DOI:
10.1039/D3RA01505J
(Review Article)
RSC Adv., 2023,
13, 18404-18442
Experimental and computational study of metal oxide nanoparticles for the photocatalytic degradation of organic pollutants: a review
Received
7th March 2023
, Accepted 31st May 2023
First published on 19th June 2023
Abstract
Photocatalysis is a more proficient technique that involves the breakdown or decomposition of different organic contaminants, various dyes, and harmful viruses and fungi using UV or visible light solar spectrum. Metal oxides are considered promising candidate photocatalysts owing to their low cost, efficiency, simple fabricating method, sufficient availability, and environment-friendliness for photocatalytic applications. Among metal oxides, TiO2 is the most studied photocatalyst and is highly applied in wastewater treatment and hydrogen production. However, TiO2 is relatively active only under ultraviolet light due to its wide bandgap, which limits its applicability because the production of ultraviolet is expensive. At present, the discovery of a photocatalyst of suitable bandgap with visible light or modification of the existing photocatalyst is becoming very attractive for photocatalysis technology. However, the major drawbacks of photocatalysts are the high recombination rate of photogenerated electron–hole pairs, the ultraviolet light activity limitations, and low surface coverage. In this review, the most commonly used synthesis method for metal oxide nanoparticles, photocatalytic applications of metal oxides, and applications and toxicity of different dyes are comprehensively highlighted. In addition, the challenges in the photocatalytic applications of metal oxides, strategies to suppress these challenges, and metal oxide studied by density functional theory for photocatalytic applications are described in detail.
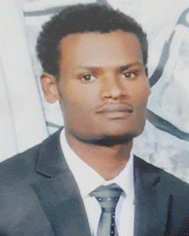 Fikadu Takele Geldasa | Fikadu Takele was born in Welega, Ethiopia, in 1993. He received his BSc degree in Applied Physics from Hawassa University in 2015 and his MSc degree in Solid State Physics from Dilla University, in 2017. Currently he is a PhD student at Adama Science and Technology University under the supervision of Dr Fekadu Gashaw, Dr Mesfin Abayneh and Dr Megersa Wodajo. His research interest is on energy storage electrode materials specifically on lithium ion batteries. |
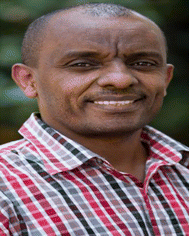 Mesfin Abayneh Kebede | Mesfin Kebede obtained his PhD in Metallurgical Engineering/Applied Physics from Inha University, South Korea. He is currently a Principal Research Scientist at Energy Centre of Council for Scientific and Industrial Research (CSIR), South Africa. In addition, he holds Honorary Associate Professor position at University of the Witwatersrand, South Africa. His current research mainly focuses on the development and characterization of electrode materials for energy storage and fabrication of coin cell and pouch cell for full cells. Improving the electrochemical performance of electrode materials for energy storage systems such as lithium-ion batteries, sodium-ion batteries, and supercapacitors. |
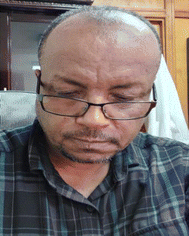 Megersa Wodajo Shura | Megersa Wodajo Shura was earned his BSc degree in Physics at Asmara University, Eritrea in 1990. After serving at different institutions for a while, he joined Addis Ababa University for his MSc Study in 1997. Then, he joined Adama Teachers College until he moved to Adama Science and Technology University in September 2006. Then, he joined Nelson Mandela University, South Africa for his PhD study in March 2009. Upon completion of his PhD, he returned to ASTU and served as Assistance and Associate Professor of Physics. His research interest is dealing with the various optoelectrical properties of inorganic semiconductors. |
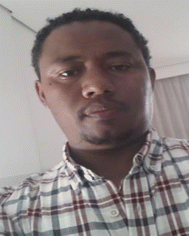 Fekadu Gashaw Hone | Dr Fekadu Gashaw is an Associate Professor of Physics and currently working at Addis Ababa University department of Physics as Researcher and lecturer. He has obtained his first degree in Physics from Debub University in 2001 and his MSc degree from AAU in Laser Spectroscopy (2006). He got his PhD degree from KNUST (Ghana) (2015). Fekadu was also a Postdoctoral researcher at the University of the Free State, (South Africa) from 2016 to 2020. His research mainly focuses on the development and characterization of novel nanomaterials for various applications such as Energy storage and conversion, Gas sensors and Antimicrobial activities. Currently, he is supervising more than ten PhD students. |
1 Introduction
Nowadays, environmental pollution and the energy crisis are the two main concerns of our world. With urban growth and rapid industrialization, our globe is in an era of prosperity and wealth. The increasing world population and continuous development of urban, industrial, and agricultural processes cause an increase in pollutants in air and water sources. Human activity due to population growth, such as household activity and the development of industrial and agricultural byproducts, leads to mostly organic contaminants. If these organic contaminants are biodegradable, it can be easily treated by biological processes. However, pollutants from industries, such as textiles, pharmaceuticals, and agriculture, often contain low biodegradability. In addition to these, chemicals, microbes, metal plating, pulp, refineries, and food processing are waste-generating businesses that discharge many wastes, contaminating terrestrial land, atmosphere, and water sources.1 Byproducts from textile industries pollute the environment, especially the water resources, due to the random use of various types of dyes and discharge them to the water sources. Thus, water sources polluted by textile industries contain a variety of organic dyes and chemicals; the chemical composition of these effluents is a major environmental problem.2 On the other hand, antibiotics are used to treat animal and human diseases. The consumption of these antibiotics leads to their release into the environment after incomplete metabolism. The entering of these compounds into the environment through wastewater from the pharmaceutical industry, human and animal waste deposits, and hospitals causes environmental pollution and is difficult to remove using traditional treatments.3 Agricultural waste products also cause environmental pollution including water sources. For the development of modern agriculture, pesticides are used. Pesticides and agro wastewater are risks to human health and the aquatic ecosystem.4
Moreover, synthetic dyes that are used in the textile industries and agriculture are physically and chemically stable compounds, which enormously affect the environment. Some of these organic pollutants are persistent and identified at a significant level in surface and groundwater, soil, and wastewater effluents.5 Different conventional techniques such as biological treatment, anaerobic, electrochemical, aerobic, oxidation, adsorption, flotation, reduction, precipitation, and flocculation have been used in wastewater treatment.6 However, these techniques cannot fully degrade the organic pollutants. On the other hand, we can classify the developed methods to eliminate or reduce organic pollutants into four types:7–9 (i) physicochemical, which includes membrane bioreactor, adsorption, sedimentation, coagulation, filtration, and flocculation; (ii) chemical treatment, which includes chemical reduction, electrochemistry, advanced oxidation process (AOP), ozone oxidation, enzymes, and photocatalytic degradation; (iii) bioremediation, which includes biological oxidation, and activated sludge; (iv) the combined process. Physicochemical treatment has advantages such as low cost, high treatment efficiency, and easy operation, but its degradation ability is low. Bioremediation can remove organic pollutants from large areas at a very low cost, but it has low efficiency. Chemical treatment has an incredible efficiency to mineralize organic pollutants, but it can cause secondary pollution.
