DOI:
10.1039/D3RA01570J
(Paper)
RSC Adv., 2023,
13, 21318-21326
Highly selective optical sensor N/S-doped carbon quantum dots (CQDs) for the assessment of human chorionic gonadotropin β-hCG in the serum of breast and prostate cancer patients
Received
9th March 2023
, Accepted 4th July 2023
First published on 17th July 2023
Abstract
A low-cost, accurate, and highly selective method was used for the assessment of the human chorionic gonadotropin β-hCG in the serum of breast and prostate cancer patients. This method is based on enhancing the intensity of luminescence displayed by the optical sensor N/S-doped carbon dots (CQDs) upon adding different concentrations of β-hCG. The luminescent optical sensor was synthesized and characterized through absorption and emission and is tailored to present blue luminescence at λem = 345 nm and λex = 288 nm at pH 7.8 in DMSO. The enhancement of the luminescence intensity of the N/S-doped CQDs, especially, the characteristic band at λem = 345 nm, is typically used for determining β-hCG in different serum samples. The dynamic range is 1.35–22.95 mU mL−1, and the limit of detection (LOD) and quantitation limit of detection (LOQ) are 0.235 and 0.670 mU mL−1, respectively. This method was practical, simple, and relatively free from interference effect. It was successfully applied to measure PCT in the samples of human serum, and from this method, we can assess some biomarkers of cancer-related diseases in human body.
1. Introduction
The discovery of carbon dots (CDs) was mainly in 2004 during the sorted single-walled carbon nanotube (SWCNT) purification process via preparative electrophoresis. CDs are classed as a novel fluorescent small carbon nanomaterial with less than 10 nm particle size.1–3
Carbon dots (CDs) are highly-emissive nanoparticles obtained through fast and cheap syntheses as a new type of carbon-based nanomaterial. They have attracted considerable attention of researchers for years owing to their diverse physicochemical properties and promising attributes, such as high biocompatibility and unique optical properties. These nanomaterials have outstanding water solubility with low toxicity and low production cost and offer tunable fluorescence emission and excitation. They are photochemically and physicochemically stable, eco-friendly, and have abundant functional groups (e.g., amino, hydroxyl, and, carboxyl), high stability, superficial synthesis methods, easy surface modification, and electron mobility.2–4
Carbon dots (CDs) have a wide range of applications in bio-imaging fields, bio-sensing, drug-gene delivery system, disease discovery, synthetic chemistry, in vitro and in vivo bio-imaging, chemical and biological sensing, and photodynamic and photo-thermal therapies. The development of various morphologies, sizes, and target-specific carbon dots represent future areas of study.3
Human chorionic gonadotropin (HCG) is a glycoproteinhormone endogenously secreted by the placenta that is composed of 2 noncovalently linked molecules, namely, the α and the β subunits. The earliest indication of an association between HCG and neoplasms was the identification of HCG as a tumor marker.5
The β-subunit of the peptide hormone (β-hCG) was recognized in different germ cell and non-germ cell tumors, and it was used previously to monitor response to treatment and to detect tumor relapse.6 The elevated level of β-hCG was observed in prostate7 and breast cancer8 patients. The diagnosis of breast cancer in the current scenario is done by mammogram, MRI and, MR spectroscopy, and breast biopsy tests. All these detection techniques are very costly and time-consuming, involve surgical techniques, and the results are sometimes negative. Hence, there is a need to develop a cost-effective technique that can qualitatively and/or quantitatively detect breast cancer at an early stage. Therefore, the development of a highly sensitive sensor system for the monitoring of early-stage breast cancer is required, so that the patient can stay alert about the risk of diagnosis.9
Several techniques were used to evaluate β-hCG, such as immunologic and reverse transcriptase-polymerase chain reaction (RT-PCR), in prostate cancer,10 but different studies11 showed that they did not help. It was reported that hCG is not detected in normal prostate tissues, but is specific for low-grade prostate cancer,10 resulting in a lack of quantification in these studies.
In this study a highly selective and straightforward analytical strategy was adopted for the accurate, fast, and affordable determination of human chorionic gonadotropin β-hCG in the serum of breast and prostate cancer patients. It depends on enhancing the luminescence intensity of N/S-doped carbon dot optical sensor after the addition of β-hCG with different concentrations. The newly designed sensor was fabricated and characterized via absorption and emission specifications and is customized to exhibit blue luminescence in DMSO at a λem of 345 nm when excited at 288 nm. The main mechanism through which the sensor functions is the fluorescence resonance energy transfer (FRET). The obtained results were statistically analyzed and were found to be satisfactory. The limit of detection of the suggested probe was 6.7 × 10−10 mIU mL−1 with a correlation coefficient of 0.98.
2. Experimental
2.1. Instrumentation
A double-beam UV-Vis spectrophotometer (model: Edinburgh Instruments DS5) equipped with a Xenon flash lamp with a spectral range of 190–1100 nm. A spectrofluorometer (Edinburgh Instruments FS5) having a spectral range up to 1650 nm and fluorescence lifetimes down to 25 ps. A pH meter (model: Jenway-3040). A Daihan Scientific centrifuge device (model: CF-10). Fourier transform infrared (FTIR) (model: Shimadzu-FTIR-8400 S, Japan). The structures of the phases formed were examined using a high-resolution transmission electron microscope (HR-TEM) with an acceleration voltage up to 200 kV (JEM-2100-JEOL, Japan). The oxidation states and species in the prepared materials were determined by a Thermo Scientific™ K-Alpha™ X-ray photoelectron spectrometer (XPS) (Thermo Scientific, USA), using Al-Kα micro-focused monochromator within an energy range up to 4 keV. The X-ray diffraction (XRD) analysis of N/S-doped QDs was performed with a D8-AVANCE X-ray diffractometer (Bruker, Germany) with Cu-Kα radiation (λ = 0.154056 nm) for the identification of the crystalline phase, relative crystallinity, and crystal size of the as-prepared N/S-doped QDs. The XRD analysis was performed in the 2θ range from 3.0° to 80.0° with a 0.020° step at a scan speed of 0.4 s.
