ACR3) gene is found in a wide range of organisms including bacteria, fungi, mosses, and gymnosperms, and extruded As from the cell cytoplasm thus lowering the intracellular concentration.108Mosses such as Fabriona ciliaris, Leskea angustata, Pleurozium schreberi, Scleropodium purum, Hylocomium splendens, lagiothecium denticulatum, Bryum argenteum, Sphagnum sp., Hypnum cupressiforme, Thuidium tamariscinum, Brachytechium salebrosum, Brachytechium rutabulum, Polytrichum formosum, and Sphagnum girgensohnii have been used for mapping metal pollution from localized atmospheric sources due to the accumulation by passive transport.109 Moreover, mosses can clean polluted water by absorbing contaminants. Compounds such as glutathione, phytochelatins, metallothionins, and secondary metabolites play an important role in As detoxification and their enhanced tolerance.110
Representative reports on the accumulation of As in mosses, lichens, ferns, algae, and macrophytes are summarized in Table 5.111–141
Table 5 Accumulation of As with mosses, fern, algae and macrophyte
Location |
Samplea |
Concentrationb (mg kg−1) |
Source |
Ref. |
If available, the number of samples is given in brackets. The number in brackets is the mean value, while the number after “±” is the standard deviation. |
South China |
Mosses |
0.67–9.6 (2.4 ± 1.7) |
Industrial emission |
111 |
Japan |
Scopelophila cataractae |
1300 |
Mine tailing |
112 |
Dhar, MP, India |
Foliose lichens/leprose lichens/squamulose/crustose |
10.98–51.95/28.63–51.20/(0.46 ± 0.03)/(20.99 ± 0.58) |
Industrial activities |
113 |
Katni, MP, India |
Pyxine cocoes (7) |
0–33.4 (11.58) |
Mining and industrial activities |
114 |
Rewa, MP, India |
Phaeophyscia hispidula (8) |
0–19.6 (8.53) |
Vehicular emission |
|
West Bengal, India |
Foliose lichen Pyxine cocoes (Sw.) Nyl |
(48.1 ± 2.1) |
Contaminated environment |
115 |
Austria |
Vaccinium vitis idaea and Equisetum pretense (2) |
0.27–8.45 |
Arsenic smelter |
116 |
England |
Pteris cretica chilsii/Pteris cretica crista/Pteris cretica mayii/Pteris cretica parkerii/Pteris cretica rowerii/Pteris longifolia (6) |
1358/1506/1239/2493/1425/2361 |
Simulation experiment |
117 |
South China |
Pteris cretica (fronds)/Pteris cretica (brake) |
3–704/149–694 |
Arsenic mine |
118 |
South China |
P. vittata (78)/P. cretica (13)/P. multífida (3) |
4.54–3599/28.7–757/11.2–341 |
Industrial emisión |
119 |
Yunnan, Southwestern China |
Pteris vittata L. fronds |
7215–11 110 |
Contaminated soil |
120 |
South China |
P. multifida and P. oshimensis |
1262–47 235 |
Arsenic mine |
121 |
South China |
A. capillus-veneris |
Up to 500 |
Simulation experiment |
122 |
South China |
Pteris fauriei |
337 |
Industrial emissions |
123 |
South China |
Pteris roots(5)/Pteris fronds(5)/Adiantum roots(5)/Adiantum frond(5)/Marsilea roots(5)/Marsilea frond(5)/Ceratopteris roots(5)/Ceratopteris frond(5) |
1.492–125.316/0.37–43.63/0.477–31.157/0.413–26.965/1.214–104.812/0.531–45.85/0.2578–37.3885/0.076–11.08 |
Contaminated soil |
124 |
South China |
Pteris vittata (brake fern) |
3280–4980 |
Contaminated soil |
125 |
Raipur, India |
Chara spp./Lyngbya spp./Nitella spp./Pithophora spp./Hydrodictyon spp./Spirogyra spp. |
8/10/20/10/9/9 |
River water |
126 |
Loa River Basin, Chile |
Cladophora sp. and Chara sp. and aquatic plants: Azolla sp., Myriophyllum aquaticum, Phylloscirpus cf. desserticola, Potamogeton pectinatus, Ruppia filifolia and Zannichellia palustris (5) |
182–11100/20–48 |
Contaminated water |
127 |
China |
Sea weed (Rhodophyta)/Phaeophyta/Chlorophyta (92) |
>100/20–50/10–15 |
Sea water |
128 |
Spain |
Algae (18) food product |
AsT: 2.3–141, iAs: 0.15–88 |
Industrial activities |
129 |
Bangladesh |
Green algae (Pithophora) |
1400.4 |
Simulation experiment |
130 |
Japan |
Chlorella vulgaris |
50 000 |
Simulation experiment |
131 |
China |
Hydrilla verticillata (L.f.) Royle (shoot) |
>700 |
Simulation experiment |
132 |
China |
Ceratophyllum demersum L. (shoot) |
862–963 |
Simulation experiment |
133 |
Iraq |
Wolffia globose, Ceratophyllum demersum and Ceratophyllum demersum |
1000/284/963 |
Simulation experiment |
134 |
China |
T. angustifolia |
150 |
Simulation experiment |
135 |
Japan |
Ceratophyllum demersum L. |
227.5 |
Simulation experiment |
136 |
Kinghorn Loch, UK |
Persicaria amphibia (L.) Gray (leaves, stems and roots) |
(40 ± 65.3) |
Red mud sludge |
137 |
Thailand |
Limnocharis flava (plant) |
(0.78 ± 0.31) |
Municipal landfill |
138 |
Raipur, India |
Azolla pinnata, Pistia stratiotes, Salvinia molesta, Nelumbo nucifera, Trapa natans, Persicaria punctate, and Persicaria maculosa |
1.0–8.0 |
River water |
139 |
Bolivia |
Totora plant (root)/Totora plant shoot and Periphyton |
30.8–65.0/0.4–1.5/1452–2647 |
Contaminated lake water |
140 |
Portugal |
Callitriche lusitanica/Callitriche brutia/L. minor/A. caroliniana/R. trichophyllus/Callitriche stagnalis/Fontinalis antipyretica |
2346/523/430/397/354/354/346 |
Contaminated water |
141 |
As is shown in Table 5, Epilithic and Scopelophila cataractae mosses were able to accumulate As in concentrations of up to 1300 mg kg−1.111,112 The dominant As species in the mosses were inorganic forms (87%), with low concentrations of organic forms, viz. MMA (11.8%) and DMA (6.0%).112
Lichens are also widely used as environmental indicators or bio-indicators. For instance, Asplenium nidus, Polypodium aureum, Polystichum tsus-simense, Pteris cretica, Pteris longifolia, and Pteris umbrosa have been assayed for biomonitoring of heavy metal air pollution142,143 Their secondary metabolites (e.g., usnic acid, norstictic acid, and psoromic acid) play an important role in binding the metal ions.144 As shown in Table 5, accumulation of As in the range of 0.46–51.95 mg kg−1 have been reported with different lichens (Equisetum pratense, Pyxine cocoes, Pyxine cocoes, Phaeophyscia hispidula, as well as foliose, leprose, squamulose and crustose lichens).113–116 Inorganic forms of As (arsenite and arsenate) were present in significant amounts in most of the lichen samples.145
Ferns. Ferns (oldest groups of plants) phyto-remediate both As(III) and As(V) via roots and store it in cellular vacuoles of fronds as arsenite. Transporter proteins (ATPases) mediate the metal uptake across the plasma membrane in the fern.146 Accumulation of As takes place in the cytosol via the phosphate cycle and is stored mostly as As(III) complex with thiol group compounds. The stress developed is detoxified by the use of glutathione, superoxide dismutase, and catalase for the scavenging various reactive oxygen species.The accumulation potentiality of ferns such as Pteris vittata, Pityrogramma calomelanos, P. cretica, P. longifolia, P. umbrosa, P. argyraea, P. quadriaurita, P. ryiunkensis and P. biaurita, Adiantum radiata, Chielanthes sinuta, and Polystichum acrostichoides is summarized in Table 5. They are very promising hyperaccumulators for As extraction, given that concentrations of up to 11
110 mg kg−1 have been reported.112,117–120,123–125,147–149