Over the previous decade, researchers around the world made great efforts to develop a more powerful, newer, and promising technique called advanced oxidation process (AOP).10 The AOP is the most interesting method to remove organic pollutants in wastewater.11 This method is an alternative to traditional techniques such as adsorption and coagulation because the AOP process mineralizes the organic pollutants rather than moving from place to place. Thus, photocatalysis is a promising AOP and is a green method to decompose organic pollutants nonselectively and quickly from wastewater using semiconductor metal oxides as photocatalyst materials,12 meaning that for overcoming environmental pollution, the degradation of harmful and toxic contaminant organic compounds using semiconducting photocatalysts is of great significance.13 A semiconducting photocatalyst is a material that is used as a catalyst in photocatalytic processes. Thus, a catalyst is a material that initiates and enhances the rate of a chemical reaction, but the material that enhances the rate of the chemical reaction remains unchanged at the end of the reaction.14 In other words, a semiconducting photocatalyst is a material that is capable of harvesting solar light, generating electron–hole pairs that enable transformations of participants of reaction and reproduce its chemical composition after each cycle of such interactions.15 The catalytic properties of materials under the illumination of either UV or visible light is called photocatalytic activity, and the acceleration of the rate of a chemical reaction by activating a catalyst by light (UV or visible light) is known as photocatalysis.14 Photocatalysis is a more proficient technique that involves the breakdown or decomposition of different organic pollutants, various dyes, and harmful viruses and fungi using UV or visible light solar spectrum.16 Photocatalysis was discovered in 1972 during the oil crisis in 1973 and the energy crisis in 1979 for the production of hydrogen production by water splitting.17 Nowadays, photocatalytic oxidation processes through semiconductor metal oxide nanoparticles are globally accepted and are the most advanced techniques.18
After the discovery of photocatalysis technology, researchers have been attracted to understanding the fundamental mechanisms of photocatalysis and improving the photocatalytic properties of the discovered photocatalysts. The photocatalysis technique depends on the reaction between powerful oxidizing and reduction agents (holes and electrons) generated through the illumination of UV or visible solar spectrum on the surface of photocatalyst materials and harmful organic pollutants.19 Overall, photocatalysis is a process in which the electron–hole pair is generated through the illumination of sunlight to the photocatalyst materials. When the photocatalyst material is exposed to light with energy equal to or greater than the photocatalyst bandgap, the electrons in the valence band (VB) are stimulated and excited to the highest-level conduction band (CB), leaving behind holes in the VB. Generally, the photocatalysis process follows five complementary steps including (i) light harvesting, (ii) electrons–holes generation under the irradiation of light, (iii) charge carrier's separation and moving to the photocatalyst surface, (iv) oxidation and reduction of charge carriers with reactants, and (v) charge carrier recombination on the catalyst surface. The photoinduced electrons and holes reacted with oxygen (O2), water (H2O), and hydroxyl groups to generate reactive oxygen species (ROS) such as hydroxyl radicals (˙OH) and superoxide radical anions (O2˙−) with strong oxidation abilities. These ROS are the foremost accountable species for the degradation of persistent organic pollutants in wastewater. These phenomena can be utilized to solve the main problems of our world today in the energy crisis by the process of water splitting to generate hydrogen gas, which is used as fuel, and environmental pollution through the degradation of organic pollutants in wastewater.20 Thus, the application of photocatalysis is not limited to the degradation of organic pollutants, it is also applied in bacterial disinfectants and for the removal of antibiotics from water. It has great potential in the evolution of hydrogen (H2) by water splitting as a green energy fuel in the production of hydrocarbon fuel by reducing carbon dioxide (CO2), and the potential of changing NO and NO2 into nitrate, which is used as a fertilizer to stimulate the growth of plants.21–23
According to researchers' investigations, the nanosized particles show an essential photocatalysis process due to their unique chemical and physical properties, high surface-to-volume ratio, fast dissolution, strong sorption, and high reactivity with respect to their bulk of the same compositions.24,25 Among these nanosized materials, metal oxide nanoparticles (MONPs) are used as prominent photocatalysts. MONPs have a wide range of applications including environmental remediation, energy storage, electrochemistry, sensors, lubrication, coatings, and other various areas of nanotechnology.1 Various semiconducting metal oxide photocatalyst materials have been developed to date. Semiconducting metal oxides such as TiO2, ZnO, SnO2, Cu2O, Fe2O3, SrTiO3, zinc germanium oxide, bismuth oxides, copper oxides, and WO3 have got much attention due to their ability in the degradation of various organic pollutants.13,26 Their effective advantages are attributed to their large surface area, small size, optical and chemical properties, and electrical conductivity properties.27 However, there are some shortcomings of semiconductor metal oxide photocatalysts such as a small range of the solar spectrum absorption abilities, wide bandgap, and fast recombination rates of photogenerated electrons and holes, which reduce their photocatalytic activities.20,28 A photocatalyst materials with wide bandgap value requires more energy to be absorbed for charge carrier separation. In addition, the photogenerated electrons and holes could recombine without forming oxidation and reduction, which reduces the photocatalytic activities of these photocatalysts. The fast recombination rate results in a lower number of photogenerated ROS that are available on surface reaction sites to carry out the photodegradation process. Hence, the overall photocatalytic activity is significantly reduced. Many researchers have been working on the modification of photocatalysts or engineering new photocatalysts to address these concerns. The introduction of a heteroatom doping agent into the bulk semiconductor metal oxides modifies the photocatalytic activities of semiconductor metal oxides. Thus, it provides a large dipole moment to change the electron transfer kinetic, and more electrons can be transferred from the valence band to the conduction band of the photocatalyst, hence narrowing the bandgap value.28,29 The low bandgap value indicates better absorption ability in visible light or natural sunlight. Good photocatalyst materials will now be able to reduce the recombination of photogenerated electron–hole pairs and are able to absorb the visible light range of the solar spectrum. In addition, materials used as photocatalysts should be earth-abundant and cost-effective.
A lead monoxide (PbO) nanoparticle is among the MONPs. PbO and Pb-based oxide material has attracted the researchers' focus due to its exceptional mechanical, optical, and electrical properties.30 The photocatalyst activity of PbO nanoparticles have been investigated for the degradation of organic pollutants.31–33 However, for the photocatalytic applications of PbO, there are only very few reports, but the coupled PbO with other semiconductors has been widely reported. Thus, the combination of PbO with different metal oxide semiconductors has advantages such as shifting the ultraviolet activity of materials to visible light active by controlling the bandgap and modifying the structure and morphology of the newly formed composite photocatalyst.
Nanotechnology includes both research and engineering of new materials at the nanoscale level. Different synthetic techniques have been used for the synthesis of metal oxide nanoparticles. In this review, the synthesis method for metal oxide nanoparticles, metal oxide studied by density functional theory for photocatalytic applications, applications, and toxicity of different dyes are highlighted. In addition, the photocatalytic applications of metal oxides, challenges in the photocatalytic applications of metal oxides, and strategies to suppress these challenges in metal oxide photocatalysts are described in detail. Previously, many review articles on the photocatalytic applications of metal oxide have been published so far. However, these articles are mainly focused on either applications or challenges and strategies for solving challenges in metal oxide photocatalysts. In addition to this, these articles did not include metal oxide studied both by experimental and DFT methods simultaneously. Therefore, this review has significant advantages in giving way for understanding and studying metal oxide nanoparticles experimentally and computationally. Moreover, the reader can understand easily the drawbacks of metal oxide photocatalysts and the strategically taken action until the present to tackle the abovementioned drawbacks. Therefore, depending on the identified drawbacks, researchers can develop additional strategies to overcome the mentioned drawbacks or design new photocatalysts.