2.2. Materials
Ethanol, acetonitrile, dimethyl sulfoxide (DMSO), and dimethyl formamide (DMF), citric acid monohydrate, and thiourea (TU) were purchased from Sigma-Aldrich. Human chorionic gonadotropin (β-hCG) was purchased from BioMérieux, Inc. Human serum samples were collected from Raba'a El-Adwyea hospital (Nasr city, Egypt). The procedure for collecting human specimens was carried out in compliance with World Health Organization (WHO) approval. The ethics committee at Ain Shams University gave its approval for all tests to be carried out in accordance with the guidelines established by the “Ministry of Health and Population, Egypt.” The humans participating in this study provided informed consents.
2.3. Reagents and solutions
2.3.1. Preparation of N/S-doped CQDs. The N/S-doped CQDs was prepared by the carbonization of citric acid in a similar method reported previously with some modification.12 Briefly, 1.0 g of citric acid and 0.5 g of thiourea (TU) were ground and mixed thoroughly to make it homogenous. The solid mixture was transferred to a 100 mL beaker and heated to 200 °C in a muffle for 20 min. A brownish-yellow thick syrup solution was obtained from the thermal process. After cooling to room temperature, the thick solution was diluted to 100 mL with Milli-Q water and then subjected to dialysis in a dialysis bag (M.W. cutoff: 5 kDa). Fig. 1.
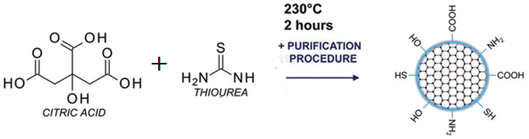 |
| Fig. 1 Scheme for the preparation of N/S-doped CQDs. | |
2.3.2. Stock and working solutions. The concentration of the stock solution of N/S-doped CQDs is 1 × 10−2 mol L−1. The Stock solution of β-hCG was prepared by dissolving 0.5 g of N/S-doped CQDs in 2 mL deionized distilled water. In a 25 mL volumetric flask, the working solution of N/S-doped CQDs was obtained by transferring 0.25 mL of the stock solution of N/S-doped CQDs into DMSO solvent, and the volume was adjusted to the mark to obtain a final concentration of 1 × 10−4 mol L−1. To prepare β-hCG working solution, 400 μL from its corresponding stock solution was accurately added to 2 mL water. From the later working solution, a calibration set for β-hCG was prepared. When not in use, all stock and working solutions were kept at 2–8 °C. The stated procedures were followed for performing the subsequent measurements of absorption and emission spectra and pH, molar ratio, and solvent effects. The intensity of luminescence was recorded at λex/λem = 288/345 nm.
2.4. General procedure
2.4.1. Calibration curve. The optical probe N/S-doped CQDs was thoroughly mixed with the standard solution of β-hCG with different concentrations, as previously detailed, in the spectrophotometer cell. The luminescence spectra were then recorded at the chosen λex = 288 nm. Then, a relation between the peak intensity on Y-axis against the concentration of β-hCG on X-axis was drawn.
2.4.2. Determination of β-hCG in serum samples. 1.0 mL of blood sample each from 11 healthy volunteers were collected and centrifuged for 15 min at 4000 rpm to remove proteins. The concentration of β-hCG was determined from the corresponding calibration graph.
3. Results and discussion
3.1. FT-IR spectrum
Fourier transform infrared (FT-IR) spectroscopy was applied to confirm the surface functional groups of the N/S-doped CQD sensor. As shown in Fig. 2A, FT-IR spectra exhibit the peaks of O–H bending and stretching vibrations at 671 and 3679 cm−1, respectively. The peak at 3444 cm−1 in 3000–3744 cm−1 range represents N–H stretching vibrations.13–15 The peak at 1253 cm−1 was attributed to O–H in-plane bending vibrations.16 These considerable amino, hydroxyl hydrophilic groups could enable N,S-CQDs superior hydrophilicity.17 The peak at 1706 cm−1 is attributed to the stretch vibration of C