Algae. Algae (photosynthetic organisms) play an important role in the cycling of arsenic. The accumulation of As in algae occurs via the biosorption process.150 Several phytoplankton and cyanobacteria have been observed to be resistant to high As exposure. Detoxification of As in microalgae is carried out through adsorption on the cell surface and intracellular metabolism of As, including a redox reaction, complexation with carboxyl, hydroxyl, sulfate, sulfhydryl (thiol), phosphate, amino, amide, imine compounds and sequestration into vacuoles, methylation and further transformation to less toxic organic forms such as arsenosugars or arsenolipids, and excretion from cells.121 The most common forms of As in seaweeds are arsenosugars, some of which are considered to be innocuous: AsB, arsenocholine, and TMAO.151Some species of microalgae (Chlamydomonas spp., Chlorella vulgaris, Scenedesmus spp., Synechocysis spp., etc.) hold the potential for remediation of inorganic arsenic.121 Contents reported in the literature on algae food products; rhodophyta, phaeophyta, and chlorophyta; Chlorella vulgaris; Chara spp., Lyngbya spp., Nitella spp., Pithophora spp., Hydrodictyon spp., Spirogyra spp. and Cladophora spp. are presented in Table 5. Concentrations over a wide varying range have been registered, from 2.3 to 50
000 mg kg−1 DW.125–127,129,130 The inorganic As3+/As5+ forms were the major species, while glycerol-arsenosugar (gly-sug), DMA, and methylarsonic acid (MAA) were only present as minor constituents.127
Macrophytes. A number of aquatic plant species: macrophytes, such as Azolla spp., Callitriche hamulate, Callitriche stagnalis, Ceratophyllum demersum, Hydrilla verticillata, Limnocharis flava, Oenanthe crocata, Persicaria amphibia, Ranunculus peltatus, Ranunculus trichophyllus, Typha angustifolia, etc. have been studied for the remediation of toxic contaminants.152 Several metal chelators and enzymes are involved in the uptake, transportation, sequestration, and modification of arsenic for its storage into vacuoles.153The accumulation of As from contaminated environments in various macrophytes is summarized in Table 5. For instance, the rootless duckweed Wolffia globosa accumulated a relatively high concentration of As (>1000 mg kg−1 DW) in the frond.154 Arsenic concentrations of over the 104−2346 mg kg−1 have been reported for Callitriche spp., Ranunculus spp., Lemna minor, Oenanthe crocata, Azolla caroliniana and Fontinalis antipyretica.141,155 Simulation experiments for As accumulation conducted with Hydrilla verticillata, Ceratophyllum demersum, Azolla spp., and Typha angustifolia achieved contents of up to 5000 mg kg−1.130–136 Much lower values were found for other macrophytes, such as Limnocharis flava; Azolla pinnata, Pistia stratiotes, Salvinia molesta, Nelumbo nucifera, Trapa natans, Persicaria punctata, Persicaria maculosa, Persicaria amphibia, and totora plant, which bioaccumulated up to 87.2 mg kg−1 from the contaminated water.137–140
Remediation
Arsenic contamination of the environment is a widespread problem in many areas due to serious health risks. Conventional techniques, including oxidation, coagulation–flocculation, and membrane techniques, are frequently used for arsenic removal from water.14 In order to oxidize As(III) into As(V), oxidants such as O2, O3, Cl2, Cl2O, ClO, H2O2, ClNH2, KMnO4; photocatalytic oxidation (UV/H2O2); or chemoautotrophic arsenite-oxidizing bacteria (CAOs) may be used.156 The combination of FeCl3, Fe2(SO4)3, ZrCl4, ZrOCl2, TiCl3, TiCl4, Al2(SO4)3, etc. with an anionic flocculant is widely employed for coagulation of As in wastewater and sludge. Various membrane filtration techniques, i.e., microfiltration, ultrafiltration, nanofiltration, and reverse osmosis, are used for As(V) removal. Concerning arsenic-contaminated soil, remediation technologies include chemical methods (soil washing and immobilization), physical methods (soil replacement, soil cover, turnover and attenuation, and electro-kinetic remediation), biological methods (phytoextraction and bioremediation) and combined methods, which are discussed in detail in a recent review by Wan et al. 2019.157 In connection with As(V) sorption, many different materials have been assayed, including biochar, activated carbon, coal, red mud, fly ash, chicken feathers, kaolinite, montmorillonite, goethite, zeolites, activated alumina, titanium dioxide, iron hydroxide, zero-valent iron, chitosan, and cation-exchange resins.14,158 The maximum adsorption achieved with pine wood was up to 124 mg g−1.158 High removal efficiencies have also been reported for carbon nanotubes and nanoparticles of Fe2O3, Fe3O4, ZrO2, CeO2, hydrous titanium dioxide, Ti-BYC, etc.159–162 as well as for metal–organic frameworks (MOFs), which feature high porosity and surface areas.157 Adsorption capacities of up to 120 mg g−1(As V by FeO nanoparticle), 122.3 mg g−1 (As III by nano-structured Fe–Cu binary oxides), 296.23 mg g−1 (As III by nanoscale Fe–Mn binary oxides loaded on zeolite), and 201.10 mg g−1 (As V by nanoscale Fe–Mn binary oxides loaded on zeolite) have been reported, respectively.
The adsorption method is simple with high removal efficiency but others are inadequate due to interferences of other ions or high rejection water.163 The physical methods based on mixing polluted and nonpolluted soils, washing with various acids, and immobilization of As over solid matrices are tedious.164 The chemical methods in which As is adsorbed over metal oxides including nanosized particles (i.e., metal oxides, mixed metal oxides, hydroxide-based nanoparticles, magnetic, nanoparticles, composite nanoparticles, photocatalytic nanoparticles, etc.), and formation of insoluble stable phase viz. FeAsO4 and FeAsO4·2H2O are inadequate due to either lower efficiency or being expensive.165 Electro-remediation and membrane technologies are effective methods for arsenic remediation but to apply in the field is expensive and tedious.15,166
Bioremediation and phytoremediation approaches based on the reduction of As with bacteria (Pseudomonas, Psychrobacter, Citrobacter, Bacillus, Bosea, Vibrio, and Enterobacter), oxidation with (archaebacterium Sulfolobus acidocaldarius strain BC, Alcaligenes faecalis, Shewanella algae, β-proteobacteria strain UPLAs1, Alcaligenes faecalis, Comamonas terrae sp.) and methylation with (Escherichia coli, Salmonella, Vibrio, Yersinia, Haemophilus, and Brucella), and bioaccumulation of As with free-floating and aquatic rooted plants viz. water hyacinth, Agrostis sp., Pteris vittata, Pteris cretica, etc. are widely accepted methods.167,168 Some plants, e.g. Baccharis neglecta, Scirpus holoschoenus, Atriplex lentiformis, Rhododendron tomentosum, Lupinus albus, Retama sphaerocarpa, Ulex europaeus, Griselinia littoralis, Eucalyptus cladocalyx, Leptospermum scoparium, Fuchsia excorticate, Anthoxanthum odoratum, Agrostis capillaries, Veronica beccabunga, Salix atrocinerea, Viola allchariensis, Viola arsenica, and Viola macedonia belonging to families Asteraceae, Cyperaceae, Chenopodiaceae, Ericaceae, Fabaceae, Griseliniaceae, Myrtaceae, Onagraceae, Poaceae, Scrophulariaceae, Salicaceae and Violaceae were reported for phytostabilization of As in the roots.169 However, other plants e.g. Piracicaba, Braz Calotropis procera L., Leersia Oryzoides, Pteris (8223 ± 791 mg kg−1), and Eichhornia (4517 ± 402 mg kg−1) have been claimed for phytoextraction of As with translocation of maximum content in the upper part of plants.170–174 Most of them are hyperaccumulators as they accumulate As in shoots beyond a certain threshold limit, in which the BF (bioaccumulation factor, soil to plant metal transfer) and TF (translocation factor, root to shoot metal transfer) should be more than one (>1).