2 Metal oxide nanoparticles
Metal oxide nanoparticles (MONPs) are semiconducting materials that have tremendous applications in different areas of science and technology. Thus, nanosized metal oxides have more imperative physicochemical properties than their bulk of the same composition and have received large attention due to their electronic, catalytic, and magnetic properties.34 These materials have unique properties such as cold welding, nonlinear, superparamagnetic behavior, higher ductility at elevated temperatures than coarse-grained ceramics, unique catalytic properties, sensitivity, and selective activity that make them indispensable tools in modern nanotechnology.35,36 In addition, they exhibit fast diffusivity and unique adsorption properties, which makes them different from their bulk materials of the same compositions.37 Low density, high surface-to-volume ratio, and quantum properties of nanoparticles are the enchanted properties of nanoparticles.38,39 More importantly, MONPs have potential applications that attract researchers from the fields of medicine, materials chemistry, materials science, physics, agriculture, electronics, information technology, energy, biomedical, catalysis, sensor, and environment.40,41 Specifically, MONPs have been attracted for their development of new and effective strategies in environmental remediation and energy production. Among these MONPs, CuO, ZnO, Al2O3, Fe2O3, ZrO2, MgO, AgO, SnO2, TiO2, CeO2, PbO, etc., are the most attractive one.35
As nanotechnology includes the search for and manufacturing nonexistent materials at the nanoscale level, several synthetic techniques and strategies were successfully used in the development of nanotechnology. In general, there are two types of approaches to the synthesis and fabrication of nanomaterials.42,43 They are (i) bottom-up approach: this approach includes the miniaturization of materials components up to the atomic level with further self-assembly process (physical forces operating at the nanoscale are used to combine basic units into larger stable structures), leading to the formation of nanostructures. This approach organizes atomic or molecular components to form clusters and then to grow into different morphologies. Quantum dots or nanoparticles formed from colloidal dispersion are examples of a bottom-up approach; (ii) the top-down approach starts from bulk structures to reduce to nanostructured materials.44
Nanotechnology techniques can be classified as wet, dry, and computational engineering, as shown in Fig. 1. Wet nanotechnology handles the process, which takes place in a wet environment mostly using living organisms and different components present in the tissue, organs, and cells in living systems. Dry engineering deals with the engineering of different technological applications and it includes (i) electronics, optoelectronic, and photonics; (ii) information processing, storage, and transmission; (iii) food packing and storage; (iv) pigments, protective glasses, textiles; and (v) sensors and energy devices. Computational engineering deals with the development and use of computer-based quantum and molecular design, modeling, and simulation of properties of systems relevant to nanotechnology.35
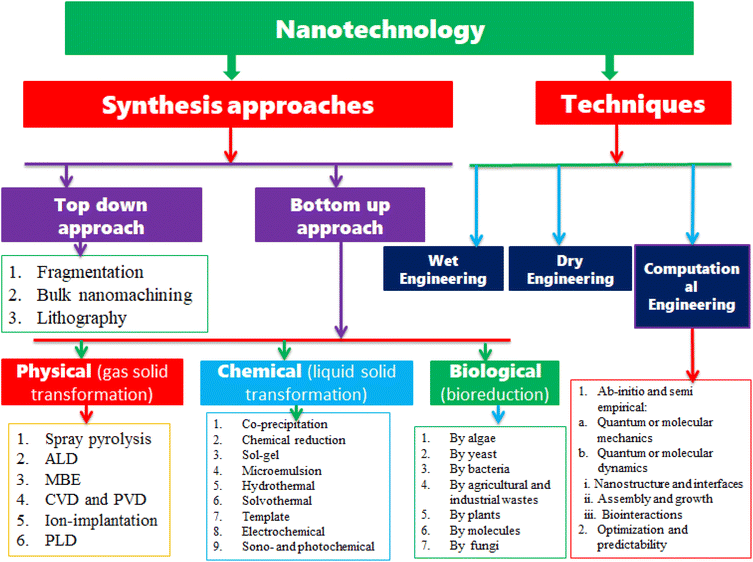 |
| Fig. 1 The major synthesis approaches of MONPs and techniques used in nanotechnology. | |
2.1 Synthesis of metal oxide nanoparticles
The strategies for the synthesis of MONPs can be classified into top-down and bottom-up approaches, as shown in Fig. 1. The top-down approach is reducing the large particle size either macro or microparticles to nanosized, whereas the bottom-up approach begins atomic growth up to nanosized particles.50 As presented in Fig. 1, the top-down approach includes fragmentation, bulk nanomachining, and lithography, whereas the bottom-up approach can be classified into physical, chemical, and biological methods. The physical methods are the process of gas transformation to solids. The physical methods under the bottom-up approaches for the synthesis of nanoparticles include spray pyrolysis, atomic layer deposition (ALD), molecular beam epitaxy (MBE), chemical vapor deposition (CVD), physical and pulse vapor deposition (PVD), and ion implantation, and the biological method is a green synthesis method of nanoparticles by extracting from algae, yeast, bacteria, agricultural and industrial wastes, plants, molecules, fungi, etc. The chemical methods for nanoparticle synthesis include coprecipitations, chemical reduction, sol–gel, microemulsion, hydro and solvothermal, template, electrochemical, and sono and photochemical. In this review, we only reviewed some of the chemical synthetic methods that are widely used for the synthesis of metal oxide nanoparticles.
2.1.1 Sol–gel process. The sol–gel process is a simple, ecofriendly, and inexpensive method that enables to control the high purity and homogeneity of the final metal oxide nanoparticles product and also to control the size and growth of particles and supports the addition of large concentrations of doping agents.51 The sol–gel process is used to prepare a high-quality metal oxide nanoparticle and metal oxide nanocomposite, which is done by the hydrolysis process of metal alkoxides dissolved in alcohol or water. From this, the corresponding hydroxide has resulted. Thereafter, by the condensation of the formed hydroxide, a metal hydroxide polymer is formed in a densely packed gel form. To obtain the metal oxide powder of the targeted particle size, the formed gel is heated and dried. Generally, the sol–gel process steps are (i) hydrolysis, (ii) polycondensation, (iii) aging, (iv) drying, and (v) thermal decomposition.45,52 The finally heated and dried have the possibility to give us metal oxide in a form of bulk materials, nanoparticles, or oxygen vacancy based on the heat-treatment process done.28 A variety of metal oxide nanoparticles such as ZnO,53 TiO2,54 MgO,55 ZrO2,56 CuO,57 and Nb2O5/SiO2 (ref. 58) were synthesized by the sol–gel process. For example, Patel et al.59 reported the synthesized CuO and ZnO by the sol–gel process using copper(II) nitrate and zinc nitrate as starting materials, respectively. The advantages and disadvantages of the sol–gel method is summarized in Table 1.