O carboxylic groups and C
N bonds,18 and the two peaks at 1646 and 1516 cm−1 are characteristic to the vibration of amide group stretching C
O (amide I) and the in-plane bending of N–H bond (amide II),18,19 respectively. The peak at 1072 cm−1 is attributed to C–O stretching vibrations.20 Then, the peaks at 1595 and 1695/1741 cm−1 are attributed to C
C and C
O stretching vibrations, respectively.21,22 The peaks at 1427 and 1395 cm−1 can be assigned to the vibrations of C–S and C–N, respectively,23 and the peak at 1184 cm−1 further confirm the existence of C–O bond.17,23
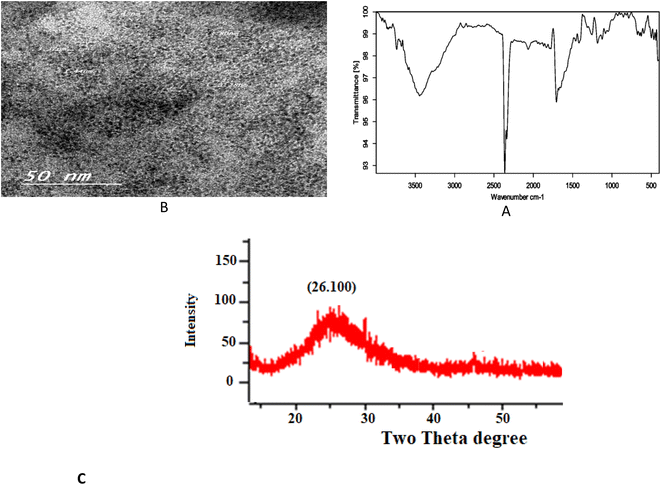 |
| Fig. 2 (A) TEM image, (B) FT-IR spectrum, and (C) XRD patterns. | |
3.2. TEM, XRD and XPS measurements
To reveal the size and morphology of the N/S-doped CQDs, HRTEM was employed, and the result is presented in Fig. 2B. HR-TEM images showed spherical N/S-doped CQDs with a narrow size distribution (1.6–3.7 nm) and an average size of 2.8 nm. The XRD pattern (Fig. 2C) displayed a broad peak (2θ ≈ 26.100°), which corresponded to the (002) plane. The result is similar to that of graphite and indicated the presence of highly disordered carbon atoms.24–26 Although XRD measurement is the best technique to evaluate the structure of N/S-doped CQDs, results from FT-IR spectroscopy, XPS, and UV-Vis measurements are also very helpful. In general, amorphous N/S-doped CQDs are mainly composed of sp3 carbons, whereas crystalline CDs have sp2 hybridized carbon domains.27,28 As will be discussed in the next few sections, the results from XPS, FT-IR spectroscopy, and UV-Vis measurements showed the presence of sp3 and sp2 carbon structures. Hence, these results could demonstrate the coexistence of crystalline and disordered (amorphous) carbon domains in the N/S-doped CQD structure. In addition, from the XPS survey spectrum (Fig. 3a), the peaks at 531.08, 398.08, 284.08, and 162.08 eV could be related to O 1s, N 1s, C 1s, and S 2p, respectively.29 The two peaks presented in the high-resolution XPS of S2P (Fig. 3b) at 162.38 and 166.78 eV could be assigned to 2p3/2 (–C–S–C– covalent bond) and S–O bonding, respectively.30,31 Also, high-resolution XPS of O 1s (Fig. 3c) showed the presence of covalent O and ketonic O atoms (peaks at 534 and 530 eV).32 N 1s (Fig. 3d) showed the presence of pyridinic N atoms (peak at 398.50 eV).32 However, the deconvoluted peak of C 1s showed the presence of three peaks, which can be attributed to the sp2 C in graphene (283.68 eV), sp3 C from C–N/C–S and C–O (285.25 eV), and carboxyl groups (288.4 eV) (Fig. 2E).33 More interestingly, XPS and FT-IR spectroscopy results are in agreement with each other and proved the presence of various surface functional groups and effective doping of S and N atoms into CQDs.
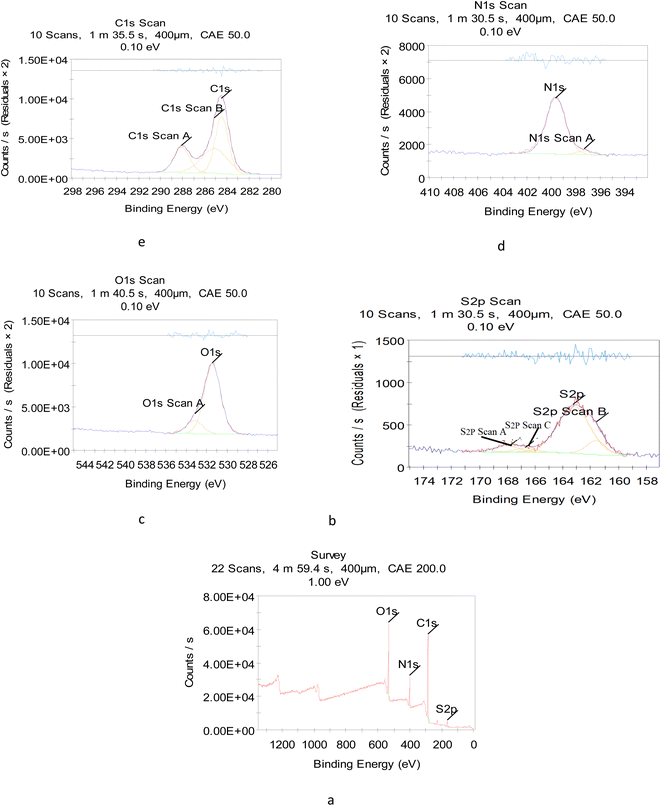 |
| Fig. 3 XPS (a) full survey, (b) S 2p, (c) O 1s, (d) N 1s, and (e) C 1s spectra. | |
3.3. Absorption spectra
Depending on the solvent's polarity, the aprotic solvent used in UV-Vis absorption and the emission fluorescence spectra of the C-dots influenced the positions and intensity. The absorption spectra of the N/S-doped CQD sensor in different solvents are shown in Fig. 4, indicating a high absorption in DMSO solvent.