The phyto-remediation technology involves phyto-stabilization (elimination of bioavailability of metal in soil), rhizodegradation (degradation of contaminant under plant root sphere), rhizo-filtration (removal of metals from water), phytodegradation (breakdown of contaminant), and phytovolatilization (uptake of contaminant by plant roots and its conversion to a volatile form) mechanisms.175 The root plants exudate allelopathic compounds, terpenoids, flavonoids, amino acids, sugars, bicarbonates, organic and inorganic acids, protons, and sugars to stabilize, demobilize and bind the contaminants in the soil matrix.176 Various factors, i.e., plant-produced chelating agents, induced pH changes, and redox reactions, are responsible to solubilize and accumulate the metal. A range of transport mechanisms or specialized proteins embedded in the plant cell plasma membrane involved in metal uptake and translocation include sequestration inside root cells, transport via the symplast across the endodermis into the stele, and membrane transport-protein–mediated release into the xylem. Arsenic phytoremediation from soil or water can take place via various mechanisms, e.g., phytoextraction (removal of metals from soil matrix) phytostabilization, rhizofiltration (absorption of contaminants present in the rhizosphere into plant root system), and phytovolatilization, as shown in Fig. 2.
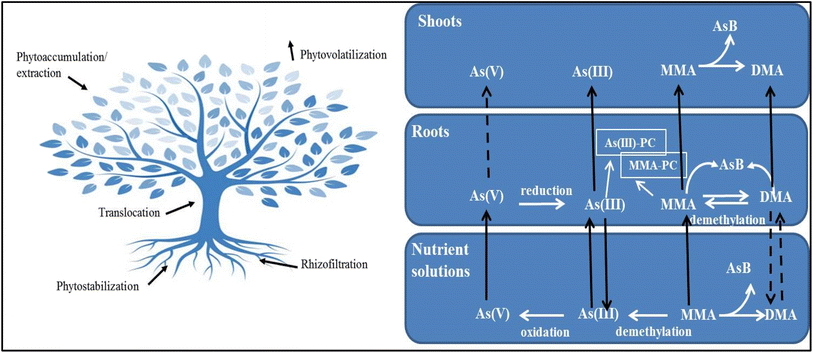 |
| Fig. 2 As transportation mechanisms. | |
Phytoremediation is a simple, convenient, and cost-effective technique to clean air, water, soil, and sediment, and discussed their potentiality with various plants, e.g., mosses and lichens, ferns, algae, and macrophytes in detail.
Removal efficiencies of As reported with representative techniques are summarized in Table 6.177–184 It can be seen from Table 6 that over 90% of As(V) can be effectively removed with adsorption, coagulation, ion exchange, and membrane methods (including NF and RO). By contrast, the removal efficiencies of As(III) are technique dependent and are apparently lower than those of As(V), which can be attributed to the possibility of As(III) existing as uncharged species (H3AsO30).177 Regarding that, inorganic As(III) is approximately 10-fold more toxic than As(V), improvement of removal efficiency of As(III) is required.
Table 6 Removal efficiencies of different arsenic removal techniques
Removal technology |
Initial concentration (mg kg−1) |
Removal efficiency for As(III) |
Removal efficiency for As(V) |
Reference |
Not available. |
Adsorption by porous adsorbent (iron oxide coated sands, green sands, activated carbon, nano-adsorbents, etc.) |
0.1 |
30–90%, <30% is also reviewed |
>90% |
177 and 178 |
Coagulation with alumina |
NAa |
<30% |
>90% |
178 |
Coagulation with iron salt |
0.2 |
60–90% |
>90% |
178 |
Ion exchange resins |
0.2 |
<30% |
>90% |
178 |
Membrane methods (NF and RO) |
0.005 |
Up to 55% |
>90% |
179 |
Membrane distillation |
5 |
Close to 100% |
NAa |
180 |
Membrane distillation |
0.3 to 0.6 |
Over 99.9% |
Over 99.9% |
181 |
Membrane distillation |
Up to 5 |
98.2% |
98.8% |
182 |
Fortunately, membrane distillation had been reported with sufficient removal efficiencies (close to 100%) for As(III) and As(V).180–182 Membrane distillation could play a role in helping the water industry meet the United Nations' Sustainable Development Goals (SDGs).183
Disposal of contaminated biomass
Contaminated biomass from phytoextraction processes is a serious threat to both the environment and human health. Several methods have been proposed for the disposal of heavy metal-contaminated biomass, including composting, compaction, incineration, ashing, pyrolysis, direct disposal, and liquid extraction.184 It should be noted that incineration – the most frequent option – is deemed as an unsafe process due to the emission of arsenic. A safer method for disposal was reported by Carrier et al. 2011,185 based on sub and supercritical water treatments of the contaminated biomass to leach out the maximum arsenic in the liquid phase. Hydrous iron oxides were then used as a sorbent for the recovery of arsenic from the aqueous phase. Recently, the flash pyrolysis biochar method has been recommended for the immobilization and disposal of contaminated biomass.186,187 Pyrolysis is a concept that describes the thermal breakdown of organic compounds in an oxygen-free condition at temperatures ranging from 250 to 900 °C.188 This is a different method of transforming biomass waste into valuation products such as biochar, syngas, and bio-oil. Pyrolysis may be classified into three broad categories based on the operating conditions (heating rate, temperature, residence time, and pressure): (i) slow pyrolysis with a temperature of 300 °C,189 (ii) intermediate pyrolysis at temperatures ranging from 300 to 500 °C,190 and (iii) fast pyrolysis with a heating temperature of over 500 °C.191
Fast pyrolysis is considered a rapid thermochemical process capable of liquefying carbon-based materials to a high-energy liquid bio-oil.191 Fast pyrolysis conditions are defined by the following characteristics: (i) rapid heating of biomass particles (>100 °C min−1)192 (ii) short heating time of structural and pyrolysis fumes (0.5–2 s) at elevated temperatures193 and (iii) moderate pyrolysis treatment temperatures (400–600 °C).194 A critical distinguishing feature of fast pyrolysis development is the requirement to minimize fume residence time in the hot zone to obtain high-quality bio-oil.188 Fast pyrolysis yields more bio-oil by ensuring that vapors are quickly extinguished or cooled.195 The properties of biochar are essentially determined by the feedstocks, temperature, particle size, and heating rate, which directly affect the quality of biochar.196 Meanwhile, the yield of biochar is influenced by the type and conditions of pyrolysis. Biochar has the potential to be a considerable resource for the elimination of harmful contaminants by bonding with functional groups, e.g., hydroxyl and carboxyl groups, on the surface of biochar. The efficiency of biochar varies according to the type of biomass and pyrolysis operations. As a result, biochar appears to be a promising method of pollution removal but needs future development for perfect performance.
Conclusions
Arsenic contamination is a serious issue on a global scale for animal and human health as well as agriculture. Research on As origin, toxicities, mobility, distribution patterns, bioaccumulation, and remediation, the scientific understanding of this metalloid is still evolving, and the study of its interactions should help in the development of methods of safe clean-up and exposure prevention all the way down to the trophic level of plants. In this review, data on natural and anthropogenic sources and the interaction of As with biota in different parts of the World have been presented, together with their main biogeochemical relations. Given that remediation technologies based on conventional physicochemical methods are financially infeasible in developing countries, natural adsorbents (in particular, waste materials/by-products and microalgal and fungal biomass) can be put forward as particularly promising solutions in terms of sustainability and cost-effectiveness. In the panorama presented herein, the latest state of the art on methods of bioaccumulation measurement and remediation that encompass the use of physicochemical and biological processes, i.e., microbes, mosses, lichens, ferns, algae, and macrophytes have been analyzed. Since these bioremediation approaches for the clean-up of this contaminant are still at the initial experimental stages, some have not been recognized at full scale. Nonetheless, extensive research on these primitive plants as bio-accumulators can be instrumental in controlling arsenic exposure and rehabilitation and may result in major progress with a view to solving the problem on a worldwide scale. Further research on As contamination in major crops and animal foods, disposal of contaminated biomass, and installation of arsenic removal systems for contaminated water to minimize its adverse effect spectrum are required.
Data availability
All data are available in this review paper, and further information could be obtained from the corresponding author (https://docs.google.com/document/d/1kz2tTt-zfVyxRTJbbxKtPA-S7LSxPosA/edit?usp=sharing%26ouid=102850718200296775047%26rtpof=true%26sd=true).
Author contributions
Khageshwar Singh Patel: conceptualization, data curation, investigation, methodology, validation, visualization, writing – original draft. Piyush Kant Pandey and Warren T. Corns: investigation, visualization, validation, editing. Pablo Martín-Ramos: data curation, graphic drafting, editing original draft. Simge Varol: software, graphics drafting, editing – original draft. Prosun Bhattacharya: review and editing. Yanbei Zhu: review, editing, submission, and revision.