Table 1 Advantages and disadvantages of the sol–gel synthesis method
Synthesis methods |
Advantages |
Disadvantages |
Ref. |
Sol–gel |
• Easy to perform in the laboratory |
• Use of organic solution that can cause toxicity |
45–47 |
• Inexpensive and high yield |
• Sensitivity to moisture |
• Synthesis is at low temperature |
• Includes many steps and the process takes a long time |
• Produce high-quality materials |
• Dimensions and volume changes during different steps |
• High scale homogeneity and purity |
• Product contains sol–gel matrix components |
• Doping of different elements can be controlled |
• Metal alkoxides are almost expensive |
• Atomic level mixing components (precursors) |
• Residual carbon and hydroxyl groups |
• High purity and proper stoichiometry can be achieved |
|
• Desired shape and length can be obtained |
|
• Precise control of size and structure |
|
• Materials can be obtained in bulk, thin film, or nanopowders |
|
• Can produce coating to provide protection against corrosion |
|
• Homogenous and high adhesion products of thin films |
|
• Synthesis of uniform compounds in the form of composite oxides |
|
2.1.2 Coprecipitation. In the synthesis of metal oxide nanoparticles using the coprecipitation process, the reaction takes place at ambient temperature. In this method, a solution of metal salt and a precipitating media produces a precipitated oxo-hydroxide in a solvent. KOH, NaOH, and ammonia solutions are commonly used as precipitating media. Thus, the alkaline nature and pH of the solution highly influence the properties and morphologies of metal oxide nanoparticles. To come up with the desired morphology and properties of metal oxide nanoparticles, the amount, rate, and mode of alkali solution added to the reaction mixture must be added. By adding surfactants, it is possible to narrow the size of the produced nanoparticles as surfactants are very useful and crucial in the tailoring of surface characteristics of metal oxide nanoparticles. This method is the highly used synthesis method for metal oxide nanoparticles due to its simplicity, low cost, scale-up, and low-temperature reaction rates. It is also possible to control the particle size and crystal structure by varying media pH and, at times, large amounts of nanoparticles can be prepared using the coprecipitation method.62 Various metal oxide nanoparticles such as MnO2,63 ZnO,64 MgO,65 BiVO4,66 Ni-doped SnO2,67 N- and Fe-doped TiO2,68 CuO,69 Cu-doped ZnO,70 PbWO4,71 etc., were synthesized using the coprecipitation method. Kotresh et al.64 synthesized ZnO nanoparticles via the coprecipitation process at 50 °C and 70 °C reaction temperatures using sodium hydroxide as the precipitating media and zinc nitrate hexahydrate as the Zn2+ precursor. Buraso et al.72 also used the same synthesizing approach for the preparation of TiO2 using titanium(IV) isopropoxide as a precursor. Rangel et al.73 used the coprecipitation method to synthesize CuO nanoparticles using copper chloride and copper sulfate as a precursor. Coprecipitation method has advantages and disadvantages in metal oxide synthesis, as given in Table 2.
Table 2 Advantages and disadvantages of the coprecipitation synthesis method
Synthesis methods |
Advantages |
Disadvantages |
Ref. |
Coprecipitation |
• Low cost and environment-friendly |
• Wide particle size distribution |
46, 48 and 49 |
• Simple synthesis |
• Wide particle size distribution, poor crystallinity, and aggregation |
• High product |
• Surface oxidation |
• Simple and efficient |
• Poor reproducibility |
• Fast reaction |
• Limited by the boiling point of water |
• Well dispersed in polar solution |
|
• Easily scale up the production |
|
2.1.3 Hydrothermal synthesis. Hydrothermal synthesis is a solution reaction-based method, and the process is called hydrothermal because water is used as a solvent, whereas in solvothermal synthesis, organic solvents are used. In this method, a wide range of temperature and pressure (above ambient temperature and pressure) is used for the formation of nanoparticles. By controlling the reaction pressure, the morphology of the prepared nanoparticles can be controlled either by decreasing or increasing the pressure conditions. In this method, the aqueous mixture is heated in a sealed stainless-steel autoclave above the water boiling point and increasing pressure in the reaction above the atmospheric pressure. This method has significant advantages over other synthesis approaches. It can generate nanoparticles that cannot be prepared at low temperatures or room temperature, and it can also produce nanoparticles if the materials have a high vapor pressure.74 During the reaction in an autoclave, the particles can be agglomerated. To prevent the agglomeration of the particles, it is necessary to use a suitable stabilizer or capping agent. Several metal oxide nanoparticles such as SnO2,75 TiO2,76 Fe2O3,77 NiO,78 and BiVO4 (ref. 79) were prepared using the hydrothermal synthesis process. Bade et al.80 prepared copper oxide nanoparticles using copper sulfate pentahydrate as a precursor and NaOH as the stabilizing agent via hydrothermal synthesis. Suthakaran et al.81 reported high-quality SnO2 nanoparticles synthesized by hydrothermal synthesis, and they studied the photocatalytic activity of these nanoparticles by degrading methyl violet dye. The advantage and disadvantage of the hydrothermal synthesis method is summarized in Table 3.
Table 3 Advantages and disadvantages of hydrothermal synthesis method
Synthesis methods |
Advantages |
Disadvantages |
Ref. |
Hydrothermal |
• Able to synthesize materials that are unstable near melting point |
• High cost of equipment such as autoclave |
46, 47, 49 and 60 |
• Ability to synthesize high quality products |
• Safety issues during the reaction process |
• Environment-friendly and versatile |
• Impossibility of observing the reaction process |
• Simple, easy, and inexpensive synthesis method |
• The reaction takes a long time |
• The morphology of products can be changed easily |
• High reaction temperature |
• Easy to control particle size, shape, and dispersion |
• High pressure |
• Production of high quality of 1D nanostructures such as nanorod |
• Usually, polydisperse samples are obtained |
• Good crystallization |
• Harsh reaction conditions |
• Magnetic controllability |
• Adsorption of capping agents |
• Reactants have elevated reactivity |
|
• Scalable |
|
2.1.4 Microemulsion. Stankic et al.85 reviewed the microemulsion method consisting of oil and water immiscible phases. These immiscible phases are separated by surfactant molecules, creating an oil/surfactant and water surfactant binary system. The surfactant molecules have properties of a hydrophobic tail, which is dissolved in the oil phase and a hydrophilic head, which is grouped to the water phase. To synthesize the homogenized phase, proper amounts of metallic precursor, surfactant, water, and oil are mixed while stirring at room temperature. For the precipitation of nanoparticles, oxidizing agent, reducing agent, and precipitating agent are added while stirring vigorously and thereafter, followed by centrifugation; subsequently, washing and drying or annealing takes place. The shape and size of metal oxide nanoparticles synthesized by this method can be easily manipulated. Different metal oxide nanoparticles such as Fe2O3,86 CeO2,87 NiO,88 ZnO,89 TiO2,90 and CuO91 were synthesized using the microemulsion method. In metal oxide synthesis, the microemulsion has advantages and disadvantages, as summarized in Table 4.
