DOI:
10.1039/D3RA01987J
(Review Article)
RSC Adv., 2023,
13, 17476-17494
Bismuth tungstate Bi2WO6: a review on structural, photophysical and photocatalytic properties
Received
26th March 2023
, Accepted 2nd June 2023
First published on 9th June 2023
Abstract
This review paper provides a comprehensive overview of the recent trends in bismuth tungstate (Bi2WO6) research, covering its structural, electrical, photoluminescent, and photocatalytic properties. The structural characteristics of bismuth tungstate are explored in detail, including its different allotropic crystal structures with respect to its isotypic materials. The electrical properties of bismuth tungstate, such as its conductivity and electron mobility, are also discussed, along with its photoluminescent properties. The photocatalytic activity of bismuth tungstate is a particular focus, with recent advances in doping and co-doping strategies with metals, rare earth and other elements summarized. The limitations and challenges of using bismuth tungstate as a photocatalyst are also examined, such as its low quantum efficiency and susceptibility to photodegradation. Finally, recommendations for future research directions are provided, including the need for further studies on the underlying mechanisms of photocatalytic activity, the development of more efficient and stable bismuth tungstate-based photocatalysts, and the exploration of new applications in fields such as water treatment and energy conversion.
1. Introduction
Bismuth tungstate (Bi2WO6), also known as BWO, is a highly sought-after material due to its numerous applications in the production of electronic components. This material is versatile and can be used for a range of purposes including capacitors, humidity detectors, optical sensors, and thermistors.1–3
This material belongs to the family of Aurivillius phases (n = 1) of the general formula Bi2An−1BnO3n+1 (with A = Ca, Sr, Ba, Pb, Bi, Na, K and B = Ti, Nb, Ta, Mo, W, Fe).4 The structure consists of alternating fluorine-like [Bi2O2]2+ ionic layers and [WO4]2− ionic layers, linked together to form “n” perovskite-like units An−1BnO3n+1. Its photocatalytic properties under visible irradiation were discovered in 1999 for the production of dioxygen by oxidation of water5 and then exploited from 2003, for the mineralization of organic molecules (chloroform and acetaldehyde) by Ye and his team.6 Its photocatalytic activity has since been widely used to degrade a wide range of molecules, in gaseous or aqueous form, or even microorganisms under visible irradiation.7,8 Bi2WO6 has indeed a suitable crystal and electronic structure for photocatalysis under visible light. The tungstate Bi2WO6 has orthorhombic symmetry, and its structure is composed of alternating Bi2O2 sheets and layers of WO6 octahedra linked together by the vertices. This stacking of alternately charged (+) and (−) sheets creates an electric field parallel to the (001) plane of the sheets that promotes the mobility of photo-generated charges in the material and facilitates the adsorption of molecules on the surface.9,10 Several other semiconductors have also been utilized as photocatalysts, including ZnO, CeO2, ZrO2, SnO2, CdS, and ZnS. However, titanium dioxide (TiO2) has emerged as a popular choice for environmental remediation due to its chemical stability, low cost, and strong oxidizing power, as documented in studies conducted by ref. 11–14. Nonetheless, TiO2 has certain limitations, such as its high gap (Eg = 3.2 eV), which restricts UV radiation absorption to only 4% of the solar spectral band, or 387.5 nm, as observed in studies by ref. 4, 15 and 16.
Since its initial study by Knight,17 Bi2WO6 has garnered significant attention owing to its exceptional physical and chemical characteristics, including ferroelectricity, piezoelectricity, catalytic properties, and nonlinear dielectric susceptibility, as highlighted in research conducted by ref. 17 and 18. With a gap of 2.8 eV, Bi2WO6 can absorb longer wavelengths, making it ideal for solar-related applications. Despite ongoing research into Bi2WO6 and its derivatives, the field remains largely underdeveloped.10
It is noteworthy that a series of review articles have been recently published on the photocatalytic performance of Bi2WO6 and its application in environmental remediation. For example, in 2019, Yi and his team.19, described the latest fabrication approaches for Bi2WO6 and even discussed the mechanism of the various morphologies of Bi2WO6. It was interesting to note that the photocatalytic activities of three bismuth-based photo-catalysts (Bi2WO6, Bi2MoO6 and BiVO4) were also assessed in a review by Liu et al.20 In the same context chen et al.21, reviewed the fundamental photocatalytic principles of BWO as well as material modification approaches to enhance the photocatalytic capabilities of BWO, comprising atomic modulation, morphology control and composite manufacturing. This current review reports the advances and the recent applications, electrical and photoluminescent properties of BWO materials as well as the photocatalytic properties of bare and doped BWO photocatalysts.
2. Synthesis and characteristics of Bi2WO6 photocatalyst
Bi2WO6 is categorized as a layered perovskite-type oxide compound, consisting of bismuth (Bi), tungsten (W), and oxygen (O). Its distinctive structural and physicochemical properties have generated substantial interest. The dimensional morphology of Bi2WO6 is closely linked to its physical structure and shape.22 Understanding the influence of various synthesis methods and morphologies on Bi2WO6 is crucial, as they have a significant impact on its characterization and properties. Therefore, gaining insight into how different synthesis methods and conditions can yield diverse morphologies of Bi2WO6 is vital for a comprehensive understanding of its characterization. It is worth noting that each synthesis method possesses its own set of advantages and disadvantages. Presented below are some commonly employed synthesis methods.
2.1. Solid-state synthesis
This method utilizes easily accessible precursors and is characterized by its simplicity and cost-effectiveness. It involves the direct solid-state reaction between bismuth oxide (Bi2O3) and tungsten oxide (WO3) at elevated temperatures.23 However, the use of high temperatures can influence the crystal structure, potentially leading to particle aggregation and the formation of larger crystals. This, in turn, can have implications for the material's optical, electrical, and catalytic properties, as well as its potential applications.24
2.2. Hydrothermal/solvothermal method
This process utilizes either an autoclave or a sealed container to facilitate the reaction between bismuth and tungsten precursors in a water-based solution at high pressure and temperature. By employing this technique, precise control over the particle size, shape, and crystal structure is achieved, resulting in enhanced properties and the formation of Bi2WO6.25,26 However, it should be noted that this method requires specialized equipment and longer reaction times when compared to alternative approaches. The solvothermal method,27 which is similar to the hydrothermal technique, offers another option. It allows for better regulation of the particle size, shape, and crystal structure of Bi2WO6 through the manipulation of factors such as reaction temperature, duration, precursor concentration, and pH. Nonetheless, the solvothermal method also calls for specialized equipment and longer reaction durations.
2.3. Sol–gel method
In this technique, a colloidal suspension (sol) is formed, which later undergoes gelation to create a solid network. The sol–gel method allows for effective management of composition, purity, and homogeneity. It also offers the possibility of incorporating dopants and customizing properties. However, it is important to note that this process is relatively intricate, involving multiple steps. Longer processing times are necessary for both gel formation and subsequent thermal treatment.28
2.4. Microwave-assisted
Synthesis involves utilizing microwave irradiation to heat a reaction mixture that contains bismuth and tungsten precursors.29 This approach provides several advantages, such as rapid heating and shorter reaction times. Additionally, it results in enhanced crystallinity and improved phase purity when compared to conventional methods. However, there are some drawbacks to consider. The scalability of this technique for large-scale production is limited. It is crucial to optimize the reaction parameters carefully to prevent overheating or the formation of localized hotspots.
The selection of a synthesis method depends on specific requirements, desired properties, and available resources. Factors such as cost, scalability, purity, control over morphology, and crystallinity are typically considered by researchers to determine the most suitable approach. In order to enhance photocatalytic activity, various structures of Bi2WO6 photocatalysts, including three-dimensional, two-dimensional, one-dimensional, and zero-dimensional, have been successfully synthesized using these methods. These photocatalysts have demonstrated impressive effectiveness across a wide range of applications. Among different metal oxide semiconductors responsive to visible light, bismuth-based catalysts, particularly bismuth tungstate (Bi2WO6), have shown significant potential in energy production and environmental treatment. Bi2WO6 has gained substantial attention for its efficient removal of organic pollutants from wastewater through photocatalysis. With a suitable band gap energy of 2.8 eV, Bi2WO6 effectively absorbs photons in the visible light region. While efforts have been made to improve the photocatalytic performance of semiconductors by incorporating rare-earth metals, Bi2WO6 benefits from its inherent properties, reducing the need for complex methodologies. Table 1 provides a summary comparing Bi2WO6 with common Bismuth-based catalysts and recently employed photocatalysts. While other bismuth-based catalysts may have advantages in specific applications, Bi2WO6's favorable band gap, visible light absorption, high performance, inherent properties, and broad applicability make it a preferred choice for many researchers and practitioners in the field. Additionally, the choice of BWO is explained by its simple synthesis methods with different morphologies and shapes, easiness to remediate its drawbacks of rapid recombination of charge carrier using, cocatalysts, different morphologies and shapes, doping, co-doping etc.
Table 1 Comparing Bi2WO6 with common bismuth-based catalysts, their advantages, and disadvantages
Bismuth-based catalysts |
Materials |
Advantages |
Disadvantages |
Environmental application |
Ref. |
Bismuth vanadate |
BiVO4 |
Low band gap, excellent dispersibility, non-toxic nature, corrosion resistance, and exceptional photocatalytic performance in degrading organic pollutants under visible light |
Overall photocatalytic activity is limited by the relatively low mobility of charge carriers it exhibits |
Water splitting, photocatalytic degradation of pollutants, solar fuel production, antibacterial applications, air purification |
30 and 31 |
Bismuth molybdate |
Bi2MoO6 |
Being a visible light-responsive catalyst used in photocatalytic applications such as water purification and pollutant degradation with a good photocatalytic efficiency and stability |
Its main limitation is its relatively wide bandgap, which limits its absorption of visible light and reduces overall efficiency |
Photocatalysis, water treatment, air purification, solar fuel, production, energy storage |
32 |
Bismuth-based perovskites |
BiFeO3 |
Exhibit exceptional stability and offer a broad spectrum of multifunctional properties. Including water splitting, CO2 reduction, and catalytic energy storage |
The synthesis of bismuth-based perovskites presents challenges, and their overall efficiency can be constrained by defects and surface reactivity, which have an impact on their performance |
Photocatalysis, water splitting, solar cells |
33 |
BiMnO3 |
Wastewater treatment, gas sensors, energy storage |
Bismuth-based zeolites |
Bismuth-containing ZSM-5 zeolite |
In the processes of NOx reduction and hydrocarbon conversion, these catalysts demonstrate notable activity and exhibit a high level of selectivity |
The synthesis process of these catalysts can involve complexity, and various factors such as metal loading, acidity, and reaction conditions can influence their catalytic performance |
Catalytic applications, adsorption and separation, environmental sensing, energy storage and conversion |
34 |
Bismuth-containing beta zeolite |
Bismuth-exchanged Y zeolite |
Bismuth-containing MFI zeolite |
Bismuth tungstate |
Bi2WO6 |
Suitable band gap exhibits responsiveness to visible light, demonstrates exceptional effectiveness in achieving photocatalytic efficacy, and maintains good chemical and thermal stability |
Bi2WO6 exhibits a tendency towards rapid charge carrier recombination, which can detrimentally impact its overall photocatalytic efficiency. Achieving efficient charge separation and effectively suppressing recombination pathways are pivotal factors in enhancing the catalytic performance of Bi2WO6 |
Photocatalysis, antibacterial applications, self-cleaning surfaces, water splitting for hydrogen production, environmental sensors |
34 |
3. Structural properties of Bi2WO6 and its isotypic materials
Both Bi2WO6 and Bi2MoO6 compounds are known to exist in three polymorphic phases that are temperature-dependent. At room temperature, both compounds adopt an orthorhombic structure, known as the L phase with space group P21ab, and exhibit ferroelectricity. The structure consists of alternating [Bi2O2]2+ fluorine layers and [MO4]2− layers, which contain octahedral units, as depicted in Fig. 1a. Bi2WO6 exhibits a medium-temperature orthorhombic form (phase I) that has a higher symmetry with space group B2cb, but the connectivity is similar to the low-temperature orthorhombic phase (L). The high-temperature monoclinic form (phase H) of Bi2WO6 (Fig. 1b) still retains its layered nature, but the [MO4]2− moieties now contain octahedral-bonded side chains, as reported in studies by ref. 35.