Conflicts of interest
The authors declare that they have no known competing financial interests or personal relationships that could influence the work reported in this paper.
Acknowledgements
The University Grants Commission (UGC), New Delhi is gratefully acknowledged for providing financial support through BSR grant no. F.18-1/2011(BSR)2016.
References
- L. Han, B. Gao, H. Hao, J. Lu and D. Xu, Sci. Total Environ., 2019, 651, 1983–1991, DOI:10.1016/j.scitotenv.2018.09.381.
- K. F. Akter, G. Owens, D. E. Davey and R. Naidu, Rev. Environ. Contam. Toxicol., 2005, 184, 97–149, DOI:10.1007/0-387-27565-7_3.
- S. Alka, S. Shahir, N. Ibrahim, M. J. Ndejiko, D. V. Vo and F. A. Manan, J. Cleaner Prod., 2021, 278, 123805, DOI:10.1016/j.jclepro.2020.123805.
- Y. C. Jang, Y. Somanna and H. J. I. J. Kim, Source, distribution, toxicity and remediation of arsenic in the environment–a review, Int. J. Appl. Environ. Sci., 2016, 11(2), 559–581 Search PubMed.
- P. K. Srivastava, A. Vaish, S. Dwivedi, D. Chakrabarty, N. Singh and R. D. Tripathi, Sci. Total Environ., 2011, 409, 2430–2442, DOI:10.1016/j.scitotenv.2011.03.002.
- J.-Y. Chung, S.-D. Yu and Y.-S. Hong, J. Prev. Med. Public Health, 2014, 47(5), 253–257, DOI:10.3961/jpmph.14.036.
- N. J. Raju, Environ. Res., 2022, 203, 111782, DOI:10.1016/j.envres.2021.111782.
- A. Aftabtalab, J. Rinklebe, S. M. Shaheen, N. K. Niazi, E. Moreno-Jiménez, J. Schaller and K. H. Knorr, Chemosphere, 2022, 286(Pt 2), 131790, DOI:10.1016/j.chemosphere.2021.131790.
- A. Dadwal and V. Mishra, Clean: Soil, Air, Water, 2017, 45, 7, DOI:10.1002/clen.201600364.
- M. Pigna, A. Caporale, L. Cavalca, A. Sommella and A. Violante, Environ. Eng. Sci., 2015, 32, 551–563, DOI:10.1089/ees.2015.0018.
- J. R. Shaw, B. Jackson, K. Gabor, S. Stanton, J. W. Hamilton and B. A. Stanton, Environ. Toxicol. Chem., 2007, 26, 2704–2709, DOI:10.1897/07-032.1.
- J. Ventura-Lima, M. Reis Bogo and J. M. Monserrat, Ecotoxicol. Environ. Saf., 2011, 74, 211–218, DOI:10.1016/j.ecoenv.2010.11.002.
- Z. Liu and K. Q. Tran, Ecotoxicol. Environ. Saf., 2021, 226, 112821, DOI:10.1016/j.ecoenv.2021.112821.
- N. R. Nicomel, K. Leus, K. Folens, P. Van Der Voort and G. Du Laing, Int. J. Environ. Res. Public Health, 2016, 13, 62, DOI:10.3390/ijerph13010062.
- R. Singh, S. Singh, P. Parihar, V. P. Singh and S. M. Prasad, Ecotoxicol. Environ. Saf., 2015, 112, 247–270, DOI:10.1016/j.ecoenv.2014.10.009.
- X. Wan, M. Lei and T. Chen, Front. Environ. Sci. Eng., 2020, 14, 24, DOI:10.1007/s11783-019-1203-7.
- C. N. Worou, Z. Chen and T. Bacharou, Water Pract. Technol., 2021, 16, 291–319, DOI:10.2166/wpt.2021.018.
- T. G. Asere, C. V. Stevens and G. Du Laing, Sci. Total Environ., 2019, 676, 706–720, DOI:10.1016/j.scitotenv.2019.04.237.
- D. L. Alonso, S. Latorre, E. Castillo and P. F. B. Brandão, Environ. Pollut., 2014, 186, 272–281, DOI:10.1016/j.envpol.2013.12.009.
- M. M. Hussain, J. Wang, I. Bibi, M. Shahid, N. K. Niazi, J. Iqbal, I. A. Mian, S. M. Shaheen, S. Bashir, N. S. Shah, K. Hina and J. Rinklebe, J. Hazard. Mater., 2020, 124027, DOI:10.1016/j.jhazmat.2020.124027.
- X. Meng, R. R. Dupont, D. L. Sorensen, A. R. Jacobson and J. E. McLean, Appl. Geochem., 2016, 66, 250–263, DOI:10.1016/j.apgeochem.2016.01.004.
- S. Shankar, U. Shanker and Shikha, Sci. World J., 2014, 304524, DOI:10.1155/2014/304524.
- N. Garg and P. Singla, Environ. Chem. Lett., 2011, 9, 303–321, DOI:10.1007/s10311-011-0313-7.
- M. K. Upadhyay, A. Shukla, P. Yadav and S. Srivastava, Food Chem., 2019, 276, 608–618, DOI:10.1016/j.foodchem.2018.10.069.
- G. Abbas, B. Murtaza, I. Bibi, M. Shahid, N. K. Niazi, M. I. Khan, M. Amjad, M. Hussain and M. Natasha, Int. J. Environ. Res. Public Health, 2018, 15, 59, DOI:10.3390/ijerph15010059.
- P. M. Finnegan and W. Chen, Front. Physiol., 2012, 3, 182, DOI:10.3389/fphys.2012.00182.
- J. I. Martínez-Castillo, A. Saldaña-Robles and C. Ozuna, Plant Stress, 2022, 3, 100055, DOI:10.1016/j.stress.2022.100055.
- M. M. Rady, A. Kuşvuran, H. F. Alharby, Y. Alzahrani and S. Kuşvuran, J. Plant Growth Reg., 2019, 38, 449–462, DOI:10.1007/s00344-018-9860-5.
- S. Wani, A. Ahmad, S. Hayat and I. Tahir, Environ. Sci. Pollut. Res., 2016, 23, 13413–13423, DOI:10.1007/s11356-016-6533-4.
- H. Khalid, M. Zia-ur-Rehman, A. Naeem, M. U. Khalid, M. Rizwan, S. Ali, M. Umair and M. I Sohail, Solanum nigrum L.: a novel hyperaccumulator for the phyto-management of cadmium contaminated soils, in Cadmium Toxicity and Tolerance in Plants, Academic Press, 2019, pp. 451–477, DOI:10.1016/B978-0-12-814864-8.00018-8.
- T. Komal, M. Mustafa, Z. Ali and A. G. Kazi, Heavy metal uptake and transport in plants, in Heavy metal contamination of soils, Springer, Cham, 2015, pp. 181–194, DOI:10.1007/978-3-319-14526-6_10.
- D. K. Gupta, S. Srivastava, H. G. Huang, M. C. Romero-Puertas and L. M. Sandalio, Arsenic tolerance and detoxification mechanisms in plants, in Detoxification of heavy metals. Springer, Berlin, Heidelberg, 2011, pp. 169–179, DOI:10.1007/978-3-642-21408-0_9.
- N. Mirza, Q. Mahmood, M. Maroof Shah, A. Pervez and S. Sultan, Sci. World J., 2014, 921581, DOI:10.1155/2014/921581.
- C. Navarro, C. Mateo-Elizalde, T. C. Mohan, E. Sánchez-Bermejo, O. Urrutia, M. N. Fernández-Muñiz, J. Paz-Ares, G. Castrillo and A. Leyva, Mol. Plant., 2021, 14, 1489–1507, DOI:10.1016/j.molp.2021.05.020.
- H. F. Bakhat, Z. Zia, S. Abbas, H. M. Hammad, G. M. Shah, S. Khalid, N. Shahid, M. Sajjad and S. Fahad, Groundwater Sustain. Develop., 2019, 9, 100263, DOI:10.1016/j.gsd.2019.100263.
- P. M. Finnegan and W. Chen, Front. Physiol., 2012, 3, 182, DOI:10.3389/fphys.2012.00182.
- S. Pandey, R. Rai and L. C. Rai, Biochemical and molecular basis of arsenic toxicity and tolerance in microbes and plants, in Handbook of arsenic toxicology, Academic Press, 2015, 627–674, DOI:10.1016/B978-0-12-418688-0.00027-7.