Table 4 Advantages and disadvantages of the microemulsion synthesis method
Synthesis methods |
Advantages |
Disadvantages |
Ref. |
Microemulsion |
• Easy and do not require energy during synthesis |
• Large amounts surfactants required, poor yield, and time consuming |
46, 49 and 61 |
• Better thermodynamic stability |
• Surfactants are difficult to remove |
• Low viscosity |
• For substances with high melting points limited solubility capacity |
• Uniform properties |
• Difficulty in scale up produces |
• Narrow pore size distribution |
• Microemulsion stability is influenced by parameters such as temperature and pH |
• Homogeneous particle size distribution and good control of shape |
|
• Increase the rate of absorption |
|
• Increase bioavailability |
|
• Helpful in taste masking |
|
• Eliminates variability in absorption |
|
2.1.5 Sonochemical synthesis. The sonochemical method uses ultrasound to facilitate the chemical reaction in which the ultrasound induces a chemical effect on the reaction process. In sonochemical synthesis, a starting material is dissolved in a solvent to get a solution. Thereafter, the resulting solution is irradiated with ultrasonic waves with a frequency above 20 kHz. Ultrasonic irradiation causes acoustic cavitation, which leads to the formation, growth, and implosive collapse of bubbles in a liquid.85 The implosive collapse of millions of bubbles in the liquid generates extremely high energy (temperature 5000 K and pressure 20 MPa) and is subsequently released, thereby heating the solution. In general, for metal oxide preparation, we follow three steps: (i) formation of metal hydroxide precursor's precipitate, (ii) ultrasonic irradiation, and (iii) transformation to metal oxide nanoparticles through heat treatment. The rate at which the solution is cooled affects the crystal shape and morphology of the resulting metal oxide nanoparticles. This method is advantageous as it is rapid, nonhazardous, and it produces metal oxide nanoparticles of high surface area, uniform size, and high purity products. In addition to this, the sonochemical method is a low-cost process and also produces small particle sizes.84 Various metal oxide nanoparticles such as TiO2,92 Cu-doped CeO2,93 La-doped CeO2,94 ZnO,95 Zn2SnO4–V2O5 nanocomposite,96 MoO3,97 Ag-PbMoO4,98 Co- and Mn-codoped W3O8,99 and Cu2O16 were produced using this method. Hassanjani-Roshan et al.100 prepared iron oxide nanoparticles using the sonochemical method, and they argued that the morphology and the size of the final product were influenced by the parameters such as power of ultrasonication, temperature, and sonication time. Noman et al.95 reported the successfully prepared ZnO nanoparticle by the sonochemical process for photocatalytic applications. The advantages and disadvantages of the sonochemical method are summarized in Table 5.
Table 5 Advantages and disadvantages of the sonochemical synthesis method
Synthesis methods |
Advantages |
Disadvantages |
Ref. |
Sonochemical |
• Improves the reaction rates and selectivity |
• The rate of sonochemical reduction completely depends on the ultrasonic frequency |
46, 47, 82–84 |
• Less toxic compounds and environmentally safe solvents are used |
• Extensions of problems |
• Reduction in the energy use for chemical transformations |
• Inefficient energy |
• Reusability of materials |
• Low yield |
• Reduced number of reaction steps |
|
• Successfully employed for less volatile organic liquids |
|
2.2 Dyes
Dyes are used for the coloration of several materials such as textile fibers, cosmetics, paper, food, tannery, leather, and pharmaceutical products.8 Generally, dyes can be classified as natural and synthetic. Natural dyes are the naturally existing dyes originating from plant parts including leaves, berries, wood, fungi, lichens, and bark, while synthetic dyes are man-made dyes synthesized from chemicals, petroleum derivatives, and earth minerals.101 Various synthetic dyes and their applications are presented in Fig. 2. These dyes are mostly used in food and beverages, printing, textile industries, and pharmaceuticals. The textile industry is the main contributor to the dyes released into the environment. The annually worldwide fabricated dyes are about 7 × 107 tons and over 10
000 different types of dyes are used by textile industries.9 The azo dye aniline yellow dye is used in textile industries in the production of nylon and silk. The acid dye acid blue is used in the production of cosmetics, wool, and leather. The reactive dye reactive red 198 is applied in the production of nylon, silk, and wool. The sulfur dye thiazine is used in the fabrication of paper, silk, and cotton. The mordant dye mordant red 11 is used in the production of anodized aluminum and wool. The direct dye direct yellow 11 is applied in the fabrication of paper, cotton, and leather. The disperse dye disperse red 60 is used in the production of plastic, polyamide, and nylon. A basic dye, methylene blue is used in inks and medicines.
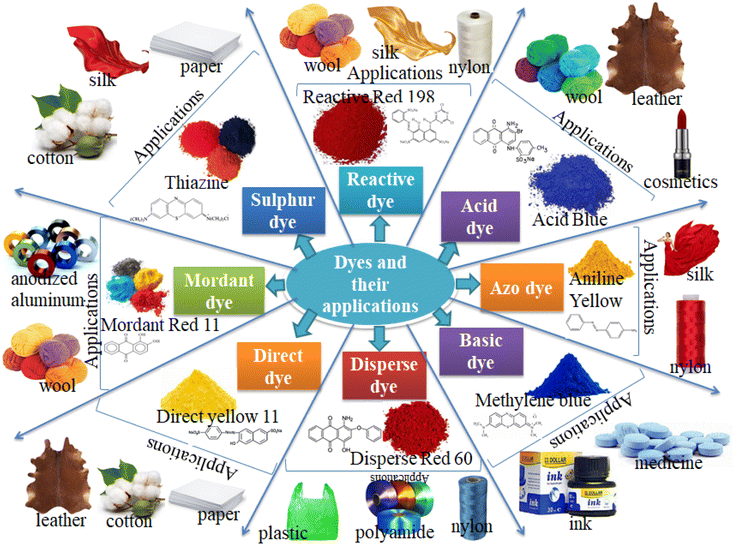 |
| Fig. 2 Different types of dyes and their applications in industries (colors online). | |
These dyes are used as coloration in textile industries and discharging textile industry wastewater into water bodies is not important as the color prevents reoxygenation in receiving water by cutting off the penetration of sunlight. These organic dyes have a complex structure and can produce aromatic amines as they are released into the environment. These carcinogens and hazardous dyes resist the sunlight entering into the water bodies, resulting in influencing the natural aquatic processes such as biodegradable and photosynthesis.102 When they are released into the environment, they cause environmental pollution and are highly toxic to aquatic life, microorganisms, and human beings as they are mutagenic and carcinogenic.10 Dyes found in wastewater discharge or dust produced in the textile industry pose a serious health concern to human beings, as presented in Fig. 3. These dyes affect our body systems such as the immune, respiratory, and reproductive and also affect vital organs including the heart, liver, kidney, and brain.8 They cause diseases either directly via inhalation such as nausea, respiratory problems, allergy, asthma, dermatitis, and eye or skin irritation, or indirectly via the food chain including tuberculosis, gene mutations, cancer, heart disease, and hemorrhage.