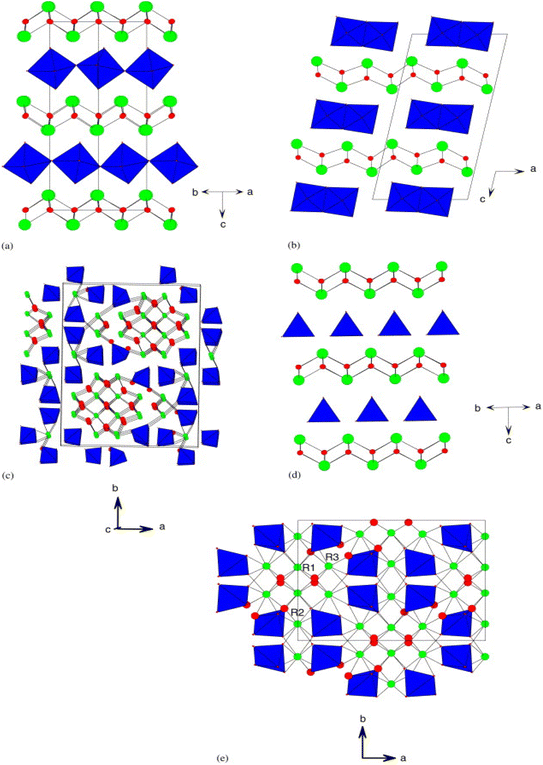 |
| Fig. 1 (a) The structure of the L-Bi2WO6 phase, space group P21ab; (b) the structure of the H-Bi2WO6 phase, space group A2/m; (c) the structure of γ(H)-Bi2MoO6, space group P21/c; (d) the structure of La2MoO6, space group I41/a; and (e) the structure α-R2MoO6, space group C2/c (reproduced with permission from ref. 35 with permission from Wiley-VCH, Copyright 2006). | |
In the case of Bi2MoO6, the three polymorphisms are generally referred to as γ, γ′′ and γ′ or γ(L), γ(I) and γ(H) for the low, medium and high temperature phase respectively.36,37 In the case of Bi2WO6, the (L) and (I) phases are closely related to orthorhombic Aurivillius-like structures, but the monoclinic γ (H) phase at high temperature is very complex. Fig. 1c depicts this structure, which has been described as “columnar” and contains isolated [MO4]2− tetrahedral units.38,39 Bismuth tungstate Bi2WO6 has three structural transitions: the first occurs at 660 °C with a space group change from P21ab to B2cb; the second paraelectric–ferroelectric transition occurs at about 930 °C and finally an orthorhombic–monoclinic phase transition at 960 °C with the space group changing to A2/m.35,40,41
The Bi2−xTRxMO6 solid solution series (with M = W or Mo and RE = rare earth) exhibits several structure types at the opposite end of the series (x = 2), depending on the nature of elements RE and M. For instance, La2MoO6 compound has a quadratic structure that is closely related to Aurivillius phases. It comprises fluorine-like layers [La2O2]2+ and tetrahedral layers [MoO4]2− as illustrated in Fig. 1d.42 On the other hand, Pr2MoO6 and Nd2MoO6 adopt a monoclinic (H) γ-TR2MoO6 structure, while Ce2MoO6 adopts a pseudoclinic (β-polymorph) structure, whose details remain unknown. In the case of TR lanthanides with low ionic radii, the structure observed is of the α-TR2MoO6 type (C2/c monoclinic), which exhibits a complex superstructure related to fluorine layers containing isolated [MoO]5 polyhedra, as shown in Fig. 1e.43,44 Tyulin and Efremov45 reported seven polymorphic forms, including quadratic I (observed in the case of lanthanum) and monoclinic II (in the cases of TR = Ce–Ho, Y), which are identical to α-TR2MoO6. The remaining forms (III–VII) have completely different structures.
Numerous investigations have been carried out on Bi2−xTRxMO6 solid solutions. In the case of M = W, Watanabe discovered monoclinic phases of P2/c symmetry for all lanthanides over a range of compositions from 0.3 to 1.3.46 Meanwhile,47 recently demonstrated that this phase corresponds to H-Bi2WO6 (A2/m) and conducted a detailed structural analysis of Bi0.7Yb1.3WO6 using neutron diffraction.48 As for molybdates, the Bi2−xTRxMoO6 solid solution also exhibits an H-Bi2WO6-like phase,48 as seen in the case of the compound Bi2−xNdxMoO6 in the compositional range 0.4 < x < 0.7.
According to Taoufyq and coworkers49 The non-centrosymmetric space group Pca21, which had been previously suggested for this phase, was confirmed by both the Rietveld analysis and electron diffraction. Furthermore, they have delivered the first direct imaging of structural layers with the two types of sandwiches of Bi2O22+ (Bi–O–Bi layers) and WO42− (O–W–O layers) chemical entities. Generally, these pure phases can be obtained by solid-state synthesis, using careful selection of sintering temperature and time, cooling rate and even the rate of alkali metal used.47
3.1. Electrical properties
These materials present multiple interesting properties including their piezoelectricity, their ferroelectricity and their non-linear dielectric susceptibility.35,36,50,51
Piezoelectricity is a property possessed by certain crystalline phases that become electrically polarized under the action of a mechanical stress, and conversely deform when an electric field is applied to them. This property is used in acoustic, shock and vibration wave sensors.52 Ferroelectricity refers to a material's ability to exhibit an electrical polarization in its spontaneous state, which can be reversed by an external electric field. Bi2WO6 displays complex conduction properties. At temperatures below 700 °C and oxygen partial pressures of 1 to 10–1 atm, Mączka et al. found that Bi2WO6's conductivity is mostly ionic, but for pressure values below 10–1 atm, it is mostly electronic.53
In a separate study, Salkus et al.54 investigated the electrical impedance properties of Bi2WO6 pellets and discovered that electronic conductivity is highly dependent on oxygen activity (Fig. 2): lowering oxygen activity results in an increase in n-type conduction, while high oxygen activity yields p-type conduction. This behavior is typical of oxygen ionic conductors, in which redox reactions occur as a function of oxygen partial pressure. Both n- and p-type electronic conductivities begin to saturate at the extremes of oxygen activity. Generally, oxygen vacancies associated with negative electronic charges in the conduction band are the majority of defects in the n conduction domain, whereas cationic vacancies associated with positively charged holes in the valence band are the majority of defects in the p conduction domain.
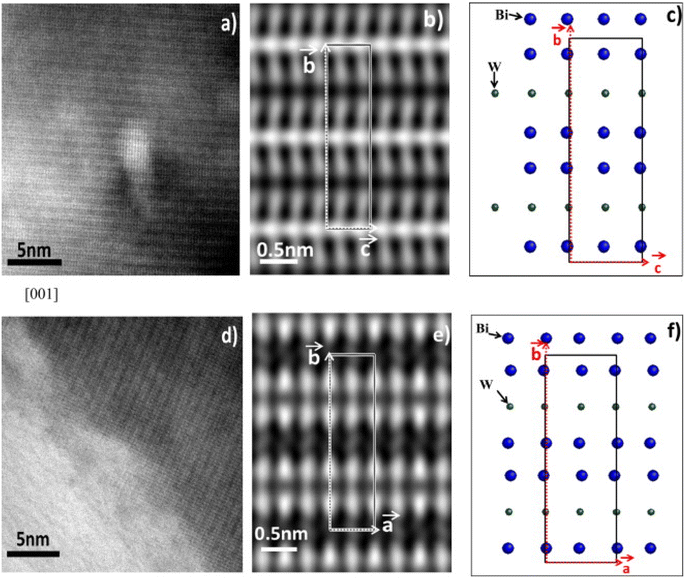 |
| Fig. 2 High resolution TEM images of Bi2WO6 (reproduced from ref. 49 with permission from Elsevier, Copyright 2013). | |
The impedance spectra of Bi2WO6 were found to exhibit two distinct regions of dispersion, as noted by the authors. To simulate these spectra, they used an equivalent circuit consisting of two circuits. At high frequencies, the first circuit consisted of a resistor and a constant-phase element in parallel (Rb//CPEb), which described the movement of charge carriers within the ceramic bulk. The second circuit was composed of a resistor and a constant-phase element in parallel (Rgb-CPEgb), which described the movement of charge carriers through the grain boundaries of the ceramic (Fig. 3b). The authors also observed an ion blocking effect at the solid–electrode interface at 800 K. The conductivities σb and σgb were then calculated (Fig. 3b), and their activation energies were found to be 0.92 and 1.44 eV, respectively.
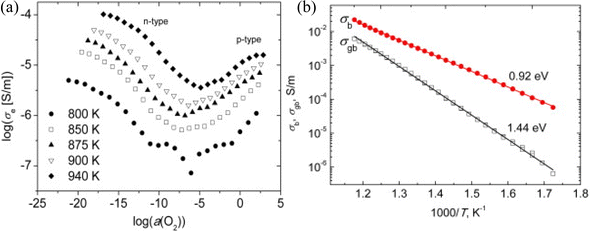 |
| Fig. 3 (a) The variation of electronic conductivities as a function of O2 activity at different temperatures in BWO, (b) variation of σb and σgb conductivities as a function of BWO temperature (reproduced from ref. 54 with permission from Elsevier, Copyright 2015). | |
Toufyq et al.,49 have investigated the electrical properties under air and argon. Authors have used electrical impedance spectrometry (EIS) and direct current (DC) analyses were used to determine the electrical properties of Bi2WO6 compacted pellets systems between 350 and 700 °C, under air and argon conditions. The DC analyses revealed that the conduction observed from EIS analyses was primarily ionic in nature within this temperature range, with a minor electronic contribution. Electrical changes were observed above the transition temperature of 660 °C, under both air and argon atmospheres (Fig. 4). The significant increase in conductivity observed under argon is explained by the formation of additional oxygen vacancies, coupled with electron conduction.
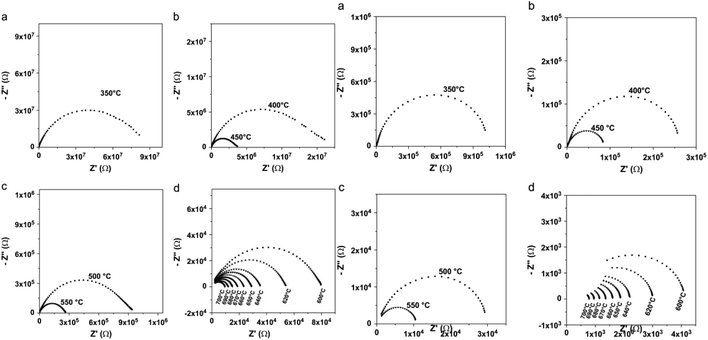 |
| Fig. 4 (Left) Typical Nyquist representations for compacted pellet of Bi2WO6, as a function of temperature, under air; (a) T = 350 °C, (b) 400 °C ≤ T ≤ 450 °C, (c) 500 °C ≤ T ≤ 550 °C, (d) 600 °C ≤ T ≤ 700 °C. (Right) Typical Nyquist representations for compacted pellet of Bi2WO6, as a function of temperature, under argon flow; (a) T = 350 °C, (b) 400 °C ≤ T ≤ 450 °C, (c) 500 °C ≤ T ≤ 550 °C, (d) 600 °C ≤ T ≤ 700 °C. (reproduced from ref. 49 with permission from Elsevier, Copyright 2013). | |
3.2. Photoluminescence properties
Luminescent materials are used for the detection of low or high energy radiation, UV, X-rays or γ-rays, by converting them into UV, visible, or infrared emission, with wavelengths corresponding to the sensitivity domains of the different photodetectors (photomultiplier, photodiode, photographic film, CCD camera, amorphous silicon…).55
The process of luminescence is extremely complex. We can describe the phenomenon of luminescence in three main steps: the absorption of a photon of suitable energy, then the creation of electron–holes and finally the recombination of the pairs with emission of photons (Fig. 4). In the case of a semiconductor the excitation of the electrons of the valence band is obtained from photons of energy higher than the gap in general.
In the case of doping, the electrons and holes thus created relax and can transfer their energy towards the luminescent center which can be a dopant, a defect or a molecular grouping and finally the last stage is the luminescence of the activator center.