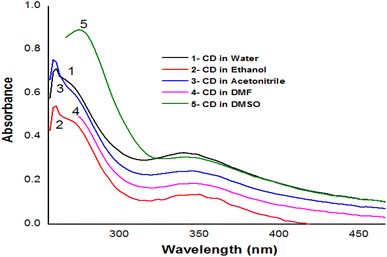 |
| Fig. 4 Absorption spectra of CD sensor in different solvents. | |
The absorption spectra of N/S-doped CQD sensor in DMSO with different concentrations of β-hCG are illustrated in Fig. 5. Spectrum1 represents the absorption spectrum of free N/S-doped CQDs exhibiting a prominent peak at 260 nm and a tail extending into the visible range. This is attributed to the n–π* transition of C
O bond and π–π* transition of C
C bond electronic transitions in N/S-doped CQD rings.14,34,35 While spectra 2, 3, and 4 indicate higher absorption after the addition of 5.4, 45.9, and 72.9 mU mL−1 of β-hCG, respectively.
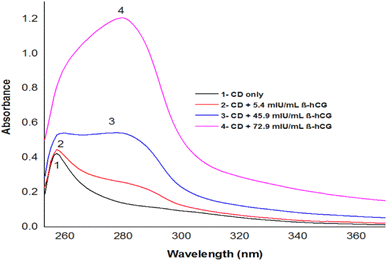 |
| Fig. 5 Absorption spectra of CD sensor in DMSO with different concentrations of β-hCG. | |
3.4. Emission spectra
The emission spectra of N/S-doped CQDs in different concentrations of β-hCG show that the intensity of the characteristic peak at λem = 345 nm of N/S-doped CQDs is enhanced due to energy transfer from the optical sensor to β-hCG, Fig. 6. The mechanism of the energy transfer is taken place by electron transfer from the N-linked protein glycosylation (N-glycosylation of N-glycans) at Asn residues (Asn-x-Ser/Thr motifs) in the glycoproteins of β-hCG to the N/S-doped CQDs. The N-linked protein glycosylation is a post-translational modification that occurs in many glycoproteins, including β-hCG. In this modification, a sugar molecule is attached to the Asn residue of the protein. The sugar molecule has a negative charge, which attracts the positively charged N/S-doped CQDs. This attraction brings the N/S-doped CQDs close to the protein, and allows for the electron transfer to occur. The electron transfer from the protein to the N/S-doped CQDs causes an increase in the energy of the CQDs. This increase in the energy is released as light, which enhanced the intensity of the characteristic peak at λem = 345 nm of the N/S-doped CQDs. The energy transfer from the N/S-doped CQDs to β-hCG can be used to detect β-hCG in a sample. The intensity of the characteristic peak at λem = 345 nm of the N/S-doped CQDs is directly proportional to the concentration of β-hCG in the sample. This means that the intensity of the peak can be used to quantify the concentration of β-hCG in the sample, Fig. 7.
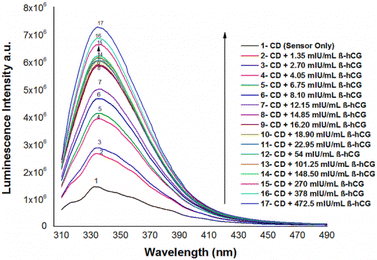 |
| Fig. 6 Luminescence emission spectra of N/S-doped CQDs in the presence of different concentrations of β-hCG in DMSO at λex = 288 nm. | |
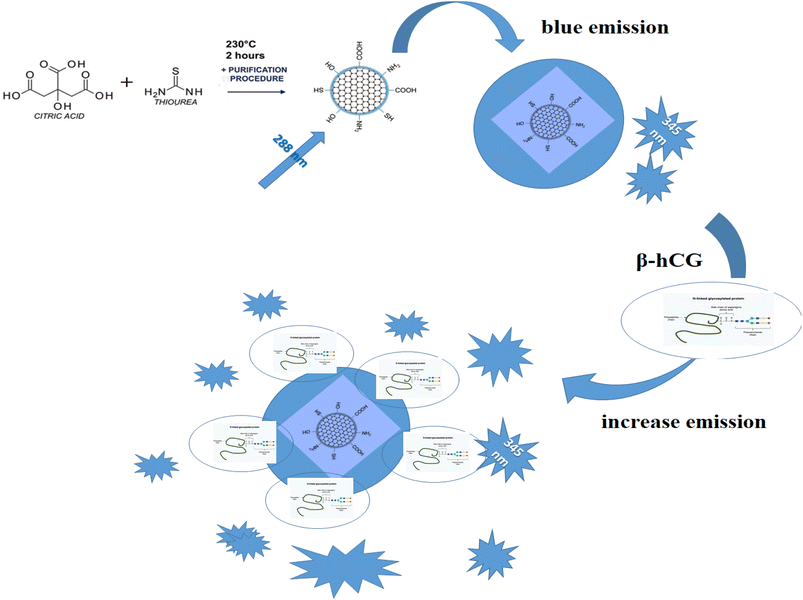 |
| Fig. 7 Mechanism of the energy transfer between β-hCG to the N/S-doped CQD. | |
3.5. Effect of experimental variables
3.5.1. Effect of solvent. The influence of the solvent on the luminescence intensities of the solutions containing N/S-doped CQDs was studied under the above-mentioned conditions. The results show the enhanced emission of N/S-doped CQDs in DMSO with pH = 7.8, as shown in Fig. 8. Also, the luminescence intensity for the N/S-doped CQDs in the DMSO solution is stronger than in DMF, acetonitrile, water, and ethanol. This can be attributed to the interactions between the solvent and N/S-doped CQDs, which affects the energy difference between the ground and the excited states, and the orientation polarizability of solvents. The dipole moment represents the electron distribution in a molecule with a specific structure. In combination with the reactive field around it, the dipole moment plays an essential role in the transition of a molecule because a molecule can absorb light when its dipole moment changes.35
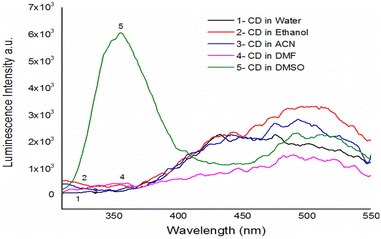 |
| Fig. 8 Luminescence emission spectra of N/S-doped CQDs in the presence of different solvents at λex = 288 nm. | |
4. Analytical performance
4.1. Method validation
4.1.1. Analytical parameters of optical sensor method. A linear correlation was found between the luminescence intensity of the optical sensor at λem = 345 nm and the concentration of β-hCG in the range of 1.35–22.95 mIU mL−1, as shown in Fig. 9, and a calibration curve was obtained by plotting the peak intensity of the N/S-doped CQDs versus the concentration of β-hCG at λem = 345 nm. The limit of detection (LOD) and quantitation (LOQ) calculated according to the ICH guidelines36 using the formula: LOD = 3.3S/b (equals to 0.234 mIU mL−1) and LOQ = 10S/b (equals to 0.67 mIU mL−1) (where S is the standard deviation of blank luminescence intensity value, and b is the slope of the calibration plot) are also given in Table 1.37–58