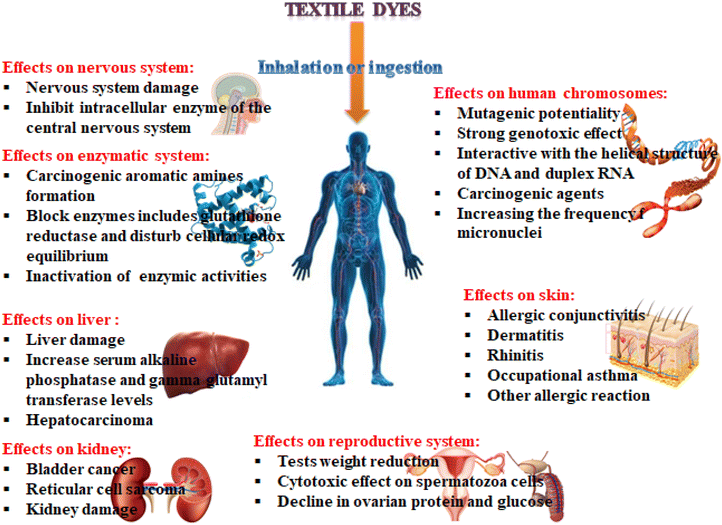 |
| Fig. 3 Negative effects of textile dyes on human health on different parts of the body (colors online). | |
2.2.1 Toxicity of methylene blue. The fabrication of synthetic dyes is a way to increasing due to their highly demanded in the textile and clothing industries. In addition to the aforementioned dyes, dyes such as crystal violet, aniline blue, basic fuchsin, congo red, toluidine blue, and methylene blue are also a few examples of synthetic dyes. These synthetic dyes remain in the environment when they are directly or indirectly released into the environment because most of them are nonbiodegradable and are not completely removed during conventional water treatment. Thus, they persist in the environment because of their high stability to light, temperature, water, and other substance such as detergent and soap.103 Effluents from textiles and factories using dyes release highly colored dyes, which can damage the aquatic environment.104 Dye molecules may resist sunlight penetration into the water bodies, thereby inhibiting natural photosynthesis.Methylene blue (MB) is applied in huge amounts as a colorant for silk, paper, cotton, and wool. In addition to these, cosmetics, food, and pharmaceutical industries consume large amounts of MB dye in their fabrications.105 MB is a cationic dye and a heterocyclic aromatic compound with a planar structure.103,106 It has C16H18N3SCl chemical formula with a molecular weight of 319.85 g mol−1. MB is highly soluble in water and forms a stable solution at room temperature. MB is a class of phenothiazine with an International Union of Pure and Applied Chemistry (IUPAC) name [3,7-bis(dimethylamino)phenothiazine chloride tetramethylthionine chloride] with color index (CI) 52
015.107,108 MB is nonbiodegradable and carcinogenic, having characteristic stability of the aromatic ring molecular structure, as presented in Fig. 4. When released partially or without treating, the MB dye-contaminated wastewater from any of the industries that used MB dye could cause a lot of human health risks, such as vomiting, increased heart rate, Heinz body formation, shock, quadriplegia, cyanosis, jaundice, and tissue necrosis in human beings.109 The health risks associated with the discharge of untreated MB-loaded wastewater includes respiratory disorder, genitourinary complications, gastrointestinal complications, cardiovascular issues, central nervous system, and dermatological effects, which are also included.110 The discharge of MB dye wastewater into the environment also causes risks such as reduction of pigment, growth inhibition, and protein content of microalgae Spirulina platensi and Chlorella vulgaris in plants.104 Moreover, the discharge of untreated MB dye-loaded water to either groundwater or surface water recently has caused a shortage of clean water in society. Commonly, developing countries release large amounts of MB dye-loaded wastewater into the physical environment without efficient and effective management.111
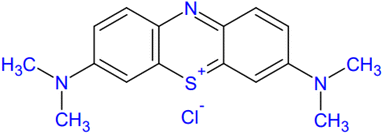 |
| Fig. 4 Molecular structure of Methylene Blue. | |
In addition, it is known that MB is commonly an inert dye, but it acts as a potent reversible monoamine oxidase (MAO) inhibitor (MAOI), which makes it difficult to find applications in medicine by ameliorating a clinical condition known as hypotension and improving hyperdynamic and hypoxia circulation in liver cirrhosis and severe hepatopulmonary syndrome.103,112,113 However, if infused intravenously at a dosage higher than the recommended concentration, MAOI may precipitate serious serotonin toxicity.113,114 Serotonin toxicity, also called serotonin syndrome, is a deadly disorder that is linked to elevated serotonergic activity in the spinal cord and brain.112 Also, skin-contacting MB using improperly or untreated MB-loaded water causes redness, itching, and skin necrosis, which causes the death of cells in tissues and organs.115 Generally, the discharge of MB-loaded wastewater from any utilization causes devastating effects on the environment. Therefore, it shall be removed or degraded before being exposed to the environment. However, it cannot easily be removed by a traditional treatment because of its nonbiodegradability and persistence. The photocatalytic mechanism is the most effective way to degrade these dyes.
2.3 Photocatalytic applications of metal oxides
Various metal oxides are used as photocatalysts in photocatalysis technology. Photocatalysis is potentially applied in fields including (i) photodegradation of organic pollutants, (ii) for hydrogen evolution by water splitting, and (iii) photoreduction of CO2 into renewable fuels such as CH4 and CH3OH.116 Metal oxide nanoparticles have been used in different areas including the removal of heavy metals, poisonous gas sensing, energy conversation and storage, biomedical, and photocatalytic removal of organic pollutants. In this review paper, we only overview the applications of different metal oxide nanoparticles in photocatalysis technology and we start our discussion with the mechanisms of photocatalysis.
2.3.1 General mechanism of photocatalysis. Photocatalysis is a chemical reaction that triggers or accelerates the specific reduction and oxidation (redox) reactions with the irradiated semiconductors.116 The major reactions involved in photodegradation are listed in eqn (1)–(5). The mechanism of photocatalytic degradation takes place with three general processes: (i) charge separation (hole and electron separation) under light irradiation, when the irradiated light match or exceeds the bandgap of the photocatalyst; (ii) distribution of charge carriers to the photocatalysis surface (MONPs); (iii) oxidation and reduction takes place on the surface of the photocatalyst.22 The process starts from the light adsorption of the photocatalyst by a certain wavelength when the light falls on the surface of the photocatalyst, which results in the excitation of electrons (e−) from the valence band (VB) to the conduction band (CB), leaving holes (h+) in the valence band, as shown in eqn (1).1,117 When the photocatalyst materials came in contact with organic pollutants, the holes created in the valence band split water molecules or react with OH− by resulting in hydroxyl free radical ˙OH, as shown in eqn (2),117 while the electrons (e−) excited to the conduction band react with O2 to create super oxygen radical O2˙− species, as shown in eqn (3).118 Both ˙OH and O2˙− are highly reactive species and they react with organic pollutants adsorbed/absorbed on the surface of the photocatalyst materials by degrading these pollutants or reducing them into H2O and CO2, as shown in eqn (5). The general photodegradation mechanism of photocatalysis is presented in Fig. 5. |
MONP + hν → (MONP)(h+)VB + (MONP)(e−)CB
| (1) |
|
(MONP)(h+)VB + H2O → H+ + ˙OH
| (2) |
|
(MONP)(e−)CB + O2 → O2˙−
| (3) |
|
(MONP)(e−)CB + O2˙− + 2H+→˙OH + OH−
| (4) |
|
pollutants + ˙OH → degradation byproducts (CO2 + H2O)
| (5) |
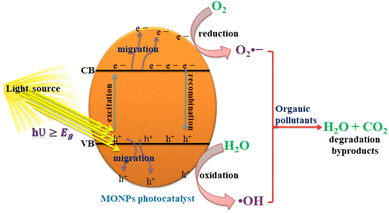 |
| Fig. 5 Schematic diagram of photocatalysis reaction mechanisms. | |
Oxidation and reduction:1
|
organic pollutants + photocatalyst (MONP(h+)VB) → oxidation products
| (6) |
|
organic pollutants + photocatalyst (MONP(e−)CB) → reduction products
| (7) |
The photocatalytic reaction takes place at ambient temperature and pressure without the production of additional sludge and a significant reduction of catalyst. The excited electrons are produced with strong reducibility while holes in the valence band with strong oxidability together result in the formation of the oxidation–reduction or shortly redox generated on the surface of the photocatalyst. The redox reaction takes place on the photocatalyst surface as the photogenerated electrons and holes are scattered on the surface of the photocatalyst. The oxidation reaction takes place by the cathode holes in the valence band reacting with the moisture on the surface of the photocatalyst to create the hydroxyl radicals. If oxygen is delivered from the outside to the photocatalytic reactor, it acts as an electron acceptor and delays the rate of charge recombination.