3.3. Luminescent properties of pure and substituted BWO phase
In recent studies, the luminescent properties of scheelite and wolframite tungstates have been explained in two ways. Firstly, it has been suggested that the luminescence arises from electronic charge transfer occurring within complex (WO4)2− oxyanions or from luminescent defects in WO3.56 Secondly, Van Oosterhout et al.57 used a molecular orbital model to demonstrate that the excited state corresponds to an electronic transition from the 2p oxygen orbital (O 2p) to the 5d tungsten orbital (W 5d) with t2g symmetry. According to this model, the emission spectra involve transitions from the double 3T1u levels (triplet states) to the inner 1Ag1 level (singlet state). Other researchers58–61 have put forth similar interpretations. Some of them have described the fundamental emission in structures resembling scheelite as transitions from two triplet states (3T1 and 3T2) to the ground state 1A1, involving charge transfer in oxyanions (WO4)2−. Similarly, in wolframite structures, they suggest transitions from two triplet states (3T1u and 3T2u) to the ground state 1A1g, again involving charge transfer in oxyanions (WO6)6−. Additionally, in the case of octahedral (WO6)6− oxyanions, two extra transitions with lower energies have been taken into account. The emission and absorbance for both structures are depicted in Fig. 5, with the blue and red arrows indicating the respective processes. The dotted arrows represent forbidden transitions. To recap, the ground state 1A1 can be visualized as the configuration where the oxygen's O 2p orbitals are fully occupied, while the tungsten's W 5d antibonding orbitals remain empty, resulting in a total spin of S = 0. The spectroscopic terms 3T or 1T correspond to the excited state, where an electron from oxygen moves to one of the W 5d levels of tungsten, leaving an unpaired electron on oxygen. Depending on the spin number, two distinct excited states with different energies and characteristics can be identified: the unstable singlet state (1T: total spin S = 0) and the triplet state (3T: total spin S = 3). In the case of compounds containing bismuth, photoluminescence signals under UV excitation have been attributed to internal bismuth transitions.62 The authors have employed theoretical calculations to explain emissions occurring within the range of 300 to 600 nm in the UV-visible spectrum, as well as emissions in the near infrared (NIR) region, specifically between 800 nm and 1500 nm or beyond. PIR emissions have frequently been observed in various doped bismuth materials, including glasses.63 These NIR emissions rely on the excitation energies and the characteristics of the matrix material itself. The origins of these emissions are still a subject of extensive discussion in the literature. It is hypothesized that these emissions could be produced by bismuth species such as Bi2+, Bi+, or by more complex bismuth-based molecules functioning as luminescent defects.
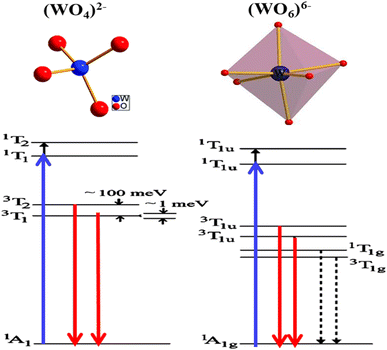 |
| Fig. 5 The energy levels of the photoluminescence process in scheelite (WO4)2− and wolframite (WO6)6− structures. This figure was modified for understanding purpose and (reproduced from ref. 58 with permission from AIP Publishing, Copyright 2005). | |
Few studies have been performed on the luminescence of substituted bismuth tungstates under UV or high energy radiation (X-rays, γ-rays). Recently, some studies have been developed to amplify the emission in the red by doping the BWO phase with Eu3+ europium ions (Fig. 6): the energy level of these ions is placed in the band gap, which favors the charge transfers between the energy levels of the BWO bismuth tungstate and the europium levels (4f–4f or 4f–5d level).64,65
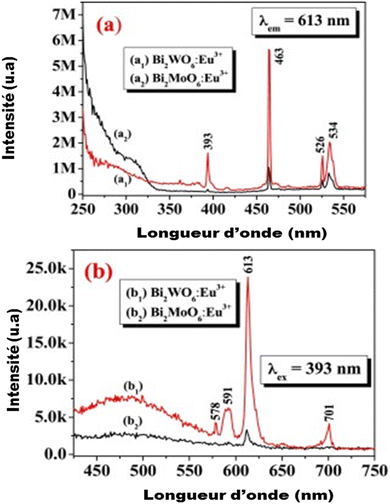 |
| Fig. 6 The emission of bismuth tungstate doped by europium according to the authors (reproduced from ref. 64 with permission from ASP, Copyright 2010). | |
The luminescent properties of Bi2WO6 are primarily attributed to the radiative decay of the anionic complex [WO4]2. This has been supported by studies on MWO4 compounds (where M = Pb, Ca and Zr) with a quadratic scheelite structure, which have shown that the nature of the M2+ cation does not affect the luminescence spectrum, which is solely determined by the [WO4]2 complex.66 In orthorhombic bismuth tungstate Bi2WO6, the luminescence is also determined by the [WO4]2 complex.67 Bordun et al.68 have confirmed these findings in their investigations on BWO and PbWO4 thin films, which showed that the luminescence bands were related to the [WO4]2− groups, even with substitutions or changes in crystal field symmetry. They also observed a strong electron–photon interaction, indicating the local character of the electronic excitations undergoing radiative decomposition. Based on research by ref. 48 and 66, the main luminescent bands of PbWO4 and Bi2WO6 are due to self-localized Frenkel excitons, which describe the excited state of the [WO4]2 complex, with respective maxima at 2.8 eV and 2.93 eV.
To conclude, in bismuth tungstate BWO, luminescent bands are observed due to electronic transitions or charge transfers within the [WO4]2 complex. Previous works by ref. 69 and 70 interpret this emission as self-localized molecular excitons. Molecular orbital calculations for the [WO4]2− complex of Td symmetry reveal that the ground state 1A1 has a t61 electronic configuration, and the excited states are 1T2 > 1T1 > 3T2 > 3T1, in decreasing order of energy. The allowed transitions from 1A1 to 1T2 and 1T1 are considered the fundamental absorption limit, while the lower triplets 3T2 and 3T1 are responsible for radiative transitions. This finding is supported by previous studies by ref. 69, 71 and 72.
The spectral position and structure of the luminescent band are significantly affected by the coordination environment of the emitting center.72 Monoclinic structures exhibit significant differences in luminescence properties compared to scheelite tungstates due to changes in tungsten environment and crystallographic data, particularly W–O distances.72 Von Oosterhout's previous calculations,72 based on a molecular orbital model for the [WO6]6− octahedron, indicated that the highest state occupied by the T1g symmetry is associated with the 2p orbital of oxygen and the excited state consists of an electron occupying the 5d orbital of tungsten with t2g symmetry. As per the allowed transitions 1Ag1 ⇒ 1T1u, it is established that the absorption by the [WO6]6− complex is attributed to these transitions (Fig. 5). Therefore, the most likely scenario for emission involves transitions from two 3T1u levels to the fundamental 1Ag1 energy level. Ait ahsaine et al.,73 have studied the photoluminescence properties of different substituted BWO materials with lutetium (Fig. 7). Authors have found that the formation of the monoclinic structure induced by the substitution of bismuth with lutetium is closely linked to the photoluminescence observed under monochromatic UV excitation. The resulting emission can be classified into two types. The first is the conventional emission of WO66− tungstate groups, which has two components attributed to charge transfers “W 5d O 2p” when octahedral coordination occurs. The second is a specific emission observed in the near-infrared region at 1.25 eV and is strongly correlated with the presence of lutetium. However, the exact origin of this emission remains uncertain. According to previous research on tungstates, it may result from defect centers originating from oxygen vacancies or defects in bismuth species with different valences or molecular clustering.
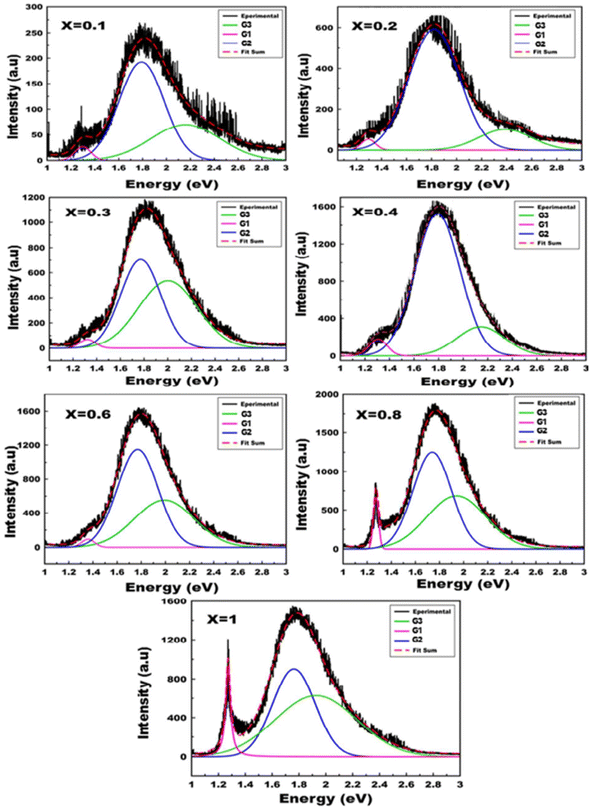 |
| Fig. 7 Photoluminescence spectra of the Bi2−xLuxWO6 materials (reproduced from ref. 73 with permission from Royal Society of Chemistry, Copyright 2015). | |
4. Photocatalytic properties
Photocatalysis involves using UV or visible light to activate a semiconductor material, which then promotes redox reactions that degrade organic molecules in an aqueous medium.74–77 The semiconductor's electronic structure consists of a valence band and a conduction band separated by a band gap. Upon irradiation with photons having energy greater than or equal to the band gap, electrons move from the valence band to the conduction band, generating electronic holes. The electron–hole pairs created in the photocatalyst may recombine or, if their lifetime is long enough, migrate to the surface of the photocatalyst and contribute to the photocatalytic reaction. Surface charge carriers can also be trapped before recombination or release. Recombination is the main limiting factor in photocatalysis because it prevents the use of surface charge carriers for catalytic oxidation reactions.76,78
4.1. The relationship between structure and photo-catalysis capacity of BWO photocatalyst
Bi2WO6, as one of the most common Aurivillius oxides, possesses an orthorhombic structure composed of [Bi2O2]2+ layers connected to corner-shared [WO4]2− layers. The arrangement and bonding within these layers affects the characteristics and performance of the Bi2WO6 as a photocatalyst.79,80 The layered structure of BWO offers a wide surface area, allowing the provision of active sites for adsorption and reaction with target species. In addition, the unique sandwich architecture of BWO enables the induction of a built-in electric field between the layers, favoring the separation of the photo-generated holes and electrons.21 In this context, Density Functional Theory (DFT) calculations may be used to examine the contribution of orbitals to the CB and VB. It is well known that the VB of BWO is formed by the hybrid orbitals of O 2p and Bi 6s, the CB of BWO is composed of W 5d orbitals.81 On that basis, the band gap of BWO is usually between 2.6 and 2.9 eV, according to the position of VB and CB, making it a visible light sensitive photo-catalyst.21 In the other hand, defects, like oxygen vacancies are commonly present in BWO photo-catalyst and can act as active sites for catalytic reactions. Recently, Liu et al.82 demonstrated that with a high concentration of oxygen vacancies in Bi2WO6 surface, a photo-degradation of 100% of levofloxacin was achieved, they revealed that oxygen vacancies can not only speed up the separation of photo-generated charges, but can also activate O2 molecules to form superoxide radical species.82 In a related study, Yang and his team83 demonstrated that the marked enhancement of photocatalytic performance in the decomposition of phenol and dyes may be ascribed to the synergistic impact of the oxygen deficiency-induced band shifts.83 In addition to oxygen vacancies, the crystallinity and exposed crystal facets of BWO can have a major impact on its photocatalytic performance.21 Various crystal facets may have different reactivity and surface characteristics, influencing the adsorption and reaction kinetics of desired molecules.84 Facets with greater surface energy generally exhibit more exposed reactive sites, promoting the adsorption of molecules and favoring surface reactions. This enhanced surface reactivity may enhance the overall photocatalytic performance of BWO.85 To summarize, the structure of BWO, comprising its layer morphology, surface defects, crystal facets and band structure collectively affect its photocatalytic capacity. These structural properties define the performance of the material in light uptake, charge separation and redox reactions, thus ultimately impacting its capability as a photocatalyst. Nevertheless, as a photocatalyst, BWO has the same problems: the recombination rate of photo-induced holes and electrons of BWO is still high, the BWO can only adsorb visible light having wavelength shorter than 450 nm because of the band gap restriction.15,86 Hence, optimization of the aforementioned properties of BWO photocatalyst is the main key to improve its photocatalytic performance.