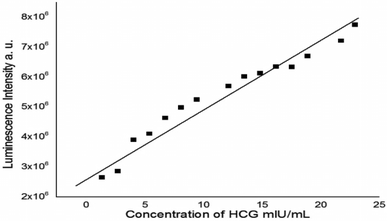 |
| Fig. 9 Linear relationship between the luminescence intensity of N/S-doped CQDs and different concentrations of β-hCG at λem = 345 nm. | |
Table 1 Sensitivity and regression parameters for photo probea
Parameter |
Value |
Where Y = fluorescence intensity, X = β-hCG concentration in mIU mL−1, a = intercept, b = slope. |
λ em (nm) |
345 |
Liner range, (mIU mL−1) |
1.35–22.95 |
Limit of detection (LOD), (mIU mL−1) |
0.670 |
Limit of quantitation (LOQ) (mIU mL−1) |
0.236 |
Regression equation, Y* |
Y = a + bX |
Intercept (a) |
2.27 × 106 |
Slope (b) |
3.37 × 105 |
Standard deviation |
6.86 × 10−5 |
Variance (Sa2) |
4.70 × 10−9 |
Regression coefficient (r) |
0.98 |
4.1.2. Selectivity. The method selectivity and validity was investigated by studying the effect of possible interfering substances on the luminescence spectra of the N/S-doped CQDs after the addition of 24 mIU mL−1. The interfering substances included bilirubin (40 mg dL−1), hemoglobin (1 mg dL−1), ascorbic acid (20 mg dL−1), glucose (2000 mg dL−1), and triglycerides (1200 mg dL−1). It was shown that all interfering substances have no significant influence on the luminescence intensity of the N/S-doped CQD sensor.
4.1.3. Application to formulations. For testing the applicability of the proposed method, it was applied for the determination of β-hCG concentration for 11 serum samples of the health state human. The results in Table 2 show that the method is successful for the determination of β-hCG in serum samples. It is clear that the average value of the β-hCG concentration for patients obtained by the proposed method is 30
003 ± 3.91 mIU mL−1 and that obtained by the standard method is 30
000 mIU mL−1, highlighting excellent accuracy and precision. The average recovery and RSD for the serum samples in our method found to be 100.35 ± 1.96% and were also presented for comparison and show a good correlation with those of the reference method and with the label claimed.
Table 2 Evaluation of intra-day an inter-day accuracy and precisiona
Sample |
Standard method average mIU mL−1 |
Propose method |
Intra-day accuracy and precision (n = 3) |
Inter-day accuracy and precision (n = 3) |
Average found |
% RE |
Average recovery |
% RSD |
Average found |
% RE |
Average recovery |
% RSD |
mIU mL−1 |
±CL |
mIU mL−1 |
±CL |
% RE, percent relative error. % RE = [(concentration proposed − concentration known)/concentration known] × 100, % RSD, relative standard deviation. % RSD = [S/(average measurements)] × 100, and ±CL, confidence limits: CL = tS/n(1/2). (The tabulated value of t is 4.303, at the 95% confidence level; S = standard deviation and n = number of measurements). |
Patient (1) |
30 000 |
30 003 |
±3.91 |
0.010 |
100.01 |
0.005 |
29 998 |
±3.92 |
0.007 |
99.99 |
0.005 |
Patient (2) |
21 000 |
21 002 |
±2.48 |
0.009 |
100 |
0.0047 |
21 001 |
±2.48 |
0.03 |
100 |
0.0047 |
Patient (3) |
920 |
921.3 |
±1.29 |
0.14 |
100.14 |
0.056 |
920.67 |
±1.88 |
0.29 |
100.07 |
0.08 |
Patient (4) |
863 |
862.66 |
±1.41 |
0.039 |
99.96 |
0.066 |
862 |
±1.86 |
0.12 |
99.88 |
0.087 |
Patient (5) |
370 |
370.66 |
±1.41 |
0.178 |
100.18 |
0.15 |
370.33 |
±1.88 |
0.089 |
100.09 |
0.016 |
Patient (6) |
226 |
225.67 |
±1.88 |
0.55 |
99.85 |
0.33 |
226.17 |
±1.41 |
0.075 |
100.07 |
0.25 |
Patient (7) |
179 |
178 |
±2.13 |
0.56 |
99.44 |
0.48 |
179.77 |
±0.62 |
0.43 |
100.43 |
0.13 |
Patient (8) |
100 |
99.90 |
±0.71 |
0.10 |
99.90 |
0.29 |
99.98 |
±0.868 |
0.02 |
99.68 |
0.35 |
Patient (9) |
80 |
79.16 |
±1.06 |
0.625 |
98.95 |
0.96 |
80.63 |
±1 |
0.78 |
100.79 |
0.54 |
Patient (10) |
48 |
48.33 |
±1.88 |
0.68 |
100.69 |
1.57 |
47.82 |
±2.20 |
0.375 |
99.63 |
1.80 |
Patient (11) |
17 |
17.30 |
±0.63 |
1.76 |
101.76 |
1.44 |
16.83 |
±1.6 |
1 |
99 |
3.92 |
4.1.4. Recovery study. To further evaluate the accuracy of the method recovery experiments was assessed by determining the agreement between the measured standard concentration and the added known concentration to the sample. In all cases, the values of the recovery percentage ranged between 98.95 and 101.76% with a relative standard deviation in the range of 0.0047–3.92% for serum samples. The closeness of the results to 100% showed the fairly good accuracy of the method.