In semiconductor metal oxides, when the conduction band electrons possess a chemical potential of +0.5 to −1.5 V versus normal hydrogen electrode (NHE), then they act as reductants, while the valence band holes possess a strong oxidative potential of +1.0 to +3.5 V versus NHE.116,119 Thus, for a semiconductor to be photochemically active as a photocatalyst for the generation of ˙OH radicals, the redox potential of the photoinduced valence band hole must be sufficiently positive, whereas the redox potential of the photoinduced conduction band electrons must be sufficiently negative for the reduction of the adsorbed O2 to superoxide.120
There are constraints to the photocatalytic mechanism. Only the photons with energy equal or larger than the bandgap of the photocatalyst are used in the target reaction. Thus, the optimization and tuning of the bandgap is required to achieve high efficiency of the photocatalyst. Another main challenge in the processes of photodegradation of photocatalysis is the rapid recombination of charges (electron–hole pair's recombination) in photocatalysts without forming free radicals. To solve these challenges, many interesting options such as the manipulation of experimental conditions, producing low bandgap photocatalyst, and modification of the discovered photocatalyst are among the strategies undertaken to overcome these challenges.
2.3.2 Performance evaluation of a photocatalyst. Photocatalyst performance can be measured by the degradation of concentrations in liquids. The degradation efficiency of the photocatalyst can be calculated by eqn (8)121 |
degradation efficiency = (C0 − C)/C0 × 100
| (8) |
where C0 is the concentration of the dye before light irradiation and C is the concentration after a certain light illumination time.122 The Beer–Lambert law given by A = εcd is used to calculate the concentration of the organic compound in the liquid, where A is the absorbance, ε is the molar extinction coefficient, c is the absorber concentration of the absorbing species, and d is the optical path length.123The kinetic model, the Langmuir–Hinshelwood model, is used to evaluate the performance of the photocatalytic reaction occurring in the solid–liquid interface.124,125 The concentration of the organic pollutant, reaction parameters, temperature, and light irradiation intensity can be covered within the frame of the Langmuir–Hinshelwood model. For the first-order reaction, the Langmuir–Hinshelwood model is given as
where
C is the concentration,
t is the respective time, and
k is the rate constant. From the first-order plot, the rate constant can be calculated according to the equation
121where
k is the apparent first-order rate constant given by the slope of the graph of ln
C0/
C versus t.
2.3.3 Challenges in photocatalysis. The photocatalytic activity of the photocatalyst highly depends on its optical, crystallinity, structure, surface chemistry, and electronics. However, substantial challenges such as the high cost of semiconductors, lower solar energy utilization rate, and rapid recombination of photogenerated electron–hole pairs limit its potential applications in small-scale environmental areas.126 The stability, photocorrosion, and availability are also the challenges.127 The main challenge for the photocatalytic performance is associated with its semiconductor metal oxides including the mismatch of solar spectrum and bandgap of metal oxides, materials instability, and inefficient separation and transport of charges. The incident photon absorption efficiency of the photocatalyst is determined by its bandgap. Thus, most of the discovered photocatalysts such as TiO2 and ZnO have a wide bandgap, which made then active only under the ultraviolet region of the solar spectrum. The solar spectrum consists of 5% UV, 45% visible, and 50% infrared (IR), which means that UV is expensive and limits the wide applications in different areas. Therefore, bandgap tailoring is the fundamental challenge in photocatalysts to be solved. The recombination of photogenerated electron–hole pairs is another key issue influencing the photocatalytic activity of metal oxides. The recombination can occur either in the bulk or on the catalyst surface by dissipating energy as nonradiative (heat) or radiative (light) reducing the photocatalytic activity. The low surface chemistry also influences the photocatalytic activity of semiconductor metal oxides. In the photocatalytic reaction, the surface energy plays a great role in transferring electrons between the reactants. Various article reviews are reported on the strategies to develop a novel and high performance photocatalysts. Recently, Ahmad et al.128 reviewed on the most effective strategies for overcoming the shortcoming of photocatalysts to enhance their applications in pollutant degradation, CO2 reduction, H2 evolution, and N2 fixation. The strategies to suppress these challenges are described in detail in the next sections.
2.3.4 Doping in metal oxide nanoparticles. Doping is adding or substituting foreign elements either metals or nonmetals into parent elements, which can induce changes in the electronic and crystal structure of the parent oxide.129 Doping into host semiconductors is one of the most effective methods to manipulate the electronic structures, thus separating the charges efficiently.22 The main advantage of doping is to tune the bandgap of parent oxides either by increasing or decreasing the bandgap of metal oxides. Nonmetal dopants are fluorine, nitrogen, chlorine, carbon, iodine, phosphorus, selenium, sulfur, and tellurium, which are commonly used as dopants in metal oxides. These dopants can substitute in the site of oxygen or can exist as interstitial dopants.50 The contribution of orbital states of these dopants creates mixed or localized states at the edges of the valence band and/or conduction band edges, which cause the narrowing of the bandgap of metal oxides and subsequently enhance the visible light activity of metal oxides.130 A foreign dopant can also create an additional dopant impurity level at the middle of the bandgap, which initiates new excitations and increases the concentrations of photogenerated electrons and holes.131 The added or substituted impurity can also lead to the formation of defects, which can trigger new optical characteristics. Moreover, in photocatalytic applications, they can create an additional trapping state, which suppresses the rapid charge recombination and increases the lifetime of charge carriers.132 Metal dopants such as alkaline earth metals, transition metals, rare earth metals, and post-transition metals can also be used in the bandgap engineering of metal oxides.50,133,134 Metal ion dopants have the advantage of inducing defects and changing the diffusion behaviors, which can improve the electron mobilities and charge transport, thus enhancing the electrical properties.19,50 Metal doping can also activate the surface of the photocatalyst to boost the reaction. Generally, metal doping has advantages such as improving the mobility rate of the electron–hole pairs, formation of new energy states either inside or beyond the bandgap of host semiconductor metal oxides, and enhancing the visible light adsorption by the red-shifting of the adsorption edge.19
2.4 Metal oxides in photocatalytic degradation
A huge number of semiconductor metal oxide nanoparticles have been discovered for the degradation of pollutants in wastewater and air for a green and suitable environment. Among these metal oxides, TiO2 is the foremost discovered photocatalyst, which attracted many researchers around the world to engineer new photocatalyst materials. Thus, after the discovery of TiO2 in 1972, many other metal oxides are discovered for photocatalytic applications. Photocatalysts are generally divided into two main categories: (i) homogeneous catalysts and (ii) heterogeneous catalysts. When a catalyst is present in the same phase as that of the reagents, it is defined as homogeneous, which means that the catalysts are present as solutes in a homogeneous catalytic reaction system. In contrast, a catalyst is said to be heterogeneous if it is present in a different phase in the reaction mixture.14 In heterogeneous photocatalysis, the photocatalyst acts as an active surface for the reaction or mineralizing of organic pollutants by generating free radicals, which are capable of the complete mineralization of organic pollutants through complex mechanisms.5 In this section, we have overviewed the commonly used metal oxide nanoparticles in photocatalysis technology.