4.2. Photocatalytic properties of pure and substituted BWO
For some years, bismuth tungstate has been considered as a potential solar energy converter,87 which can activate the degradation of organic matter88 through surface redox mechanisms. Despite recent studies, the reaction mechanisms remain complex and poorly understood. In general, several researchers agree that photocatalysis corresponds largely to an oxidation phenomenon governed by the holes (h+) of the valence band, and likely to be influenced by the presence of certain ions (SO42−, HCO3−, NO3−, Na+, K+, Ca2+, Mg2+, Cl−, Cu2+, Fe3+) at variable concentration in aqueous medium.89 In recent times, CdWO4, PbWO4, ZnWO4, and Ag2WO4 have been utilized for photocatalysis because of their favorable electronic band structures that promote chemical reactivity and facilitate the production of free radicals in aqueous suspensions.90–94 Nevertheless, the practical application of these materials in the visible range is hindered by their high gap energy.
The valence band of Bi2WO6 consists of a hybridization of O 2p and Bi 6p orbitals with a minor contribution from Bi 6s orbitals, while the conduction band is primarily composed of 5d tungsten orbitals with a slight contribution from Bi 6p orbitals, as revealed by theoretical studies on its electronic band structure (Fig. 8).6,95 This results in a widely dispersed valence band that enhances the mobility of photon-excited holes and facilitates oxidation reactions. Bi2WO6 has demonstrated great potential in selective organic synthesis and bacterial inactivation in aqueous solutions under visible light, such as benzyl alcohol oxidation to aldehydes and glycerol oxidation to dihydroxyacetone, according to previous.7,96 Various hierarchical morphologies of Bi2WO6 have been synthesized using classical methods, including sol–gel, hydrothermal/solvothermal, combined sol–gel/hydrothermal, and sonochemical methods, producing nanosheets, globules, bowl shapes, peony flowers, microspheres, nanolaminars, hollow structures, microdisks, and multilayer disks.97–99 These morphologies possess large specific surface areas, facilitating the adsorption of organic molecules and promoting photodegradation. Despite its versatility, Bi2WO6 has limitations such as low light absorption capacity, limited response to visible light, and high degree of recombination of charge carriers that hinder its use in photocatalysis. Possible modifications to overcome these limitations are discussed below.
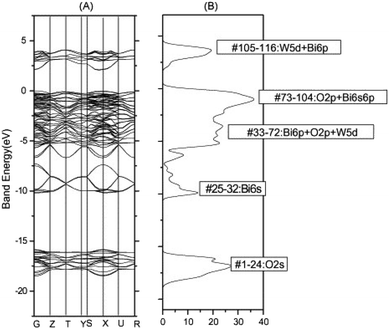 |
| Fig. 8 Bi2WO6: (A) band structure, (B) density of states calculated by the DFT method (reproduced from ref. 95 with permission from Elsevier, Copyright 2006). | |
The separation of charge carriers could be improved by modifying the surface of Bi2WO6 with copper ions (Fig. 9)100 and by using the synthesis of {0 1 0} reactive facets.101 This separation of electron–hole pairs, essential for reduction and oxidation reactions, allows the generation of reactive oxygen species. The crystalline facets provide larger surfaces for active sites for photocatalysis.
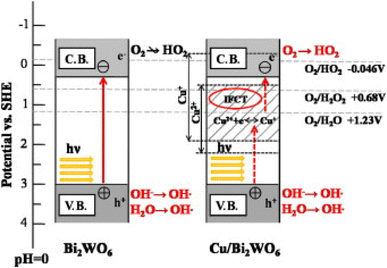 |
| Fig. 9 The photocatalytic mechanism of pure BWO and BWO doped with copper (reproduced from ref. 100 with permission from Elsevier, Copyright 2014). | |
4.2.1. Metals coupled-Bi2WO6. Although doping with noble metals such as silver can prevent recombination of conduction band electrons,102,103 the Ag–Bi2WO6 material exhibits photoactivity even at temperatures between 50–70 °C due to the thermal effects induced by surface plasmon resonance. This suggests that the composite can function as a photocatalyst at higher-than-room temperatures. The combination of the Ag–Bi2WO6 composite with mesoporous carbon or graphene has also resulted in excellent radiation adsorption capacity and improved photoactivity.104,105 In addition, silver nanoparticles are highly effective in scattering and light absorbing properties, and their hybrid effect enhances the spectral range. Moreover, the high conductivity of the carbonaceous structures enables better separation of electron–hole pairs, improving surface reactivities and delaying their recombination. In this context, Chang and his team106 succeeded in fabricating the Ag–BWO hybrid via an electrochemical method. They demonstrated that the contact between BWO, the Ag nanoparticles (NPs) and the substrate helped to effectively separate the photo-excited charges resulting in enhanced photocatalytic activities. In addition, they confirmed that decorated Ag NPs extend the absorption range of UV light to the visible light wavelength region.106 Recently, a novel Ag-doped BWO/BiOI photocatalyst has been produced using a two-step hydrothermal process. It was observed that the quantity of Ag in BWO/BiOI obviously influenced the photoelectric characteristics and photocatalytic performance.107 On the other hand, Yang et al.108 attempted to combine Ag with AgCl and BWO to form a ternary system capable of degrading toluene. They demonstrated that the plasmonic effect of silver and high redox performance of the Z-heterojunction significantly enhanced the separation of photo-induced electron/hole pairs and the transfer efficacy.108 Shen et al.109 have successfully engineered a peony shaped hierarchical 3D Ag/BWO photocatalyst via a hydrothermal approach. The possible mechanism discussed in their study suggests that the improved photocatalytic activity derives from the unique morphology of the material, which enables the adsorption of organic substances, and also from the raised Schottky barriers separating the interface of the Ag NPs and BWO, which favor the transfer of photo-induced electrons from BWO to the Ag NPs.109 The Au–Bi2WO6 composite was observed to exhibit photocatalytic activity only when exposed to photons within the 400–800 nm wavelength range, while it remained unresponsive under photon excitation within the 500–800 nm range, despite the existence of surface plasmon resonance of gold nanoparticles in this range. This suggests that there is no direct correlation between the plasmon absorption of gold nanoparticles and the photocatalytic efficiency, as reported by.110 Furthermore, in another study the excellent photocatalytic activities of Au–BWO composite were attributed to the increased light harvesting efficacy caused by the addition of gold and the high separation of photo-generated electron–hole pairs.111 Li et al.112 indicated that the surface plasmon resonance effect facilitated photo-generated electron transfer between BWO and Au, leading to accelerated charge transmission and thus inhibiting electron/hole recombination, conferring high photocatalytic properties to the photocatalyst.112 Similarly, wan et al.113 reported that the inclusion of gold in the BWO@MoS2 structure allowed the creation of additional charges, which were subsequently passed on to the MoS2 nano-sheets through S–O conferring high photocatalytic properties to the hybrid. In addition, superior photocatalytic yields have also been ascribed to the effective steering of the charge kinetics implied in this system, notably the HEI (hot electron injection) effect with its extended spectral response range to light for enhanced charge generation.113 Recently Phuruangrat et al.114 have noted that gold with oxidation degree 3+ was successfully embedded in the BWO lattice, in this investigation they reported that BWO doped with 3% Au has the weakest emission intensity and excellent absorption properties in the UV-visible range with an absorption peak of 437 nm.114 In another research, Hu et al.115 exploited the optical characteristics of gold to improve the infrared light absorption of BWO. This upgrade was credited to SPR effects and large-scale collection of infrared light using Au nanorods.115
4.2.2. Metals ions doped-Bi2WO6. Chemical substitution (or doping) influences the arrangement of atoms within the structure, modifies the chemical bonds and thus the band structure of substituted compounds of the type Bi2−x MxWO6 where M is a trivalent ion (Lu3+). This substitution also plays a role in the crystal growth during synthesis, and can therefore induce various morphological modifications110 that can be useful for photocatalytic reactions.The addition of Zr4+ metal ions to the Bi2WO6 matrix, instead of W6+, can induce an extrinsic oxygen gap by charge compensation, which results in a significant red shift in the band gap absorption.116 These oxygen gaps can easily trap excited electrons and prevent charge carrier recombination. Furthermore, these oxygen vacancies can enhance oxygen adsorption on the semiconductor surface, which facilitates the reduction of dioxygen to form superoxide radicals.117 According to118 doping with Europium was the primary reason for the high activity of the photocatalyst, rather than changes in band gap absorption properties. Recently, Tahir and his team119 reported on the in situ hydrothermal engineering of a Mn-doped Bi2WO6–GO/MoS2 photocatalyst. They found that the Mn-doped composite had an absorption edge located in the visible zone with a gap energy of 2.2 eV. Additionally, the Mn-doped BMG displayed an even larger light absorption region in the entire visible domain when compared to the virgin ternary sample. In the same year, Su et al.120 succeeded in making Ni2+/Bi2WO6 with a flower shape. The characterization findings showed that Ni2+ was able to penetrate into Bi2WO6 and partly supplant Bi3+. Furthermore, the improvement of photocatalytic properties is attributed to the creation of defects and the reduction of the gap in the Ni2+–Bi2WO6. Photocatalytic findings revealed that the highest photocatalytic breakdown rate of RhB, up to 93%, was attained when using 0.25% Ni2+–Bi2WO6. In another similar work by ref. 121, have shown that Bi5+ doping allows to narrow the bandgap of Bi2WO6 nano-flowers, and enhances the absorption capability of Bi2WO6 nano-flowers in the visible-light rang significantly. A Bi2WO6 doped Bi–I photo-catalyst was successfully produced through a solvothermal process. The superior photoactivity of the I-doped Bi2WO6 photocatalyst was mainly attributed to two factors: the introduction of impurity levels by the I dopant, which enhanced visible light absorption, and the efficient migration and separation of charge carriers. Additionally, the surface plasmon resonance (SPR) effect of the Bi metal also contributed significantly to the improvement in photocatalytic activity, as reported by ref. 121.