4.1.5. Accuracy and precision study. For precision computation, the assays were performed in triplicates within the same day and on three different days to determine the repeatability of the method and intermediate precision, respectively. The average percentage relative standard deviation (% RSD) values were calculated and found to be ≤0.0047–1.57% and ≤0.0047–3.92% for intra-day and inter-day precisions, respectively, for the serum samples, indicating the high precision of the proposed method. The inter-day values indicate high precision of the method. Accuracy was assessed as percentage relative error (%RE) between the measured mean concentrations and the known β-hCG concentrations and was determined at each concentration. % RE results are summarized in Table 2 having values ≤0.009–1.76% for intra-day and ≤0.007–1% for inter-day for the serum samples, demonstrating the high accuracy of the proposed method.
5. Conclusion
The N/S-doped CQDs exhibited characteristic peaks, which are significantly enhanced in the presence of β-hCG at λex/λem = 288/345 nm owing to the energy transfer from the N/S-doped CQDs. The proposed method was found to be simple, reliable, and applicable for the determination of β-hCG with a wide range of analysis and high accuracy and precision.
Conflicts of interest
The authors declare no conflict of interest.
Acknowledgements
Authors extend their appreciation to the Deputyship for Research & Innovation, Ministry of Education in Saudi Arabia for funding this research work through the project number “NBU-FFR-2023-0020”
References
- Y. Wang and A. Hu, Carbon quantum dots: synthesis, properties and applications, J. Mater. Chem. C, 2014, 2, 6921–6939 RSC
. - A. Sharma and J. Das, Small molecules derived carbon dots: synthesis and applications in sensing, catalysis, imaging, and biomedicine, J. Nanobiotechnol., 2019, 17, 92 CrossRef PubMed
. - M. Nasrollahzadeh, M. Sajjadi, S. Mohammad Sajadi and Z. Issaabadi, Chapter 5 – Green Nanotechnology, Interface Science and Technology, Elsevier, 2019, vol. 28, pp. 145–198 Search PubMed
. - J. Liu, R. Li and B. Yang, Carbon Dots: A New Type of Carbon-Based Nanomaterial with Wide Applications, ACS Cent. Sci., 2020, 6, 2179–2195 CrossRef CAS PubMed
. - J. V. Klavins, Advances in biological markers for cancer, Ann. Clin. Lab. Sci., 1983, 13, 275 CAS
. - M. T. Sheaff, J. E. Martin, D. F. Badenoch and S. I. Baithun, β-hCG as a prognostic marker in adenocarcinoma of the prostate, J. Clin. Pathol., 1996, 49, 329–332 CrossRef CAS PubMed
. - U. Otite, S. Baithun, F. Chinegwundoh, V. H. Nargund and R. K. Iles, Detection of Human Chorionic Gonadotrophin in Serum or Urine of Prostate Cancer Patients Is of No Clinical Significance, Tumor Biol., 2006, 27(4), 181–186 CrossRef CAS PubMed
. - S. K. Sengodan, R. Nadhan, R. S. Nair, S. K. Hemalatha, V. Somasundaram, R. R. Sushama, A. Rajan, N. R. Latha, G. R. Varghese, R. Thankappan, J. M. Kumar, A. Chil, T. V. Anilkumar and P. Srinivas, BRCA1 regulation on β-hCG: a mechanism for tumorigenicity in BRCA1 defective breast cancer, Oncogenesis, 2017, 6, e376 CrossRef CAS PubMed
. - S. P. Georgia Chris, L. P. Ravandhu and B. K. Haseena, International Conference on Signal Processing Image Processing & Pattern Recognition (ICSIPR), 2013, vol. 75 Search PubMed
. - M. Daja, M. Aghmesheh, K. Ow, P. Rohde, K. D. Barrow and P. Russell, Beta-human chrionic gonadrtropin in semen: a marker for early detection of prostate cancer?, Mol. Urol., 2000, 4(4), 421–427 CAS
. - P. N. Span, C. M. G. Thomas, J. J. Heuvel, R. R. Bosch, J. A. Schalken, L. vd Locht, E. J. B. M. Mensink and C. G. J. Sweep, Analysis of expression of chorionic gonadotrophin transcripts in prostate cancer by quantitative Taqman and a modified molecular beacon RT-PCR, J. Endocrinol., 2002, 172(3), 489–495 CAS