- B. Pommerrenig, T. A. Diehn and G. P. Bienert, Plant Sci., 2015, 238, 212–227, DOI:10.1016/j.plantsci.2015.06.002.
- J. Raven and H. Thomas, Curr. Biol., 2010, 20(19), PR837–R839, DOI:10.1016/j.cub.2010.08.031.
- M. Halwart, J. Food Compos. Anal., 2006, 19, 747–751, DOI:10.1016/j.jfca.2006.03.012.
- A. Dradrach, A. Karczewska and K. Szopka, Chemosphere, 2020, 248, 126045, DOI:10.1016/j.chemosphere2020.126045.
- N. S. Klaber and A. V. Barker, Commun. Soil Sci. Plant Anal., 2014, 45, 810–818, DOI:10.1080/00103624.2013.857681.
- R. Sultana, K. Kobayashi and K. H. Kim, Environ. Monit. Assess., 2015, 187, 4101, DOI:10.1007/s10661-014-4101-2.
- R. W. Weaver, J. R. Melton, DeS. Wang and R. L. Duble, Environ. Pollut., Ser. A, 1984, 33, 133–142, DOI:10.1016/0143-1471(84)90173-9.
- J. O. Nriagu, P. Bhattacharya, A. Mukherjee, J. Bundschuh, R. Zevenhoven and R. H. Loeppert, Trace Met. Other Contam. Environ., 2007, 9, 3–60, DOI:10.1016/S1875-1121(06)09001-8.
- L. Liang, Z. Xu, G. Qiu, P. Wu and R. Zhang, Atmos. Pollut. Res., 2019, 10, 455–461, DOI:10.1016/j.apr.2018.09.005.
- K. S. Patel, B. L. Sahu, S. Ramteke and E. Bontempi, J. Environ. Prot., 2016, 7, 689–698, DOI:10.4236/jep.2016.75061.
- M. A. Rahman, H. Hasegawa, M. M. Rahman, M. A. M. Miah and A. Tasmin, Ecotoxicol. Environ. Saf., 2008, 69, 317–324, DOI:10.1016/j.ecoenv.2007.01.005.
- M. S. Kabir, M. A. Salam, D. N. R. Paul, M. I. Hossain, N. M. F. Rahman, A. Aziz and M. A. Latif, Sci. World J., 2016, 2186069, DOI:10.1155/2016/2186069.
- C. Wu, Z. Ye, W. Shu, Y. Zhu and M. Wong, J. Exp. Bot., 2011, 62, 2889–2898, DOI:10.1093/jxb/erq462.
- D. Tattibayeva, C. Nebot, J. M. Miranda, A. Cepeda, E. Mateyev, M. Erkebaev and C. M. Franco, Environ. Geochem. Health, 2016, 38, 85–98, DOI:10.1007/s10653-015-9687-y.
- S. Hensawang and P. Chanpiwat, Environ. Monit. Assess., 2017, 189, 599, DOI:10.1007/s10661-017-6321-8.
- S. M. Praveena and N. A Omar, Food Chem., 2017, 235, 203–211, DOI:10.1016/j.foodchem.2017.05.049.
- N. Fão, S. Nascimento, A. H. de La Cruz, D. Calderon, R. Rocha, T. Saint'Pierre, A. Gioda, F. V. Thiesen, N. Brucker, T. Emanuelli and S. C. Garcia, Drug Chem. Toxicol., 2019, 1–9, DOI:10.1080/01480545.2019.1591435.
- Y. K. Sahu, K. S. Patel, P. Martín-Ramos and E. K. Towett, Eur. J. Med. Plants, 2019, 28, 1–8, DOI:10.9734/EJMP/2019/v28i330137.
- A. Yadav, P. K. Sahu, K. S. Patel, L. Lata, H. Milosh, W. T. Corns, J. Allen and M. P. Ramos, J. Hazard., Toxic Radioact. Waste, 2020, 24, 210296841, DOI:10.1061/(ASCE)HZ.2153-5515.0000478.
- P. Bhattacharya, A. C. Samal, J. Majumdar and S. C. Santra, Water, Air, Soil Pollut., 2010, 213, 3–13, DOI:10.1007/s11270-010-0361-9.
- S. Ramteke, K. S. Patel, B. Blazhev and L. Matini, J. Environ. Prot., 2016, 7, 996–1004, DOI:10.4236/jep.2016.77088.
- K. S. Patel, R. Sharma, N. Dahariya, A. Yadav, B. Blazhev, L. Matini and J. Hoinkis, Am. J. Anal. Chem., 2015, 6, 687–693, DOI:10.4236/ajac.2015.68066.
- R. Kundu, K. Bhattacharyya and S. Pal, J. Spices Aromat. Crops, 2012, 21(2), 125–129 Search PubMed.
- T. Roychowdhury, T. Uchino, H. Tokunaga and M. Ando, Food Chem. Toxicol., 2002, 40, 1611–1621, DOI:10.1016/s0278-6915(02)00104-7.
- S. Pyne and S. C. Santra, Adv. Appl. Sci. Res., 2016, 7(4), 168–175 CAS.
- Md. S. Ahmed, Md. M. H. Biswas, Md. A. Mottalib, M. N. Alam and M. Khan, IOSR J. Environ. Sci., Toxicol. Food Technol., 2019, 13, 59–71, DOI:10.9790/2402-1305015971.
- B. M. Dahal, M. Fuerhacker, A. Mentler, K. B. Karki, R. R. Shrestha and W. E. H. Blum, Environ. Pollut., 2008, 155, 157–163, DOI:10.1016/j.envpol.2007.10.024.
- C. D. Ezeonyejiaku and M. O. Obiakor, J. Health Pollut., 2017, 7, 40–50, DOI:10.5696/2156-9614-7.15.40.
- S. M. Blagojević, S. N. Blagojević and B. M. Begović, Phys. Chem. Technol., 2015, 13, 191–202, DOI:10.2298/FUPCT1503191.
- G. George and B. M. Gqaza, J. Adv. Agric. Technol., 2015, 2, 29–35, DOI:10.12720/joaat.2.1.29-33.
- C. Ubonnuch, S. Ruangwises, W. Gritsanapanand and N. Ruangwises, Bota. Diet. Suppl., 2013, 506389, DOI:10.1155/2013/506389.
- S. W. Al-Rmalli, R. O. Jenkins and P. I. Haris, J. Hazard. Mater., 2011, 190, 69–74, DOI:10.1016/j.jhazmat.2011.02.068.
- R. C. Campbell, W. E. Stephens and A. A. Meharg, Tob. Induced Dis., 2014, 12, 24, DOI:10.1186/s12971-014-0024-5.
- X. Liao, Y. Fu, Y. He and Y. Yang, Catena, 2014, 113, 213–218, DOI:10.1016/j.catena.2013.07.011.
- S. Safiullah, M. Mahamud-Ul-Hoque, M. A. Sabur and M. E. Haque, Asian J. Water, Environ. Pollut., 2013, 10, 23–28 CAS , https://www.researchgate.net/publication/265553257.
- CXS 193-1995: General Standard for Contaminants and Toxins in Food and Feed, Codex Alimentarius Commission International Food Standards, FAO/WHO, 2019. https://www.fao.org/fao-who-codexalimentarius/sh-proxy/en/?lnk=1%26url=https%253A%252F%252Fworkspace.fao.org%252Fsites%252Fcodex%252FStandards%252FCXS%2B193-1995%252FCXS_193e.pdf Search PubMed.
- P. K. Sahoo and K. Kim, Geosci. J., 2013, 17, 107–122, DOI:10.1007/s12303-013-0004-4.
- A. Sharma and S. Pawan, Int. J. Curr. Res., 2016, 8, 26027–26030 Search PubMed.
- L. M. Walsh, M. E. Sumner and D. E. Keeney, Environ. Health Perspect., 1977, 19, 67–71, DOI:10.1289/ehp.771967.
- F. A. McClure, The bamboos: A fresh perspective, Harvard University Press, 1966 Search PubMed.
- R. Zhao, M. Zhao, H. Wang, Y. Taneike and X. Zhang, Sci. Total Environ., 2006, 371, 293–303, DOI:10.1016/j.scitotenv.2006.03.019.