A comparative study was carried out by Zhu et al.122 in which they compared the photocatalytic performances of Bi2WO6 doped with various metals ions such as (Cu2+, Zn2+, Mg2+ and Fe3+). They have found that Bi2WO6 doped with metal ions greatly enhances the photodecomposition of ciprofloxacin (CIP) and norfloxacin (NOR) antibiotics, this improvement was explained by the increase of the specific surface area after doping and the reduction of the gap energies (novel impurity levels entered between the CB and the VB of Bi2WO6). Furthermore, they found that Mg2+ doped as an electron reservoir allowed the Mg/Bi2WO6 composite to have the highest degradation rate of 99.11% for CIP and 89.44% for NOR. On the other hand, iron doping displayed a plasmonic resonance effect and consequently a strong absorption of visible light was noted.122 In a related study, Lovisa et al.,123 reported the manufacture of zinc-doped Bi2WO6. In this study they demonstrated that increasing the Zn2+ concentration promoted the inhibition of photocatalytic characteristics through the occurrence of oxygen vacancies that functioned as a charge recombination center. On the other hand, the photoluminescence was further enhanced by the Zn2+ doping, moreover the color adjustment was favored according to the increase in zinc content noticing the transition from: yellow to orange to green. Bera and his co-authors,124 proved that in situ doping of the Bi2WO6 microstructure with a metal (Fe, Zn, Mo) has improved the absorption and charge transfer efficacy. It was revealed that Mo doping displayed the strongest photocurrent density, which was 57 fold better compared to that of naked Bi2WO6. In addition, the smaller ionization potential for Mo–Bi2WO6 indicated an enhancement in electron mobility and charge separation. On the other hand, Koteski et al.125 have exploited density functional theory to investigate substitutional doped Bi2W1−x MxO6 with (M = Mo, Fe, Cr, Zn; x = 0.125, 0.50, 0.25). They found that for all transition metal dopants examined, the optical characteristics in the visible spectrum were superior to those of non-doped Bi2WO6 (Fig. 10a). This improvement was explained by the localized defective states in the bandgap upon insertion of Fe, Mo and Cr which leads to the narrowing of the bandgap. In addition, as shown in Fig. 10b Zinc doping exhibited more enhanced optical properties compared to other dopants as it eased the narrowing of the band gap through inducing a shift of the states close to the peak of the valence band to higher energies. In another study a series of Cu2+/Ni2+ doped Bi2WO6 was manufactured with success via a simple solvothermal procedure and it displayed the highest photocatalytic ability with low charge transfer resistance and considerable photocurrent.126 Zhong et al.127 demonstrated that after the insertion of copper into the Bi2WO6 structure the absorption edge was dramatically enhanced and moved to the red in the visible zone, which was ascribed to the charge transfer between the Cu species and the host matrix. Moreover, the inclusion of copper ions in the BWO structure has introduced some new energy levels of the copper ions within the band gap of BWO, leading to a diminished band gap. In a related study, iron doping exhibited a lower emission intensity in comparison to the non-doped Bi2WO6, which confirmed that the iron effectively suppressed the charge recombination. Additionally, Fe-BWO gave a greater photocurrent response and a reduced charge transfer resistance. It was observed that the increase in iron concentration led to an increase in PL intensity, this was accounted for by the fact that the surplus oxygen vacancies might function as hole–electron recombination centers128 Arif et al.129 indicated that titanium (Ti) doping has a significant impact on the band structure and electron dynamics, and also was able to insert redox couples (Ti3+/Ti4+), leading to a meaningful increase in reactive oxygen entities during the photocatalytic process, in addition to the Ti doping may also produce crystal defects in the crystal lattice of BWO, leading to highly improved visible-light driven photocatalytic activity. Optical results showed that Ti doping exhibited an apparent blue shifting (about 18 nm), which might be attributed to the substitution of Bi3+ by the Ti3+ cation and the nano-scale (NS) effect, in addition to which a weaker PL emission intensity was found for the Ti-doped Bi2WO6 pointing to a low rate of recombination of the photo-induced hole–electron pairs. In addition, the presence of Ti cations upon the surface of BWO might function as an oxygen scavenging site, thus benefiting redox pair generation and the interfacial charge transfer, thereby resulting in improved photocatalytic efficiency.129 In order to achieve improved photocatalytic properties of Bi2WO6, the same team controlled the Ti dopant level to alter the crystal defects and oxygen vacancies in the Bi2WO6 crystal lattice. It was shown that the crystal defects mediated via Ti doping and generated oxygen vacancies were critically significant for controlling the photocatalytic behavior of Bi2WO6. Remarkably, the Ti–Bi2WO6 hybrid displays deeply enhanced photocatalytic activity for the decomposition of ceftriaxone sodium and an especially enhanced photocatalytic H2 yield, with a 5.8 times higher rate than Bi2WO6.130 Mahhumane and co-authors,131 documented that iron doping gave super-hydrophilicity to Bi2WO6 and resulted in better charge separation. As shown in Fig. 10c, electrochemical impedance spectroscopy experiments showed that switching the EG-Fe-doped BWO electrode to a slower frequency confirms a more rapid electron transport process. Furthermore, the influence of Fe-doping was visible through the increased absorbance in comparison to the electrode not doped by Fe (Fig. 10d). This indicated that Fe doping enhances the photoresponse of BWO. It was noted that the gap energy of Bi2WO6 and iron doped Bi2WO6 was 2.7 eV and 1.6 eV respectively, this reduction was due to the creation of an iron doping level in the band gap of BWO, which helped to separate holes and electrons.131 New Co-doped Bi2WO6 nano-sheets onto the surfaces of a Fe3O4 composite material were successfully produced using a facile hydrothermal approach, it was suggested that the enhancement of photocatalytic properties was attributed to the effect of Co2+ doping as well as the synergistic action between the heterojunction interface, which gave rise to high visible light uptake with a great charge separation efficacy. The PL results indicated that the fabricated composite showed noticeably low emission intensity, suggesting a weaker recombination rate of photo-induced electrons/holes. Furthermore, gap energy calculations indicated that the doped Bi2WO6 exhibited a gap energy of 2.75 eV which was 0.06 eV shorter compared to that of pristine Bi2WO6.132
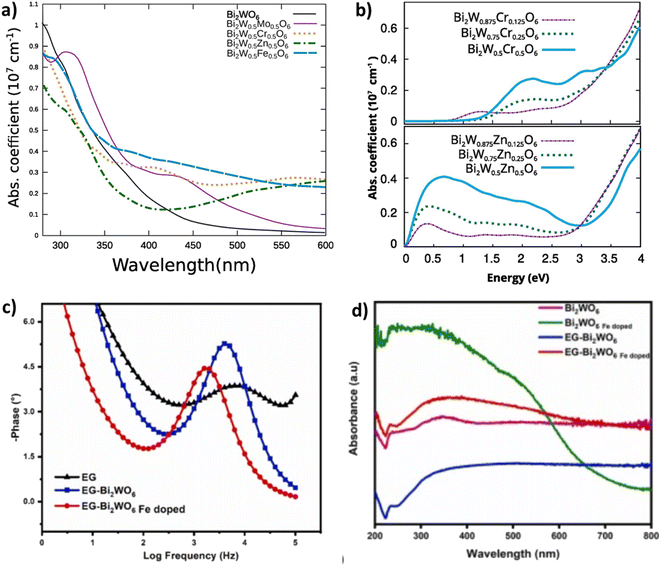 |
| Fig. 10 (a and b) DRS analysis and absorbance coefficients of doped and non-doped BWO (reproduced from ref. 125 with permission from Elsevier, copyright 2020). (c) EIS experiments of EG, EG-BWO and EG-Fe doped BWO electrodes. (d) DRS spectra of doped and doped BWO (reproduced from ref. 131 with permission from Elsevier, Copyright 2022). | |
4.2.3. Doping with RE elements. According to research by ref. 133, rare earth ions including Ce3+, Nd3+ are effective dopants for promoting photocatalytic activity, while Pr ions show poor efficiency. Rare earth-modified Bi2WO6 composites (RE/Bi2WO6) also show great potential as Bi2WO6 dopants. The rare earth series, consisting of 17 rare earth elements including Sc, Y, and lanthanides (La to Lu), are known for their unique electronic and optical properties and have been extensively studied for their photocatalytic performance due to their rich energy level structures and strong multi-band emission.134,135 They can be incorporated into the Bi2WO6 crystal lattice by replacing Bi3+ ions, leading to the formation of RE-Bi2WO6 solid solutions. This incorporation of RE3+ ions can act as electron traps, which can extend the lifetime of photogenerated charge carriers (electrons and holes) and improve their separation efficiency. In addition, RE3+ ions can also change the band structure of the material, leading to a shift in band gap energy and an increase in visible light absorption.136 This in turn can lead to an improvement in the overall photocatalytic performance of the material. Furthermore, as each rare earth element has its own electronic structure and properties, the specific properties of the modified Bi2WO6 will depend on the type of RE3+ ion used; this renewed interest in rare earth has led to many research efforts dedicated to the preparation of new types of Bi2WO6 nanomaterials for photocatalytic applications.137 In particular, different modification strategies have been successfully used to improve the photocatalytic properties of Bi2WO6 nanomaterials.
4.2.3.1 Single rare-earth doping. Different Bi2WO6 composite photocatalysts have been doped with a single rare earth that has shown great promise in enhancing the photocatalytic activity and other properties of Bi2WO6, making it an attractive option for researchers studying photocatalytic reactions. For instance, Tian et al.138 used a hydrothermal process to validate that Gd/Bi2WO6 has higher potential applications in various fields such as photocatalysis, optoelectronics, and energy storage. However, further research is needed to fully understand its properties and optimize its performance for specific applications. Ahsaine et al.,139 Successfully fabricated a composite by a co-precipitation method that showed enhanced photocatalytic activity under UV light irradiation, as the lutetium dopant can reduce the band gap of Bi2WO6, which facilitates the absorption of UV light and can increase the number of electron–hole pairs generated in the material. Indeed, the lutetium dopant can improve the charge separation and stability to reduce the electron–hole recombination rate and enhance the overall photocatalytic efficiency of the material. Similarly, the related research of doped tungstate matrix rare earth is numerous such as Guo and Ren140 elaborated the photocatalyst Tb3+ doped Bi2WO6 by a co-precipitation method and studied their luminescence properties. Gu et al.141 synthesized Eu/Bi2WO6 by a hydrothermal process, which can effectively improve the photostability of Bi2WO6 nanoparticles making them suitable for use in long-term applications.142 prepared 3D hierarchical flower-shaped microspheres of Sm3+-doped Bi2WO6 and found that the 0.5% Sm-BWO sample exhibited the best adsorption capacity among the materials studied due to the synergistic effect of a suitable band gap and high specific surface area. Since Bi2WO6 is considered relatively safe and non-toxic, this makes it, along with Sm3+, a potentially attractive material for biomedical applications and could also lead to promising candidates for a wide range of applications, especially in photocatalysis and optoelectronics.133 synthesized and compared the photocatalytic activity of doped Bi2WO6 (Ce3+, Nd3+, Pr3+ or Sm3+) via a facile hydrothermal process. The result of all these samples is that Nd3+ in Bi2WO6 enhanced the photocatalytic activity with 99.8% degradation of RhB dye after 20 minutes of irradiation. In this context143 doped La, Ce, Gd, and Yb via simple hydrothermal method into the catalyst; the results showed that Gd and Yb are the best for improving their catalytic ability. The more 4f electrons in Gd and Yb cause a stronger distortion of the crystal lattice, which affects the position of the valence band maximum and conduction band minimum. This leads to a larger bandgap and a red shift of the absorption edge to the visible light region. In contrast, La/Bi2WO6 and Ce/Bi2WO6 do not have as many 4f electrons, and therefore, their crystal lattice distortion is not as strong, resulting in a smaller bandgap and a weaker red shift to the visible light region (Fig. 11a and b). Compared to the doping amount of 2% Yb/Bi2WO6 presented by ref. 144 shows that 2% Yb significantly enhances the visible light absorption properties of the material and can act as an electron acceptor that facilitates the electron transfer from the conduction band of Bi2WO6 to the Yb ions, leading to better improvements in photocatalytic performance. Photoluminescence analysis (Fig. 11c) showed that the emission capacity of the Yb–BWO hybrid catalysts was considerably decreased relative to that of the original BWO, and the intensity of 2.0%Yb–BWO was the lowest. In addition, compared to BWO, the Yb–BWO compound exhibits a greater photocurrent response (Fig. 11d), which is coherent with lower fluorescence and greater photo-activity. Recently,145 showed that the 1% flower-shaped Pr–Bi2WO6 microspheres prepared by one-step hydrothermal method exhibited the best photocatalytic performance to degrade RhB based on radical scavenging experiments. Besides to Pr,146 employed 16% Er3+–Bi2WO6 heterostructure photocatalysts to break down tetracycline which can be used for longer periods without losing its effectiveness.