. - J. Malakova, P. Pavek, I. Svecova, P. Zivny and V. Palicka, J. Chromatogr. B, 2009, 877, 3226 CrossRef CAS PubMed
. - O. Stern and M. Volmer, Über die abklingzeit der fluoreszenz, Z. Med. Phys., 1919, 20, 183–188 CAS
. - R.-Y. Xu, X.-F. Zhu, Y. Yang and P. Ye, High-sensitive cardiac troponin T, J. Geriatr. Cardiol., 2013, 10, 102–109 CAS
. - E. M. Antman, Decision making with cardiac troponin tests, N. Engl. J. Med., 2002, 346, 2079–2082 CrossRef PubMed
. - S. H. Jin, D. H. Kim, G. H. Jun, S. H. Hong and S. Jeon, Tuning the photoluminescence of graphene quantum dots through the charge transfer effect of functional groups, ACS Nano., 2013, 7, 1239–1245 CrossRef CAS PubMed
. - G. C. Semeraro, C. M. Cipolla and D. M. Cardinale, Role of Cardiac Biomarkers in Cancer Patients, Cancers, 2021, 13, 5426 CrossRef CAS PubMed
. - Y. Liu, F. He, F. Gao, Y. Su, H. Wei and Y. Zhang, Nitrogen-doped, carbon-rich, highly photoluminescent carbon dots from ammonium citrate, Nanoscale, 2014, 6, 1890–1895 RSC
. - K. Thygesen, J. Mair and H. Katus, et al., Recommendations for the use of cardiac troponin measurement in acute cardiac care, Eur. Heart J., 2010, 31, 2197–2204 CrossRef CAS PubMed
. - A. H. Wu, R. Valdes Jr and F. S. Apple, et al., Cardiac troponin-T immunoassay for diagnosis of acute myocardial infarction, Clin. Chem., 1994, 40, 900–907 CrossRef CAS
. - M. Muller-Bardorff, K. Hallermayer and A. Schroder, et al., Improved troponin T ELISA specific for cardiac troponin T isoform: assay development and analytical and clinical validation, Clin. Chem., 1997, 43, 458–466 CrossRef CAS
. - K. Hallermayer, D. Klenner and R. Vogel, Use of recombinant human cardiac Troponin T for standardization of third generation Troponin T methods, Scand. J. Clin. Lab. Invest., Suppl., 1999, 230, 128–131 CrossRef CAS PubMed
. - E. Giannitsis, K. Kurz and K. Hallermayer, et al., Analytical validation of a high-sensitivity cardiac troponin T assay, Clin. Chem., 2010, 56, 254–261 CrossRef CAS PubMed
. - X. Wang, L. Cao, S. T. Yang, F. Lu, M. J. Meziani, L. Tian, K. W. Sun, M. A. Bloodgood and Y. P. Sun, Bandgap-like strong fluorescence in functionalized carbon nanoparticles, Angew. Chem., Int. Ed., 2010, 49, 5310–5314 CrossRef CAS PubMed
. - G. Eda, Y. Y. Lin, C. Mattevi, H. Yamaguchi, H. A. Chen, I. S. Chen, C. W. Chen and M. Chhowalla, Blue photoluminescence from chemically derived graphene oxide, Adv. Mater., 2010, 22, 505–509 CrossRef CAS PubMed
. - D. Sun, R. Ban, P.-H. Zhang, G.-H. Wu, J.-R. Zhang and J.-J. Zhu, Hair fiber as a precursor for synthesizing of sulfur-and nitrogen-codoped carbon dots with tunable luminescence properties, Carbon, 2013, 64, 424–434 CrossRef CAS
. - M. Jorns and D. Pappas, A review of fluorescent carbon dots, their synthesis, physical and chemical characteristics, and applications, Nanomaterials, 2021, 11(No), 1448 CrossRef CAS PubMed
. - J. Xu, Q. Liang, Z. Li, V. Y. Osipov, Y. Lin, B. Ge, Q. Xu, J. Zhu and H. Bi, Rational Synthesis of Solid-State Ultraviolet B Emitting Carbon Dots via Acetic Acid-Promoted Fractions of sp3 Bonding Strategy, Adv. Mater., 2022, 34, 2200011 CrossRef CAS PubMed
. - S. Yang, L. Zhi, K. Tang, X. Feng, J. Maier and K. Müllen, Efficient synthesis of heteroatom (N or S)-doped graphene based on ultrathin graphene oxide-porous silica sheets for oxygen reduction reactions, Adv. Funct. Mater., 2012, 22, 3634–3640 CrossRef CAS
. - Z. Yang, Z. Yao, G. Li, G. Fang, H. Nie, Z. Liu, X. Zhou, X. Chen and S. Huang, Sulfur-Doped Graphene as an Efficient Metal free Cathode Catalyst for Oxygen Reduction, ACS Nano, 2012, 6, 205–211 CrossRef CAS PubMed
. - Y. Li, Y. Hu, Y. Zhao, G. Shi, L. Deng, Y. Hou and L. Qu, An electrochemical avenue to green-luminescent graphene quantum dots as potential electron-acceptors for photovoltaics, Adv. Mater., 2011, 23, 776–780 CrossRef CAS PubMed