- B. Kumari, V. Kumar, A. K. Sinha, J. Ahsan, A. K. Ghosh, H. Wang and G. DeBoeck, Environ. Chem. Lett., 2017, 15, 43–64, DOI:10.1007/s10311-016-0588-9.
- J. M. Neff, Arsenic in the Ocean, In book: Bioaccumulation in marine organisms, 2002. https://doi.org/ DOI:10.1016/B978-008043716-3/50004-X.
- K. A. Francesconi and J. S. Edmonds, Adv. Inorg. Chem., 1996, 44, 147–189, DOI:10.1016/S0898-8838(08)60130-0.
- V. Taylor, B. Goodale, A. Raab, T. Schwerdtle, K. Reimer, S. Conklin, M. R. Karagas and K. A. Francesconi, Sci. Total Environ., 2017, 580, 266–282, DOI:10.1016/j.scitotenv.2016.12.113.
- I. Kalantzi, K. Mylona, K. Sofoulaki, K. M. Tsapakis and S. A. Pergantis, J. Environ. Sci., 2017, 56, 300–312, DOI:10.1016/j.jes.2017.03.033.
- M. Mania, M. Rebeniak, T. Szynal, M. Starska, K. Ledzion, E. Wojciechowska-Mazurek and J. Postupolski, Rocz. Panstw. Zakl. Hig., 2015, 66, 203–210 CAS.
- Z. Šlejkovec, A. Stajnko, I. Falnoga, L. Lipej, D. Mazej, M. Horvat and J. Faganeli, Int. J. Mol. Sci., 2014, 15(12), 22073–22091, DOI:10.3390/ijms151222073.
- G. Caumette, I. Koch, E. Estrada and K. J. Reimer, Environ. Sci. Technol., 2011, 45(23), 9917–9923, DOI:10.1021/es2025092.
- D. Guimarães, A. A. Roberts, M. W. Tehrani, R. Huang, L. Smieska, A. R. Woll, S. Lin and P. J. Parsons, J. Anal. At. Spectrom., 2018, 33, 1616–1630, 10.1039/C8JA00094H.
- P. K. Krishnakumar, M. A. Qurban, M. Stiboller, K. E. Nachman, T. V. Joydas, K. P. Manikandan, S. A. Mushir and K. A. Francesconi, Sci. Total Environ., 2016, 566–567, 1235–1244, DOI:10.1016/j.scitotenv.2016.05.180.
- A. Hassan, C. Rylander, M. Brustad and T. M. Sandanger, Int. J. Circumpolar Health, 2012, 71, 18187, DOI:10.3402/ijch.v71i0.18187.
- B. K. Datta, M. K. Bhar, P. H. Patra, D. Majumdar, R. R. Dey, S. Sarkar, T. K. Mandal and A. K. Chakraborty, Toxicol. Int., 2012, 19, 59–62, DOI:10.4103/0971-6580.94511.
- T. Rana, A. K. Bera, D. K. Mondal, S. D. Ghosh, D. Bhattacharya, S. Samanta, D. Pan and S. Das, Toxico. Ind. Health, 2014, 30, 576–580, DOI:10.1177/0748233712462467.
- J. C. Akan, F. I. Abdulrahman, O. A. Sodipo and Y. A. Chiroma, Res. J. Appl. Sci. Eng. Technol., 2010, 2, 743–748 CAS.
- M. S. Islam, J. Food Sci. Technol., 2018, 2, 218–227 Search PubMed.
- I. Mariam, S. Iqbal and S. A. Nagra, Int. J. Agri. Biol., 2004, 5, 816–820 Search PubMed.
- M. Moriarty, I. Koch and K. J. Reimer, Environ. Sci.: Processes Impacts, 2013, 15, 1520–1526, 10.1039/c3em00223c.
- J. Burger, M. Gochfeld, C. Jeitner, R. Zappalorti, T. Pittfield and E. DeVito, Arch. Environ. Contam. Toxicol., 2017, 72, 586–595, DOI:10.1007/s00244-017-0398-5.
- K. R. Holzwart, T. Campbell and J. Burger, Arch. Environ. Contam. Toxicol., 2005, 49, 239–248, DOI:10.1007/s00244-004-0200-3.
- T. Agusa, K. Takagi, R. Kubota, Y. Anan, H. Iwata and S. Tanabe, Environ. Pollut., 2008, 153, 127–136, DOI:10.1016/j.envpol.2007.07.013.
- P. Mandal, Emerging Contam., 2017, 3, 17–22, DOI:10.1016/j.emcon.2017.01.004.
- M. Arianejad, M. Alizadeh, A. Bahrami and S. R. Arefhoseini, Health Promot. Perspect., 2015, 5, 176–182, DOI:10.15171/hpp.2015.021.
- F. Samiee, M. Leili, J. Faradmal, Z. Torkshavand and G. Asadi, Food Control, 2019, 106, 106669, DOI:10.1016/j.foodcont.2019.05.034.
- Mi. Stiboller, G. Raber, E. L. F. Gjengedal, M. Eggesbø and K. A. Francesconi, Anal. Chem., 2017, 89, 6265–6271, DOI:10.1021/acs.analchem.7b01276.
- T. G. Kazi, K. D. Brahman, H. I. Afridi, M. B. Arain, F. N. Talpur and A. Akhtar, Chemosphere, 2016, 165, 427–433, DOI:10.1016/j.chemosphere.2016.09.015.
- M. Bassil, F. Daou, H. Hassan, O. Yamani, J. A. Kharma, Z. Attieh and J. Elaridi, Chemosphere, 2018, 191, 911–921, DOI:10.1016/j.chemosphere.2017.10.111.
- C. Ulman, S. Gezer, Ö. Anal, I. R. Töre and Ü. Kirca, Water, Air, Soil Pollut., 1998, 101, 411–416, DOI:10.1023/A:1004990721068.
- S. M. Ghosh, M. A. Awal and R. Rao, Arch. Environ. Contam. Toxicol., 2013, 64, 151–159, DOI:10.1007/s00244-012-9810-3.
- J. D. Stanković, A. D. Sabovljević and M. S. Sabovljević, Acta Bot. Croat., 2018, 77, 109–118, DOI:10.2478/botcro-2018-0014.
- E. Indriolo, G. N. Na, D. Ellis, D. E. Salt, J. A. Banks and A. Vacuolar, Plant Cell, 2010, 22, 2045–2057, DOI:10.1105/tpc.109.069773.
- G. Macedo-Miranda, P. Avila-Pérez, P. Gil-Vargas, G. Zarazú, J. C. Sánchez-Meza, C. Zepeda-Gómez and S. Tejeda, Accumulation of heavy metals in mosses: a biomonitoring study, Springer Plus, 2016, vol. 5, p. 715, DOI:10.1186/s40064-016-2524-7.
- L. Molina and A. Segura, Biochemical and metabolic plant responses toward polycyclic aromatic hydrocarbons and heavy metals present in atmospheric pollution, Plants, 2021, 10(11), 2305 CrossRef CAS PubMed.
- L. Liang, Z. Xu, G. Qiu, P. Wu and R. Zhang, Atmos. Pollut. Res., 2019, 10, 455–461, DOI:10.1016/j.apr.2018.09.005.
- Y. Suzuki, C. Takenaka, R. Tomioka, H. Tsubota, Y. Takasaki and T. Umemura, Mine Water Environ., 2016, 35, 265–272, DOI:10.1007/s10230-015-0335-7.
- R. Bajpai, D. K. Upreti and S. K. Dwivedi, Environ. Monit. Assess., 2009, 159, 437–442, DOI:10.1007/s10661-008-0641-7.
- R. Bajpai, G. K. Mishra, S. Mohabe, D. K. Upreti and S. Nayaka, J. Environ. Biol., 2011, 32, 195–199 CAS.
- R. Bajpai and D. K. Upreti, Ecotoxicol. Environ. Saf., 2012, 83, 63–70, DOI:10.1016/j.ecoenv.2012.06.001.
- D. Kuehnelt, J. Lintschinger and W. Goessler, Appl. Organomet. Chem., 2000, 14, 411–420, DOI:10.1002/1099-0739(200008)14:8<411::AID-AOC24>3.0.CO;2-M.
- A. Meharg, New Phytol., 2003, 157, 25–31, DOI:10.1046/j.1469-8137.2003.00541.x.