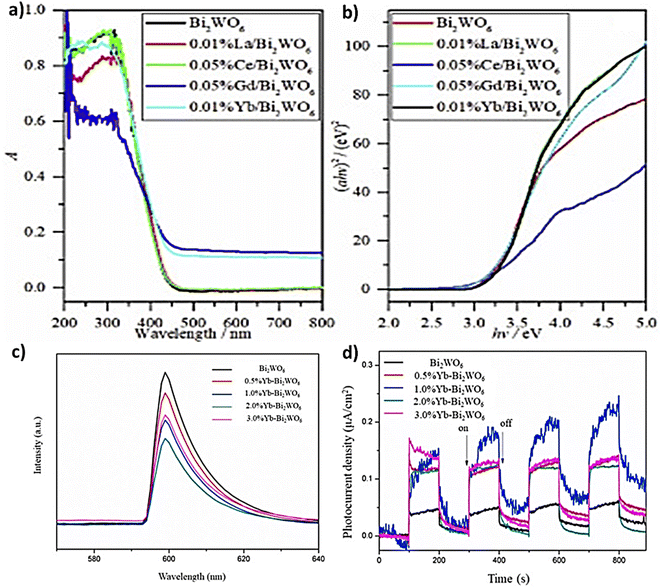 |
| Fig. 11 (a and b) DRS and Tauc's spectra of doped and non-doped BWO (reproduced from ref. 143 with permission from Elsevier, copyright 2021). (c) PL spectra of BWO doped by different Yb concentration. (d) Photocurrent responses of pristine and doped BWO (reproduced from ref. 144 with permission from Elsevier, copyright 2021). | |
4.2.3.2 Co-doping with rare-earths. While doping Bi2WO6 with other elements is not a novel idea, there are few studies on rare earth co-doping with Bi2WO6. In a study by ref. 147, Yb3+/Ho3+-Bi2WO6 was synthesized using a hydrothermal method and a heat treatment process, which demonstrated good upconversion luminescence, exceptional photocatalytic activity, and stability in degrading RhB solution under visible light exposure. Similarly,148 found that Yb3+/Nd3+-Bi2WO6 prepared through the hydrothermal approach offered several benefits over single doping. The Yb3+/Nd3+ addition acted as electron traps, facilitating charge transfer and reducing recombination of photoinduced electron–hole pairs. Moreover, the addition of 1.5% Yb3+ and 0.5% Nd3+ in the Bi2WO6 crystal lattice generated more oxygen vacancies, thereby increasing the yield of O2− and restraining electron–hole pair recombination, ultimately enhancing the photocatalytic performance.149 created Bi2WO6: Er, Yb nanofibers through the solvothermal-electrospinning approach, which displayed great compatibility between crystal and nanofiber. The Yb3+ ions served as sensitizers to absorb photons and transfer energy to Er3+ ions, absorb near-infrared light and transfer energy to Bi2WO6, thus increasing the number of electron–hole pairs and improving photocatalytic activity. Recently,150 produced and employed Bi2WO6 through a hydrothermal process. The literature suggests that co-doping Nd and Pr in Bi2WO6 can enhance photocatalytic activity under visible light irradiation by improving its light responsiveness, enlarging the specific surface area of the photocatalyst, and decreasing the recombination rate of electrons and photogenerated holes, making it useful for degrading contaminants.
4.2.3.3 Triple-doping with rare-earths. Besides, co-doping RE, many research efforts have been devoted to the preparation of a new photocatalysis strategy. Recently, a publication by ref. 151 produced a new Bi2WO6 with triple doping of Yb, Er and Pr via a hydrothermal process, which exhibits superior performance in photocatalytic activity compared to Bi2WO6 doped with a single and co-dopant. The collaborative effect between the three rare earth dopants can act as an electron-trapping center to produce more O2− radicals and promote electron–hole pair separation. In addition, the material exhibits good stability and durability, as well as a wide band gap, making it an attractive option for researchers investigating photocatalytic materials as new candidates for a range of advanced technological applications.
4.2.4. Doping with other elements. In this section, the research data also shows the practical use of other doped elements to achieve their goal. They have successfully used different modification strategies to improve the photocatalytic efficiency of these elements in various applications. The following are some of the commonly used dopants for Bi2WO6. Incorporating nitrogen, boron, and iodine as dopants has been shown to enhance the photocatalytic activity of Bi2WO6. Although boron can improve the catalyst's acidity, it has a limited response to visible light Bi2WO6, as noted by ref. 152 and 153. However, due to its electron-deficient nature, boron can capture electrons to eliminate recombination channels and act as an electron scavenger, promoting the separation of photogenerated electron–hole pairs and thus improving photocatalytic efficiency. Iodine was used as an insertion dopant because of the presence of multivalent species (I0 and I−) in the host matrix. During photocatalysis, I0 acts as an electron acceptor and becomes I−, while hole trapping leads to simultaneous re-oxidation. Additionally, the high concentration of oxygen vacancies and reduction of tungsten from the 6+ to 4+ state can reconfigure the internal local electric field, which promotes charge carrier separation.Recently, self-doping with bismuth ions (Bi4+ or Bi5+) and with cerium–fluorine elements (Ce–F) have been reported in the literature in order to obtain better photoactivity.154,155 The introduction of self-dopants such as Bi3+ or Bi5+ promoted the generation of superoxide radicals and boosted the density of charge carriers. Meanwhile, doping with cerium caused a blue-shift in the absorption spectrum of the synthesized material, while the incorporation of fluorine expanded the spectral response. When multiple species were modified simultaneously, synergistic effects emerged that facilitated the separation of charge carriers and accelerated the degradation of pollutants.
Boron, being a metalloid with properties of both metals and nonmetals, has been successfully doped into Bi2WO6 using a hydrothermal method, as reported in ref. 156. The addition of 0.5% B/Bi2WO6 has shown an increase in mesopores and pore volume, leading to stronger catalytic activity under simulated solar light for degradation. Other studies, such as,152,157 have used a hydrothermal process and a two-step and microwave-assisted hydrothermal method, respectively, to achieve N-doping of Bi2WO6. However, it has been observed that increasing the dopant concentration leads to an increase in the recombination rate, resulting in decreased efficiency. On the other hand,158 found that I-doping into Bi2WO6 nanosheets is effective in the photocatalytic removal of Hg0, as it enhances visible light absorption, generates higher electron–hole pairs, and improves the selectivity of Bi2WO6 for Hg0 removal, making it a promising product for environmental remediation applications. Recently,159 synthesized P-doped and S-doped Bi2WO6 by hydrothermal treatment of Bi2WO6 nanosheets. S-doped Bi2WO6 was found to have a higher ability to adsorb CO2 than P-doped Bi2WO6, as it increases the surface area and the number of active sites available for CO2 adsorption. The presence of S in Bi2WO6 also leads to the formation of oxygen vacancies and stronger chemical bonds between Bi2WO6 and CO2, providing a useful strategy to further optimize the photocatalytic performance of Bi2WO6 for future applications.
5. Conclusion, challenges and perspectives
In conclusion, in this review we have provided a detailed structural study of the Bi2WO6 and its isotypic materials. This material has a promising electrical, and photoluminescent properties which explains its wide use a range of purposes including capacitors, humidity detectors, optical sensors, and thermistors. Bismuth tungstate has shown promising photocatalytic activity for water treatment and dye photocatalysis applications both in its bare and doped configurations. Doping with noble metals such as silver or rare earth elements such as Yb3+, Ho3+, Nd3+, and Pr3+ can further enhance the photocatalytic performance of Bi2WO6 by facilitating charge transfer and reducing the recombination of photoinduced electron–hole pairs. However, the practical application of Bi2WO6 as a photocatalyst for water treatment is still limited by several challenges as follows:
(a) High recombination rates: the recombination rate of photoinduced electron–hole pairs is high, which reduces the efficiency of photocatalytic degradation.
(b) Narrow light absorption range: bismuth tungstates have limited light absorption in the visible region, which limits their use in practical applications.
(c) Low quantum efficiency: bismuth tungstates have a low quantum efficiency, meaning that only a small fraction of the absorbed photons is converted into electron–hole pairs, resulting in low photocatalytic efficiency.
(d) Difficulty in synthesis: the synthesis of bismuth tungstates can be challenging and time-consuming, limiting their large-scale production.
Some of the potential solutions and strategies to overcome these drawbacks are as follow:
Doping bismuth tungstates with metals or non-metals can improve their photocatalytic properties, such as enhancing their visible light absorption and reducing recombination rates.
Introduction of co-catalysts to further improve the photocatalytic activity of bismuth tungstate. Noble metals (such as Pt, Au) or co-catalysts such as cocooning agents, metal oxide nanoparticles, and carbon-based materials have been employed to promote charge transfer and increase the effectiveness of particular reactions.
Engineering of nanostructures by increasing surface area to improve light harvesting efficiency was also reported.
Facets engineering to obtain highly photoactive facets for photoconversion applications.
New experimental and computational methodologies have improved our comprehension of the photocatalytic processes of bismuth tungstate. To maximize the effectiveness and performance of bismuth's photocatalytic properties, scientists are examining the particular functions of defect engineering, surface states, and band locations.
Optimization of synthesis conditions such as temperature, time, and reactant concentration can improve the photocatalytic performance of bismuth tungstates.
However, there is a need for further studies on the underlying mechanisms of photocatalytic activity using operando investigation, the development of more efficient and stable bismuth tungstate-based photocatalysts, and the exploration of new applications in fields such as water treatment and energy conversion.
Conflicts of interest
The authors declare that they have no competing interests.
References
- G. Zhao, S. Hao, Y. Xing, Y. Wang, Y. Wang, K. Xu and X. Xu, Phys. Status Solidi A, 2019, 216, 1900035 CrossRef.
- K. Zhang, J. Wang, W. Jiang, W. Yao, H. Yang and Y. Zhu, Appl. Catal., B, 2018, 232, 175–181 CrossRef CAS.
- H. Ait Ahsaine, A. BaQais, M. Arab, B. Bakiz and A. Benlhachemi, Catalysts, 2022, 12, 1335 CrossRef CAS.
- Y. Li, J. Liu and X. Huang, Nanoscale Res. Lett., 2008, 3, 365–371 CrossRef CAS.
- A. Kudo and S. Hijii, Chem. Lett., 1999, 28, 1103–1104 CrossRef.
- J. Tang, Z. Zou and J. Ye, Catal. Lett., 2004, 92, 53–56 CrossRef CAS.
- J. Ren, W. Wang, L. Zhang, J. Chang and S. Hu, Catal. Commun., 2009, 10, 1940–1943 CrossRef CAS.
- H. Fu, C. Pan, W. Yao and Y. Zhu, J. Phys. Chem. B, 2005, 109, 22432–22439 CrossRef CAS PubMed.
- Z. Liu, B. Wu, J. Niu, X. Huang and Y. Zhu, Appl. Surf. Sci., 2014, 288, 369–372 CrossRef CAS.
- H. Ait Ahsaine, M. Ezahri, A. Benlhachemi, B. Bakiz, S. Villain, F. Guinneton and J.-R. Gavarri, Ceram. Int., 2016, 42, 8552–8558 CrossRef CAS.
- S. O. Alfaro and A. Martínez-de la Cruz, Appl. Catal., A, 2010, 383, 128–133 CrossRef CAS.
- Z. He, C. Sun, S. Yang, Y. Ding, H. He and Z. Wang, J. Hazard. Mater., 2009, 162, 1477–1486 CrossRef CAS PubMed.
- K. Tekintas, Ö. Kesmez, O. Bekircan and E. T. Saka, J. Mol. Struct., 2022, 1248, 131405 CrossRef CAS.
- W. Nachit, H. Ait Ahsaine, Z. Ramzi, S. Touhtouh, I. Goncharova and K. Benkhouja, Opt. Mater., 2022, 129, 112256 CrossRef CAS.
- L. Zhang and Y. Zhu, Catal. Sci. Technol., 2012, 2, 694–706 RSC.
- F. Amano, K. Nogami and B. Ohtani, Catal. Commun., 2012, 20, 12–16 CrossRef CAS.
- K. S. Knight, Mineral. Mag., 1992, 56, 399–409 CrossRef CAS.
- T. Zeng, H. Yan, H. Ning, J. Zeng and M. J. Reece, J. Am. Ceram. Soc., 2009, 92, 3108–3110 CrossRef CAS.
- H. Yi, L. Qin, D. Huang, G. Zeng, C. Lai, X. Liu, B. Li, H. Wang, C. Zhou, F. Huang, S. Liu and X. Guo, Chem. Eng. J., 2019, 358, 480–496 CrossRef CAS.
- X. Liu, S. Gu, Y. Zhao, G. Zhou and W. Li, J. Mater. Sci. Technol., 2020, 56, 45–68 CrossRef CAS.
- T. Chen, L. Liu, C. Hu and H. Huang, Chin. J. Catal., 2021, 42, 1413–1438 CrossRef CAS.
- L. Zhang, W. Wang, L. Zhou and H. Xu, Small, 2007, 3, 1618–1625 CrossRef CAS PubMed.
- M. Shang, W. Wang, L. Zhang, S. Sun, L. Wang and L. Zhou, J. Phys. Chem. C, 2009, 113, 14727–14731 CrossRef CAS.
- C. Wu, J. Zhong, J. Xie, D. Wang, Y. Shi, Q. Chen, H. Yan and J. Zhu, Appl. Surf. Sci., 2019, 484, 112–123 CrossRef CAS.