. - J. Zhou, Y. Yang and C.-y. Zhang, A low-temperature solid-phase method to synthesize highly fluorescent carbon nitride dots with tunable emission, Chem. Commun., 2013, 49, 8605–8607 RSC
. - Y. Dong, H. Pang, H. B. Yang, C. Guo, J. Shao, Y. Chi, C. M. Li and T. Yu, Carbon-based dots co-doped with nitrogen and sulfur for high quantum yield and excitation-independent emission, Angew. Chem., Int. Ed., 2013, 52, 7800–7804 CrossRef CAS PubMed
. - W. Horrocks and D. R. Sudnick, J. Am. Chem. Soc., 1979, 101, 334 CrossRef CAS
. - J. C. G. Bünzli and G. R. Choppin, Lanthanide probes in life, chemical and earth sciences: theory and practise, Elsevier, Amsterdam, 1989 Search PubMed
. - ICH, Validation of Analytical Procedures: Text and Methodology, Q2(R1), Complementary Guideline on Methodology, ICH, London, 2005 Search PubMed
. - M. S. Attia, M. H. Khalil, M. S. A. Abdel-Mottaleb, M. B. Lukyanova, Yu. A. Alekseenko and B. Lukyanov, Effect of Complexation with Lanthanide Metal Ions on the Photochromism of (1,3,3-Trimethyl-5-Hydroxy-6-FormylIndoline-Spiro2,2-[2H] chromene) in Different Media, Int. J. Photoenergy, 2006, 1–9 CrossRef
. - M. S. Attia, M. H. Khalil, M. S. A. Abdel-Mottaleb, M. B. Lukyanova, Y. A. Alekseenko and B. Lukyanov, Int. J. Photoenergy, 2006, 1–9 CrossRef
. - M. S. Attia, A. O. Youssef and A. A. Essawy, Anal. Methods, 2012, 4, 2323–2328 RSC
. - M. S. Attia, W. H. Mahmoud, A. O. Youssef and M. S. Mostafa, J. Fluoresc., 2011, 21, 2229–2235 CrossRef CAS PubMed
. - M. S. Attia, M. N. Ramsis, L. H. Khalil and S. G. Hashem, J. Fluoresc., 2012, 22, 779–788 CrossRef CAS PubMed
. - M. S. Attia, W. H. Mahmoud, M. N. Ramsis, L. H. Khalil, A. M. Othman, S. G. Hashem and M. S. Mostafa, J. Fluoresc., 2011, 21, 1739–1748 CrossRef CAS PubMed
. - M. S. Attia, A. M. Othman, E. Elraghi and H. Y. Aboul-Enein, J. Fluoresc., 2011, 21, 739–745 CrossRef CAS PubMed
. - A. A. Elabd and M. S. Attia, J. Lumin., 2016, 169, 313–318 CrossRef CAS
. - M. S. Attia, E. Bakir, A. A. Abdel-Aziz and M. S. A. Abdel-Mottaleb, Talanta, 2011, 84, 27–33 CrossRef CAS PubMed
. - FDA Guidance for Industry – Analytical Procedures and Method Validation, Chemistry, Manufacturing, and Controls Documentation, Center for Drug Evaluation and Re- search (CDER) and Center for Biologics Evaluation and Research (CBER), 2000 Search PubMed
. - USP 31, General Tests, Chapter 621 – Chromatography System Suitability, United States Pharmacopeial Convention (USP), Rockville, MD, 2009 Search PubMed
. - C. C. Chan, et al., Analytical Method Validation and Instrument Performance Veriflcation, John Wiley & Sons (Wiley Interscience), Hoboken, NJ, 2004 Search PubMed
. - M. S. Attia, S. A. Elsaadany, K. A. Ahmed, M. M. El-Molla and M. S. A. Abdel-Mottaleb, J. Fluoresc., 2015, 25, 119–125 CrossRef CAS PubMed
. - S. G. Hashem, M. M. Elsaady, H. G. Afify, M. El-Kemary and M. S. Attia, Talanta, 2019, 199, 89–96 CrossRef CAS PubMed
. - M. S. A. Abdel-Mottaleb, M. Saif, M. S. Attia, M. M. Abo-Aly and S. N. Mobarez, Photochem. Photobiol. Sci., 2018, 17, 221–230 CrossRef CAS PubMed
. - W. E. Omer, M. A. El-Kemary, M. M. Elsaady, A. A. Gouda and M. S. Attia, ACS Omega, 2020, 5, 5629–5637 CrossRef CAS PubMed
. - L. M. Abdullah, M. S. Attia and M. S. A. Abdel-Mottaleb, Egypt. J. Chem., 2019, 62, 247–255 Search PubMed
. - M. S. Attia, A. O. Youssef, A.-S. S. H. Elgazwy, S. M. Agami and S. I. Elewa, J. Fluoresc., 2014, 24, 759–765 CrossRef CAS PubMed
. - M. M. Abd-Elzaher, M. A. Ahmed, A. B. Farag, M. S. Attia, A. O. Youssef and S. M. Sheta, Sens. Lett., 2017, 15(12), 977–981 CrossRef
. - V. Bressi, A. Ferlazzo, D. Iannazzo and C. Espro, Nanomaterials, 2021, 11(5), 1120 CrossRef CAS PubMed
. - M. Díaz-Álvarez and A. Martín-Esteban, Biosensors, 2021, 11(3), 79 CrossRef PubMed
. - K. Murugan, V. Kumar Jothi, A. Rajaram and A. Natarajan, ACS Omega, 2022, 7(1), 1368–1379 CrossRef CAS PubMed
.
|
This journal is © The Royal Society of Chemistry 2023 |