- C.-Y. Wei and T.-B. Chen, Chemosphere, 2006, 63, 1048–1053, DOI:10.1016/j.chemosphere.2005.09.061.
- C. Y. Wei, C. Wang, X. Sun and W. Y. Wang, Environ. Geochem. Health, 2007, 29, 169–177, DOI:10.1007/s10653-006-9046-0.
- X. Wei, Y. Zhou, D. C. W. Tsang, L. Song, C. Zhang, M. Yin, J. Liu, T. Xiao, G. Zhang and J. Wang, J. Hazard. Mater., 2020, 388, 121756, DOI:10.1016/j.jhazmat.2019.121756.
- Y. Wang, S. Wang, P. Xu, C. Liu, M. Liu, Y. Wang, C. Wang, C. Zhang and Y. Ge, Rev. Environ. Sci. Biotechnol., 2015, 14, 427–451, DOI:10.1007/s11157-015-9371-9.
- N. Singh, A. Raj, P. B. Khare, R. D. Tripathi and S. Jamil, Bioresour. Technol., 2010, 101, 8960–8968, DOI:10.1016/j.biortech.2010.06.116.
- R. Feng, X. Wang, C. Wei and S. Tu, Int. J. Phytoremediation, 2015, 17, 348–354, DOI:10.1080/15226514.2013.773281.
- S. Majumdar, S. Benerjee, A. Paul and S. C. Santra, Proceeding of 3rd Inter. Conf. on Ecotoxicology and Environmental Sci., Kalyani, West Bengal, India, 2011 Search PubMed.
- L. Q. Ma, K. M. Komar, C. Tu, W. Zhang, Y. Cai and E. D. Kennelley, Nature, 2001, 409, 579, DOI:10.1038/35054664.
- Y. K. Sahu, M. K. Deb, K. S. Patel, P. Martín-Ramos, E. K. Towett and M. Tarkowska-Kukuryk, J. Hazard., Toxic Radioact. Waste, 2020, 24, 05019007, DOI:10.1061/(ASCE)HZ.2153-5515.0000481.
- A. Pell, A. Márquez, J. F. López-Sánchez, R. Rubio, M. Barbero, S. Stegen, F. Queirolo and P. Díaz-Palma, Chemosphere, 2013, 90, 556–564, DOI:10.1016/j.chemosphere.2012.08.028.
- Z. Maa, L. Linc, M. Wua, H. Yua, T. Shanga, T. Zhanga and M. Zhaoa, Aquacult, 2018, 497, 49–55, DOI:10.1016/j.aquaculture.2018.07.040.
- C. Almela, S. Algora, V. Benito, M. J. Clemente, V. Devesa, M. A. Súñer, D. Vélez and R. Montoro, J. Agric. Food Chem., 2002, 50, 918–923, DOI:10.1021/jf0110250.
- S. M. Shamsuddoha, A. Bulbul and S. M. Imamul Huq, Bangladesh J. Microbiol., 2005, 22, 148–151 Search PubMed.
- S. Maeda, S. Nakashima, T. Takeshita and S. Higashi, Sep. Sci. Technol., 1987, 20, 153–161, DOI:10.1080/01496398508058356.
- P.-Y. Xue and C.-Z. Yan, Chemosphere, 2011, 85, 1176–1181, DOI:10.1016/j.chemosphere.2011.09.051.
- P. Xue, C. Yan, G. Sun and Z. Luo, Environ. Sci. Pollut. Res., 2012, 19, 3969–3976, DOI:10.1007/s11356-012-0856-6.
- D. A. M. Barznji, Limnol. Rev., 2015, 15, 15–20, DOI:10.2478/limre-2015-0002.
- G. Yang, H. Zhong, X. Liu, C. Liu, S. Li, L. Hou, Y. Liu, Y. Wang, W. Ren and C. Duan, Bull. Environ. Contam. Toxicol., 2020, 104, 358–365, DOI:10.1007/s00128-020-02796-y.
- H. V. Khang, M. Hatayama and I. Chihiro, Environ. Exp. Bot., 2012, 83, 47–52, DOI:10.1016/j.envexpbot.2012.04.008.
- J. P. Olszewska, A. A. Meharg, K. V. Heal, M. Carey, I. D. M. Gunn, K. R. Searle, I. J. Winfield and B. M. Spears, Environ. Sci. Technol., 2016, 50, 9044–9052, DOI:10.1021/acs.est.6b00942.
- S. Intamat, P. Buasriyot, M. Sriuttha, B. Tengjaroenkul and L. Neeratanaphan, Int. J. Environ. Stud., 2017, 74, 303–314, DOI:10.1080/00207233.2017.1284382.
- Y. K. Sahu, K. S. Patel, P. Martín-Ramos, M. Rudzińska, P. Górnaś, E. K. Towett, J. Martín-Gil and M. Tarkowska-Kukuryk, Environ. Monit. Assess., 2020, 192, 38, DOI:10.1007/s10661-019-8001-3.
- G. Sarret, S. Guédron, D. Acha, S. Bureau, F. Arnaud-Godet, D. Tisserand, M. Goni-Urriza, C. Gassie, C. Duwig, O. Proux and A.-M. Aucour, Bolivia. Sci. Rep., 2019, 9, 10626, DOI:10.1038/s41598-019-47183-8.
- P. Favas, J. Pratas and M. Prasad, Sci. Total Environ., 2012, 433, 390–397, DOI:10.1016/j.scitotenv.2012.06.091.
- J. Olowoyo, E. van Heerden and J. L. Fischer, Environ. Sci. Pollut. Res. Int., 2011, 18, 663–668, DOI:10.1007/s11356-010-0410-3.
- F. J. Zhao, S. J. Dunham and S. P. McGrath, New Phytol., 2002, 156, 27–31, DOI:10.1046/j.1469-8137.2002.00493.x.
- B. Koz, N. Celik and U. Cevik, Ecol. Indic., 2010, 10, 762–765, DOI:10.1016/j.ecolind.2009.11.006.
- M. M. Farinha, Z. Šlejkovec, J. T. van Elteren, H. Th. Wolterbeek and M. C. Freitas, J. Atmos. Chem., 2004, 49, 343–353, DOI:10.1007/s10874-004-1248-1.
- S. G. Prabhu, G. Srinikethan and S. Hegde, Int. J. Res. Eng. Technol., 2016, 5, 1–9 Search PubMed.
- M. I. S. Gonzaga, J. A. G. Santos and L. Q. Ma, Review, Sci. Agric., 2006, 63, 90–101, DOI:10.1590/S0103-90162006000100015.
- R. R. Klopper, Pteridoforum, 2011, 96, 1–5 Search PubMed.
- H. B. Wang, Z. H. Ye, W. S. Shu, W. C. Li, M. H. Wong and C. Y. Lan, Int. J. Phytoremediation, 2006, 8, 1–11, DOI:10.1080/16226510500214517.
- O. Spain, M. Plöhn and C. Funk, Physiol. Plant., 2021, 173, 526–535, DOI:10.1111/ppl.13405.
- O. Dìaza, R. Pasteneb, F. Encina-Montoyac, R. Vegad and C. Oberti-Grassauc, Compr. Anal. Chem., 2019, 85, 247–265, DOI:10.1016/bs.coac.2019.04.002.
- M. A. Rahman and H. Hasegawa, Chemosphere, 2011, 83, 633–646, DOI:10.1016/j.chemosphere.2011.02.045.
- M. N. V. Prasad, Prospects for manipulation of molecular mechanisms and transgenic approaches in aquatic macrophytes for remediation of toxic metals and metalloids in wastewaters, in Transgenic plant technology for remediation of toxic metals and metalloids, Academic Press, 2019, ch. 19, pp. 395–428, DOI:10.1016/C2017-0-01241-7.
- X. Zhang, F. J. Zhao, Q. Huang, P. N. Williams, G. X. Sun and Y. G. Zhu, New Phytol., 2009, 182, 421–428, DOI:10.1111/j.1469-8137.2008.02758.x.
- J. Pratas, P. Favas, N. Rodrigues and M. N. V. Prasad, Arsenic accumulation in aquatic plants (Central Portugal), in Advances in Waste Management, ed. A. Kallel, A. Hassairi, C. A. Bulucea and N. Mastorakis, WSEAS Press, 2010 Search PubMed.