- A. J. J. Amalraj and S.-F. Wang, Colloids Surf., A, 2022, 648, 129183 CrossRef.
- W. Guo, L. Jian, X. Wang and W. Zeng, Sens. Actuators, B, 2022, 357, 131396 CrossRef CAS.
- Y.-Q. Wang, C. Yang and L.-H. Gan, Int. J. Hydrog. Energy., 2023, 48, 19372–19384 CrossRef CAS.
- Z. Lin, Z. Yang and J. Huang, Beilstein J. Nanotechnol., 2022, 13, 745–762 CrossRef CAS PubMed.
- T. Liu, F. Xue, B. Wang, R. Wang, W. Cao, X. Zhao, Y. Xia, W. Jin, Y. Zhang and H. Lin, J. Catal., 2023, 417, 41–51 CrossRef CAS.
- Y. Hu, J. Fan, C. Pu, H. Li, E. Liu and X. Hu, J. Photochem. Photobiol., A, 2017, 337, 172–183 CrossRef CAS.
- D. Lv, D. Zhang, X. Pu, D. Kong, Z. Lu, X. Shao, H. Ma and J. Dou, Sep. Purif. Technol., 2017, 174, 97–103 CrossRef CAS.
- T. Ji, E. Ha, M. Wu, X. Hu, J. Wang, Y. Sun, S. Li and J. Hu, Catalysts, 2020, 10, 1161 CrossRef CAS.
- T. Cadenbach, M. J. Benitez, A. L. Morales, C. C. Vera, L. Lascano, F. Quiroz, A. Debut and K. Vizuete, Beilstein J. Nanotechnol., 2020, 11, 1822–1833 CrossRef CAS PubMed.
- Y. Hao, Z. Tian, C. Liu and C. Xiao, Front. Chem., 2023, 11, 19 Search PubMed.
- N. A. McDowell, K. S. Knight and P. Lightfoot, Chem.–Eur. J., 2006, 12, 1493–1499 CrossRef CAS PubMed.
- H. Kodama and A. Watanabe, J. Solid State Chem., 1985, 56, 225–229 CrossRef CAS.
- G. Sankar, M. A. Roberts, J. M. Thomas, G. U. Kulkarni, N. Rangavittal and C. N. R. Rao, J. Solid State Chem., 1995, 119, 210–215 CrossRef CAS.
- D. J. Buttrey, T. Vogt, U. Wildgruber and W. R. Robinson, J. Solid State Chem., 1994, 111, 118–127 CrossRef CAS.
- P. Bégué, R. Enjalbert, J. Galy and A. Castro, Solid State Sci., 2000, 2, 637–653 CrossRef.
- V. I. Voronkova, E. P. Kharitonova and O. G. Rudnitskaya, J. Alloys Compd., 2009, 487, 274–279 CrossRef CAS.
- M. Maczka, L. Macalik, K. Hermanowicz, L. Kępiński and P. Tomaszewski, J. Raman Spectrosc., 2010, 41, 1059–1066 CrossRef CAS.
- J. S. Xue, M. R. Antonio and L. Soderholm, Chem. Mater., 1995, 7, 333–340 CrossRef CAS.
- O. Beaury, M. Faucher and G. Teste de Sagey, Acta Crystallogr., Sect. B: Struct. Crystallogr. Cryst. Chem., 1981, 37, 1166–1170 CrossRef.
- J. A. Alonso, F. Rivillas, M. J. Martınez-Lope and V. Pomjakushin, J. Solid State Chem., 2004, 177, 2470–2476 CrossRef CAS.
- A. V Tyulin and V. A. Efremov, Kristallografiya, 1987, 32, 363–370 Search PubMed.
- A. Watanabe, Mater. Res. Bull., 1980, 15, 1473–1477 CrossRef CAS.
- P. S. Berdonosov, D. O. Charkin, K. S. Knight, K. E. Johnston, R. J. Goff, V. A. Dolgikh and P. Lightfoot, J. Solid State Chem., 2006, 179, 3437–3444 CrossRef CAS.
- H. A. Ahsaine, 2016.
- A. Taoufyq, H. Ait Ahsaine, L. Patout, A. Benlhachemi, M. Ezahri, F. Guinneton, A. Lyoussi, G. Nolibe and J.-R. Gavarri, J. Solid State Chem., 2013, 203, 8–18 CrossRef CAS.
- Z. Zhang, W. Wang, L. Wang and S. Sun, ACS Appl. Mater. Interfaces, 2012, 4, 593–597 CrossRef CAS PubMed.
- H. Takeda, J. S. Han, M. Nishida, T. Shiosaki, T. Hoshina and T. Tsurumi, Solid State Commun., 2010, 150, 836–839 CrossRef CAS.
- D. O. Charkin, D. N. Lebedev, S. Y. Stefanovich and S. M. Kazakov, Solid State Sci., 2010, 12, 2079–2085 CrossRef CAS.
- M. Mączka, A. F. Fuentes, L. Kępiński, M. R. Diaz-Guillen and J. Hanuza, Mater. Chem. Phys., 2010, 120, 289–295 CrossRef.
- T. Šalkus, L. Šatas, A. Kežionis, M. Kodols, J. Grabis, V. Vikhrenko, V. Gunes and M. Barré, Solid State Ionics, 2015, 271, 73–78 CrossRef.
- S. M. Sze and K. K. Ng, Physics of semiconductor devices, John Wiley, NY, 1981, pp. 122–129 Search PubMed.
- V. Nagirnyi, E. Feldbach, L. Jönsson, M. Kirm, A. Lushchik, Ch. Lushchik, L. L. Nagornaya, V. D. Ryzhikov, F. Savikhin, G. Svensson and I. A. Tupitsina, Radiat. Meas., 1998, 29, 247–250 CrossRef CAS.
- A. B. van Oosterhout, J. Chem. Phys., 2008, 67, 2412–2418 CrossRef.
- V. B. Mikhailik, H. Kraus, G. Miller, M. S. Mykhaylyk and D. Wahl, J. Appl. Phys., 2005, 97, 083523 CrossRef.
- V. Nagirnyi, E. Feldbach, L. Jönsson, M. Kirm, A. Kotlov, A. Lushchik, V. A. Nefedov and B. I. Zadneprovski, Nucl. Instrum. Methods Phys. Res., Sect. A, 2002, 486, 395–398 CrossRef CAS.
- A. E. Ovechkin, V. D. Ryzhikov, G. Tamulaitis and A. Žukauskas, Phys. Status Solidi A, 1987, 103, 285–290 CrossRef CAS.
- R. Grasser, E. Pitt, A. Scharmann and G. Zimmerer, Phys. Status Solidi B, 1975, 69, 359–368 CrossRef CAS.
- V. O. Sokolov, V. G. Plotnichenko and E. M. Dianov, Opt. Lett., 2008, 33, 1488–1490 CrossRef CAS PubMed.
- R. Cao, M. Peng and J. Qiu, Opt. Express, 2012, 20, A977–A983 CrossRef PubMed.
- M. Mączka, A. F. Fuentes, K. Hermanowicz, L. Macalik, P. E. Tomaszewski, L. Kępínski and R. Lisiecki, J.
Nanosci. Nanotechnol., 2010, 10, 5746–5754 CrossRef PubMed.
- Z. J. Zhang and X. Y. Chen, Mater. Sci. Eng., B, 2016, 209, 10–16 CrossRef CAS.
- O. M. Bordun, J. Appl. Spectrosc., 1998, 65, 149–151 CrossRef CAS.
- G. Blasse and G. J. Dirksen, Chem. Phys. Lett., 1982, 85, 150–152 CrossRef CAS.
- O. M. Bordun, A. T. Stetskiv and T. M. Yaremchuk, Ukrainian J. Phys., 2004, 49, 991–995 CAS.
- V. Nagirny, E. Feldbach, L. Jonsson, M. Kirm and A. Kotlov, Radiat. Meas., 1998, 29, 247–250 CrossRef.
- G. Blasse, Luminescence and energy transfer, Springer, 2005, pp. 1–41 Search PubMed.
- R. Grasser, A. Scharmann and K.-R. Strack, J. Lumin., 1982, 27, 263–272 CrossRef CAS.
- V. B. Mikhailik, H. Kraus, G. Miller, M. S. Mykhaylyk and D. Wahl, J. Appl. Phys., 2005, 97, 83523 CrossRef.
- H. A. Ahsaine, M. Ezahri, A. Benlhachemi, B. Bakiz, S. Villain, J.-C. Valmalette, F. Guinneton, M. Arab and J.-R. Gavarri, RSC Adv., 2015, 5, 96242–96252 RSC.
- J.-M. Herrmann, Catal. Today, 1999, 53, 115–129 CrossRef CAS.
- A. Elaouni, M. E. Ouardi, M. Zbair, A. BaQais, M. Saadi and H. A. Ahsaine, RSC Adv., 2022, 12, 31801–31817 RSC.
- S. Lotfi, M. E. Ouardi, H. A. Ahsaine and A. Assani, Catal. Rev., 2022, 1–45 CrossRef.
- M. El Ouardi, A. El aouni, H. A. Ahsaine, M. Zbair, A. BaQais and M. Saadi, Chemosphere, 2022, 136483 CrossRef CAS.
- D. Dvoranová, V. Brezova, M. Mazúr and M. A. Malati, Appl. Catal., B, 2002, 37, 91–105 CrossRef.
- L. Zhang, H. Wang, Z. Chen, P. K. Wong and J. Liu, Appl. Catal., B, 2011, 106, 1–13 CAS.
- G. Zhang, Z. Hu, M. Sun, Y. Liu, L. Liu, H. Liu, C.-P. Huang, J. Qu and J. Li, Adv. Funct. Mater., 2015, 25, 3726–3734 CrossRef CAS.
- Y. Zhou, Y. Zhang, M. Lin, J. Long, Z. Zhang, H. Lin, J. C.-S. Wu and X. Wang, Nat. Commun., 2015, 6, 8340 CrossRef PubMed.
- R. Liu, Y. Shi, L. Lin, Z. Wang, C. Liu, J. Bi, Y. Hou, S. Lin and L. Wu, Appl. Surf. Sci., 2022, 605, 154822 CrossRef CAS.
- Y. Liu, B. Wei, L. Xu, H. Gao and M. Zhang, ChemCatChem, 2015, 7, 4076–4084 CrossRef CAS.
- L. Wang, C. Guo, F. Chen, J. Ning, Y. Zhong and Y. Hu, J. Colloid Interface Sci., 2021, 602, 868–879 CrossRef CAS PubMed.
- H. Sun, Z. Tian, G. Zhou, J. Zhang and P. Li, Appl. Surf. Sci., 2019, 469, 125–134 CrossRef CAS.
- E. Gao, W. Wang, M. Shang and J. Xu, Phys. Chem. Chem. Phys., 2011, 13, 2887–2893 RSC.
- B. Y. Alfaifi, A. A. Tahir and K. G. U. Wijayantha, Sol. Energy Mater. Sol. Cells, 2019, 195, 134–141 CrossRef CAS.
- M. B. Tahir, T. Nawaz, G. Nabi, M. Sagir, M. Rafique, A. Ahmed and S. Muhammad, Int. J. Hydrogen Energy, 2020, 45, 22833–22847 CrossRef CAS.
- C. Wang, L. Zhu, M. Wei, P. Chen and G. Shan, Water Res., 2012, 46, 845–853 CrossRef CAS PubMed.
- B. Fatima, S. I. Siddiqui, R. Ahmed and S. A. Chaudhry, Water Resour. Ind., 2019, 22, 100119 CrossRef.
- S. Zinatloo-Ajabshir, M. Baladi and M. Salavati-Niasari, Ultrason. Sonochem., 2021, 72, 105420 CrossRef CAS PubMed.
- Ch. V. Reddy, R. Koutavarapu, K. R. Reddy, N. P. Shetti, T. M. Aminabhavi and J. Shim, J. Environ. Manage., 2020, 268, 110677 CrossRef CAS PubMed.
- H. J. Khadim, A. Al-Farraji and S. H. Ammar, Environ. Nanotechnol., Monit. Manage., 2022, 18, 100722 CAS.
- H. Chen and Y. Xu, Appl. Surf. Sci., 2014, 319, 319–323 CrossRef CAS.
- H. Fu, L. Zhang, W. Yao and Y. Zhu, Appl. Catal., B, 2006, 66, 100–110 CrossRef CAS.
- Y. Zhang and Y. J. Xu, 2014.
- R. P. Panmand, Y. A. Sethi, S. R. Kadam, M. S. Tamboli, L. K. Nikam, J. D. Ambekar, C.-J. Park and B. B. Kale, CrystEngComm, 2015, 17, 107–115 RSC.
- C. Xu, X. Wei, Y. Guo, H. Wu, Z. Ren, G. Xu, G. Shen and G. Han, Mater. Res. Bull., 2009, 44, 1635–1641 CrossRef CAS.
- F.-J. Zhang, F.-Z. Xie, J. Liu, W. Zhao and K. Zhang, Ultrason. Sonochem., 2013, 20, 209–215 CrossRef CAS PubMed.
- G. Tan, J. Huang, L. Zhang, H. Ren and A. Xia, Ceram. Int., 2014, 40, 11671–11679 CrossRef CAS.
- J. Wang, J. Li, N. Zhao, J. Sha, S. Hao, E. Liu, C. Shi, C. He and D. Wang, Appl. Surf. Sci., 2015, 324, 698–704 CrossRef CAS.
- N. D. Phu, L. H. Hoang, P. Van Hai, T. Q. Huy, X.-B. Chen and W. C. Chou, J. Alloys Compd., 2020, 824, 153914 CrossRef.
- C.-J. Chang, C.-W. Wang, Y.-H. Wei and C.-Y. Chen, Int. J. Hydrogen Energy, 2018, 43, 11345–11354 CrossRef CAS.
- Q. Zhao, M. Gong, W. Liu, Y. Mao, S. Le, S. Ju, F. Long, X. Liu, K. Liu and T. Jiang, Appl. Surf. Sci., 2015, 332, 138–146 CrossRef CAS.
- J. Ma, B. Zhao, X. Fan, W. Wang, X. Chen, N. Shao and P. Jiang, Diamond Relat. Mater., 2022, 127, 109143 CrossRef CAS.
- C.-J. Chang, J.-K. Chen, K.-S. Lin, Y.-H. Wei, P.-Y. Chao and C.-Y. Huang, J. Alloys Compd., 2020, 813, 152186 CrossRef CAS.
- X. Zheng, Y. Chu, B. Miao and J. Fan, J. Alloys Compd., 2022, 893, 162382 CrossRef CAS.
- J. Yang, L. Li, F. Fu, H. Xu, K. Da, S. Cao, W. Chen, L. Yang and X. Fan, Appl. Surf. Sci., 2023, 610, 155598 CrossRef CAS.
- J. Shen, J. Xue, Z. Chen, J. Ni, B. Tang, G. He and H. Chen, J. Mater. Sci., 2018, 53, 4848–4860 CrossRef CAS.
- J. Yang, X. Wang, Y. Chen, J. Dai and S. Sun, RSC Adv., 2015, 5, 9771–9782 RSC.
- Y. Zhou, P. Lv, W. Zhang, X. Meng, H. He, X. Zeng and X. Shen, Appl. Surf. Sci., 2018, 457, 925–932 CrossRef CAS.
- B. Li, L. Tan, X. Liu, Z. Li, Z. Cui, Y. Liang, S. Zhu, X. Yang, K. W. Kwok Yeung and S. Wu, J. Hazard. Mater., 2019, 380, 120818 CrossRef CAS PubMed.
- J. Wan, Y. Zhang, R. Wang, L. Liu, E. Liu, J. Fan and F. Fu, J. Hazard. Mater., 2020, 384, 121484 CrossRef CAS PubMed.
- A. Phuruangrat, S. Buapoon, T. Bunluesak, P. Suebsom, S. Wannapop, T. Thongtem and S. Thongtem, Solid State Sci., 2022, 128, 106881 CrossRef CAS.
- X. Hu, J. Tian, Y. Xue, Y. Li and H. Cui, ChemCatChem, 2017, 9, 1511–1516 CrossRef CAS.
- Z. Zhang, W. Wang, E. Gao, M. Shang and J. Xu, J. Hazard. Mater., 2011, 196, 255–262 CrossRef CAS PubMed.
- L. G. Devi, N. Kottam and S. G. Kumar, J. Phys. Chem. C, 2009, 113, 15593–15601 CrossRef CAS.
- S.-S. Lee, B. T. Huy, N. T. K. Phuong, D. K. Tung and Y.-I. Lee, Korean J. Chem. Eng., 2019, 36, 1716–1723 CrossRef CAS.
- N. Tahir, M. Zahid, I. A. Bhatti and Y. Jamil, Environ. Sci. Pollut. Res., 2022, 29, 6552–6567 CrossRef CAS PubMed.
- H. Su, S. Li, L. Xu, C. Liu, R. Zhang and W. Tan, J. Phys. Chem. Solids, 2022, 170, 110954 CrossRef CAS.
- C. Du, X. Ma, H. Xue, F. Wang, H. Yang, L. Zhang, L. Ma, L. Jia and K. Wang, Bull. Mater. Sci., 2022, 45, 95 CrossRef CAS.
- R. Malolan, R. S. Jayaraman, S. Adithya, J. Arun, K. P. Gopinath, P. SundarRajan, O. Nasif, W. Kim and M. Govarthanan, Chemosphere, 2021, 266, 128963 CrossRef CAS PubMed.
- L. X. Lovisa, T. B. O. Nunes, R. Wilson, E. Longo, M. Daldind, M. R. D. Bomio and F. V Motta, Dalton Trans., 2022, 51, 17700–17710 RSC.
- S. Bera, S. Samajdar, S. Pal, P. S. Das, L. A. H. Jones, H. Finch, V. R. Dhanak and S. Ghosh, Ceram. Int., 2022, 48, 35814–35824 CrossRef CAS.
- V. Koteski, J. Belošević-Čavor, V. Ivanovski, A. Umićević and D. Toprek, Appl. Surf. Sci., 2020, 515, 146036 CrossRef CAS.
- Y. Zheng, J. Bao and Y. Sun, J. Iran. Chem. Soc., 2022, 1–10 Search PubMed.
- X. Zhong, W. T. Wu, H. N. Jie, W. Y. Tang, D. Y. Chen, T. Ruan and H. P. Bai, RSC Adv., 2020, 10, 38024 RSC.
- X.-X. Deng, S. Tian, Z.-M. Chai, Z.-J. Bai, Y.-X. Tan, L. Chen, J.-K. Guo, S. Shen, M.-Q. Cai and C.-T. Au, Ind. Eng. Chem. Res., 2020, 59, 13528–13538 CrossRef CAS.
- M. Arif, M. Zhang, J. Yao, H. Yin, P. Li, I. Hussain and X. Liu, J. Alloys Compd., 2019, 792, 878–893 CrossRef CAS.
- M. Arif, M. Zhang, Y. Mao, Q. Bu, A. Ali, Z. Qin, T. Muhmood, Shahnoor, X. Liu, B. Zhou and S. Ming Chen, J. Colloid Interface Sci., 2021, 581, 276–291 CrossRef CAS PubMed.
- N. Mahhumane, L. M. Cele, C. Muzenda, O. V. Nkwachukwu, B. O. Orimolade, B. A. Koiki, L. Tshwenya and O. A. Arotiba, Mater. Today Commun., 2022, 33, 104804 CrossRef CAS.
- H. Luo, B. Zhao, M. Zhang, Y. Liu, R. Han and L. Liu, New J. Chem., 2019, 43, 15335–15341 RSC.
- X. Zhang, M. Wang, X. Jia, K. Cao and M. Zhang, ChemistrySelect, 2019, 4, 12785–12793 CrossRef CAS.
- R. B. Hughes-Currie, P. S. Senanayake, J.-P. R. Wells, M. F. Reid, G. Berden, R. J. Reeves and A. Meijerink, Phys. Rev. B, 2013, 88, 104304 CrossRef.
- R. Zamiri, A. F. Lemos, A. Reblo, H. A. Ahangar and J. M. F. Ferreira, Ceram. Int., 2014, 40, 523–529 CrossRef CAS.
- X. Wang, Z. Cao, Y. Zhang, H. Xu, S. Cao and R. Zhang, Chem. Eng. J., 2020, 385, 123782 CrossRef.
- H. Wu, X. Liu, J. Wen, Y. Liu and X. Zheng, Colloids Surf., A, 2021, 610, 125933 CrossRef CAS.
- N. Tian, Y. Zhang, H. Huang, Y. He and Y. Guo, J. Phys. Chem. C, 2014, 118, 15640–15648 CrossRef CAS.
- H. A. Ahsaine, A. Slassi, M. Ezahri, A. Benlhachemi, B. Bakiz, F. Guinneton and J.-R. Gavarri, RSC Adv., 2016, 6, 101105–101114 RSC.
- L. N. Guo and S. Ren, Appl. Mech. Mater., 2016, 851, 72–77 Search PubMed.
- H. Gu, L. Yu, J. Wang, M. Ni, T. Liu and F. Chen, Spectrochim. Acta, Part A, 2017, 177, 58–62 CrossRef CAS PubMed.
- X. Zhang, M. Zhang and K. Cao, CrystEngComm, 2019, 21, 6208–6218 RSC.
- C. Wang, C. Gu, T. Zeng, Q. Zhang and X. Luo, J. Rare Earths, 2021, 39, 58–66 CrossRef CAS.
- X. Li, W. Li, S. Gu, X. Liu, H. Li, C. Ren, X. Ma and H. Zhou, J. Alloys Compd., 2021, 851, 156935 CrossRef CAS.
- X. Zhang, J. Iran. Chem. Soc., 2022, 19, 3029–3041 CrossRef CAS.
- Y. Qiu, J. Lu, Y. Yan and J. Niu, J. Hazard. Mater., 2022, 422, 126920 CrossRef CAS PubMed.
- H. Li, H. Hao, S. Jin, W. Guo, X. Hu, H. Hou, G. Zhang, S. Yan, W. Gao and G. Liu, Adv. Powder Technol., 2018, 29, 1216–1221 CrossRef CAS.
- X. Li, W. Li, X. Liu, H. Fan, L. Geng, X. Ma, M. Dong and S. Liu, J. Alloys Compd., 2021, 889, 161757 CrossRef.
- Z. Liu, L. Shen, X. He, E. Y. B. Pun and H. Lin, Colloid Interface Sci. Commun., 2021, 44, 100494 CrossRef CAS.
- X. Zhang, Y. Zhao, J. Li, W. Gai and Y. Gu, J. Phys. Chem. Solids, 2022, 171, 110998 CrossRef CAS.
- X. Li, W. Li, X. Liu, L. Geng, H. Fan, X. Ma, M. Dong and H. Qiu, Appl. Surf. Sci., 2022, 592, 153311 CrossRef CAS.
- M. Shang, W. Wang, L. Zhang and H. Xu, Mater. Chem. Phys., 2010, 120, 155–159 CrossRef CAS.
- H. A. Ahsaine, Etude de tungstate de bismuth (Bi, Lu) 2WO6: Evolution des propriétés physicochimiques, luminescentes et photocatalytiques, PhD thesis, Ibn Zohr, 2016 Search PubMed.
- X. Ding, K. Zhao and L. Zhang, Environ. Sci. Technol., 2014, 48, 5823–5831 CrossRef CAS PubMed.
- H. Huang, K. Liu, K. Chen, Y. Zhang, Y. Zhang and S. Wang, J. Phys. Chem. C, 2014, 118, 14379–14387 CrossRef CAS.
- Y. Fu, C. Chang, P. Chen, X. Chu and L. Zhu, J. Hazard. Mater., 2013, 254, 185–192 CrossRef PubMed.
- L. H. Hoang, N. D. Phu, H. Peng and X.-B. Chen, J. Alloys Compd., 2018, 744, 228–233 CrossRef CAS.
- Y. Zhang, Y. Zhao, Z. Xiong, T. Gao, B. Gong, P. Liu, J. Liu and J. Zhang, Appl. Catal., B, 2021, 282, 119534 CrossRef CAS.
- Y. W. Teh, C. Er, X. Y. Kong, B. Ng, S. Yong and S. Chai, ChemSusChem, 2022, 15, e202200471 CrossRef CAS PubMed.
|
This journal is © The Royal Society of Chemistry 2023 |