- M. Bissen and F. H. Frimmel, Acta Hydrochim. Hydrobiol., 2003, 31, 97–107, DOI:10.1002/aheh.200300485.
- C. Wang, J. Luan and C. Wu, Water Res., 2019, 158, 370–382, DOI:10.1016/j.watres.2019.04.043.
- R. Amen, H. Bashir, I. Bibi, S. M. Shaheen, N. K. Niazi, M. Shahid, M. M. Hussain, V. Antoniadis, M. B. Shakoor, S. G. Al-Solaimani and H. Wang, Chem. Eng. J., 2020, 396, 125195, DOI:10.1016/j.cej.2020.125195.
- M. I. Din, A. G. Nabi, Z. Hussain, M. Arshad, A. Intisar, A. Sharif, E. Ahmed, H. A. Mehmood and M. L. Mirza, Crit. Rev. Anal. Chem., 2019, 49, 534–541, DOI:10.1080/10408347.2018.1564647.
- S. Lata and S. R. Samadder, J. Environ. Manage., 2016, 166, 387–406, DOI:10.1016/j.jenvman.2015.10.039.
- S. I. Siddiqui, M. Naushad and S. A. Chaudhry, Process Saf. Environ. Prot., 2019, 126, 60–97, DOI:10.1016/j.psep.2019.03.037.
- T. A. Siddique, N. K. Dutta and N. Roy Choudhury, Nanomaterials, 2020, 10, E1323, DOI:10.3390/nano10071323.
- N. A. A. Qasem, R. H. Mohammed and D. U. Lawal, npj Clean Water, 2021, 4, 36, DOI:10.1038/s41545-021-00127-0.
- K. T. Lim, M. Y. Shukor and H. Wasoh, Res. Int., 2014, 503784, DOI:10.1155/2014/503784.
- X. Wan, M. Lei and T. Chen, Front. Environ. Sci. Eng., 2020, 14, 24, DOI:10.1007/s11783-019-1203-7.
- L. Liu, W. Li, W. Song and M. Guo, Sci. Total Environ., 2018, 633, 206–219, DOI:10.1016/j.scitotenv.2018.03.161.
- K. Dwivedi, S. Srivastava, S. Dwivedi and V. Tripathi, Hydrol.: Curr. Res., 2015, 6, 186, DOI:10.4172/2157-7587.1000186.
- A. Nair, A. A. Juwarkar and S. K. Singh, Water, Air, Soil Pollut., 2007, 180, 199–212, DOI:10.1007/s11270-006-9263-2.
- C. Bergqvist and M. Greger, Phytostabilization of arsenic, in In Situ remediation of arsenic-contaminated sites, 2014, pp. 53–67, DOI:10.1201/b17619-4.
- R. J. Ampiah-Bonney, J. F. Tyson and G. R. Lanza, Int. J. Phytorem., 2007, 9, 31–40, DOI:10.1080/15226510601139383.
- M. Sakakibara, A. Watanabe, M. Inoue, S. Sano and T. Kaise, Proceedings of the Annual Intl. Conf. Soils, Sediments, Water and Energy, 2010, vol. 12, p. 26, https://scholarworks.umass.edu/soilsproceedings/vol12/iss1/26 Search PubMed.
- M. I. Silva Gonzaga, J. A. Gonzaga Santos and L. Q. Ma, Sci. Agri., 2006, 63, 90–101, DOI:10.1590/S0103-90162006000100015.
- S. Singh and D. P. Fulzele, Int. J. Phytorem., 2021, 23, 1310–1318, DOI:10.1080/15226514.2021.1895717.
- S. Tiwari, B. K. Sarangi and R. A. Pandey, Environ. Eng. Res., 2014, 19, 145–149, DOI:10.4491/eer.2014.19.2.14.
- V. Tangahu, S. R. S. Abdullah, H. Basri, M. Idris, N. Anuar and M. Mukhlisin, Int. J. Chem. Eng., 2011, 939161, DOI:10.1155/2011/939161.
- T. Q. Nguyen, V. Sesin, A. Kisiala and R. J. N. Emery, Environ. Toxicol. Chem., 2021, 40, 7–22, DOI:10.1002/etc.4909.
- T. S. Y Choong, T. G. Chuah, Y. Robiah, K. F. L. Gregory and I. Azni, Desalination, 2007, 217, 139–166 CrossRef.
- R. Johnston and H. Heijnen, Safe Water Technology for Arsenic Removal, WHO, 2001 Search PubMed.
- R. Irunde, J. Ijumulana, F. Ligate, J. P. Maity, A. Ahmad, J. Mtamba, F. Mtalo and P. Bhattacharya, Groundwater Sustain. Develop., 2022, 18, 100746, DOI:10.1016/j.gsd.2022.100746.
- A. Alkhudhiri, M. Hakami, M. P. Zacharof, H. Abu Homod and A. Alsadun, Water, 2020, 12, 1574, DOI:10.3390/w12061574.
- S. Leaper, E. O. A. Caceres, J. M. Luque-Alled, S. H. Cartmell and P. Gorgojo, ACS Appl. Energy Mater., 2021, 3, 1854–1865, DOI:10.1021/acsapm.0c01402.
- S. Santoro, P. Timpano, A. H. Avci, P. Argurio, F. Chidichimo, M. De Biase, S. Straface and E. Curcio, Desalination, 2021, 520, 115378, DOI:10.1016/j.desal.2021.115378.
- N. M. A. Omar, M. H. D. Othman, Z. S. Tai, A. O. A. Amhamed, T. A. Kurniawan, M. H. Puteh and M. N. M. Sokri, J. Water Process Eng., 2023, 52, 103504, DOI:10.1016/j.jwpe.2023.103504.
- Y. Wang, S. Wang, P. Xu, C. Liu, M. Liu, Y. Wang, C. Wang, C. Zhang and Y. Ge, Rev. Environ. Sci. Biotechnol., 2015, 14, 427–451, DOI:10.1007/s11157-015-9371-9.
- M. Carrier, A. Loppinet-Serani, C. Absalon, F. Marias, C. Aymonier and M. Mench, Biomass Bioenergy, 2011, 35, 872–883, DOI:10.1016/j.biombioe.2010.11.007.
- D. Ghosh and S. K. Maiti, Int. J. Phytoremediation, 2021, 23, 559–576, DOI:10.1080/15226514.2020.1840510.
- Y. Li, H. Yu, L. Liu and H. Yu, J. Hazard. Mater., 2021, 420, 126655, DOI:10.1016/j.jhazmat.2021.126655.
- J. Wang and S. Wang, J. Cleaner Prod., 2019, 227, 1002–1022, DOI:10.1016/j.jclepro.2019.04.282.
- K. Kameyama, T. Miyamoto and Y. Iwata, Material, 2019, 12, 1732, DOI:10.3390/ma12111732.
- O. J. Oginni, Characteristics of activated carbons produced from herbaceous biomass feedstock, West Virginia University, 2018, DOI:10.33915/etd.3719.
- J. H. Ha and I. G. Lee, Processes, 2020, 8, 1407, DOI:10.3390/pr8111407.
- V. Dhyani and T. Bhaskar, Renew. Energy, 2020, 129, 695–716, DOI:10.1016/j.renene.2017.04.035.
- R. Wahi, N. F. Q. A. Zuhaidi, Y. Yusof, J. Jamel, D. Kanakaraju and Z. Ngaini, Biomass Bioenergy, 2017, 107, 411–421, DOI:10.1016/j.biombioe.2017.08.007.
- G. Daful, M. R. Chandraratne, H. College, A. Dhabi and U. A. Emirates, Biochar production from biomass waste-derived material, in Encyclopedia of Renewable and Sustainable Materials, 2018, DOI:10.1016/B978-0-12-803581-8.11249-4.
- J. Bedia, M. Peñas-Garzón, A. Gómez-Avilés, J. J. Rodriguez and C. Belver, C: J. Carbon Res., 2018, 4, 63, DOI:10.3390/c4040063.
- W. Ao, J. Fu, X. Mao, Q. Kang, C. Ran, Y. Liu, Z. Gao, H. Zhang, J. Li, G. Liu and J. Dai, Renewable Sustainable Energy Rev., 2018, 92, 958–979, DOI:10.1016/j.rser.2018.04.051.
|
This journal is © The Royal Society of Chemistry 2023 |
Click here to see how this site uses Cookies. View our privacy policy here.