2.4.1 TiO2 photocatalyst. Titanium dioxide (TiO2) is the leading photocatalytic material since its discovery in 1972 due to its low cost, corrosion resistance, strong oxidizing power, and photochemical and chemical stability. TiO2 is an n-type semiconductor metal oxide and it has the capability of oxidizing organic pollutants into nonhazardous products completely.135,136 Despite its high performance in the photocatalytic degradation of polluted air and wastewater pollutants, its photocatalytic activities are limited due to the rapid recombination of the photogenerated electron and hole pairs, poor sensitivity to visible solar radiation because of its wide bandgap, and TiO2 particles aggregated when produced in nanoparticle form, which blocks the active sites to adsorb/absorb pollutants that hinders its activity in photocatalysis technology.28Many researchers are interested in overcoming these challenges by taking different options to improve the photocatalytic activity of TiO2 nanoparticles. To enhance its poor sensitivity to the visible light region, it is important to reduce the bandgap and decrease the rate of charge recombination to improve its lifetime for photocatalytic applications. Researchers have proposed a strategic approach to address these challenges. The well-studied and sufficient approach is metal-ion doping, which introduces additional energy levels and reduces the bandgap. Metal-ion doping also introduces a charge-trapping site, which decreases the rapid recombination of photogenerated electron and hole pairs. Different metal-ion doped TiO2 is extensively studied with the advantage of increasing its absorption spectrum in visible light because the visible light region of the solar spectrum is freely available everywhere. Miyamoto et al.135 synthesized lutetium(III) ion-doped TiO2 by the sol–gel method to modify the photocatalytic performance of TiO2 for the photocatalytic degradation of adenosine 5′-triphosphate (ATP) under UV irradiation. Their result showed that the lutetium(III) ion-doped TiO2 nanoparticles indicated higher photoreactivity than undoped TiO2. Garza-Arévalo et al.137 synthesized Fe-doped TiO2 by the sol–gel method and evaluated the removal of arsenic(III) from groundwater under visible light. The incorporation of Fento TiO2 extends the absorption of TiO2 to visible light region, and induces oxygen vacancies, which initiate the photocatalytic oxidation of arsenic(III) and retarded the fast recombination of photogenerated electrons and holes. Ndabankulu et al.12 reported different lanthanides (Ce, Dy, Lu, and Sm)-doped TiO2 by the sol–gel process and evaluated the photocatalytic activity and efficacy of four different lanthanides-doped TiO2 for the degradation of caffeine under visible light irradiation. Sood et al.18 reported iron(III)-doped TiO2 nanoparticle photocatalyst synthesized by the hydrothermal method and examined its photocatalytic activity under the illumination of visible light spectrum using para-nitrophenol as a model pollutant. The maximum degradation they obtained was 92% in 5 h with 0.05 mol% Fe3+, which was attributed to the high crystalline structure, small size, maximum charge separation, retarded electron–hole recombination, and better visible light response of the prepared Fe3+-doped nanoparticle. Xie et al.138 reported Se4+-doped TiO2 nanoparticles synthesized by the sol–gel method for the degradation of rhodamine B under visible light irradiation. Se4+ introduces extra electronic states in the bandgap of TiO2 and narrowed the bandgap of TiO2, as presented in Fig. 6a. The smallest bandgap they obtained was 2.17 eV and due to the extended absorption range, the photocatalytic activity of TiO2 was improved. However, as the percentage concentration of Se4+ increased to 25%, the bandgap started to increase; hence, the photocatalytic activity also decreased.
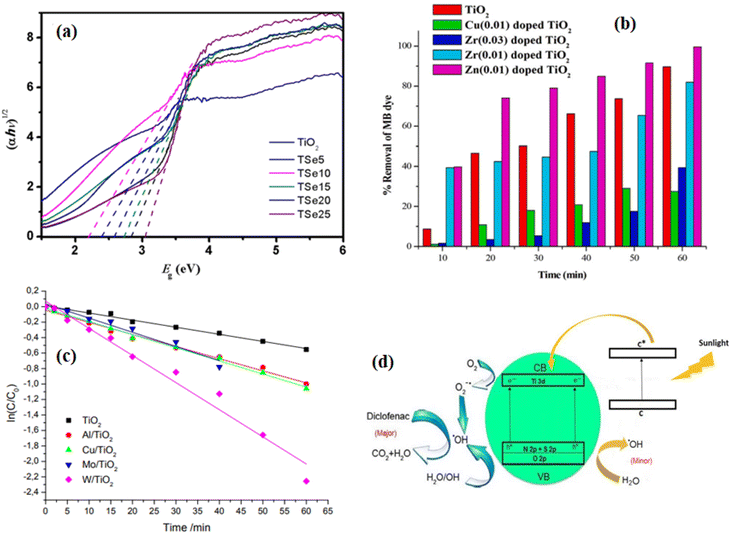 |
| Fig. 6 (a) Optical bandgap of different percentage concentrations of Se-doped TiO2 photocatalyst;138 (b) bar diagram of % removal of methylene blue (MB) dye with time under visible light irradiation using pure TiO2 and Zn, Cu, Zr-doped TiO2 catalyst;139 (c) kinetic curves of rhodamine B destruction over the undoped and doped TiO2 under the visible light irradiation;140 (d) schematically representation of the improvement in the photoactivity of C–S–N-tridoped TiO2 photocatalyst.142 | |
Depositing noble metals such as Ag, Pd, Au, and Pt on the surface of TiO2 retards the fast recombination of photogenerated electron–hole pairs. Narkbuakaew and Sujaridworakun reported the Ag metal deposited on the surface of anatase TiO2 prepared by chemical reduction, followed by the calcination method for the degradation of rhodamine B, and the deposition of silver nanoparticles significantly inhibited the fast recombination of photogenerated electron–hole pairs.136 Metal doping is another strategy for reducing charge recombination and enhancing the visible light response of the TiO2 photocatalyst. Karuppasamy et al.139 synthesized different transition metals (Zn, Zr, Cu)-doped TiO2 by the sol–gel method and utilized them for the decoloration of methylene blue dye under visible light irradiation. The decoloration efficiency of the prepared materials is presented in Fig. 6b. Among the doped TiO2, the Zn-doped photocatalyst showed greater photocatalytic activity than pristine TiO2 or other doped photocatalysts. Thus, the enhanced photocatalytic activity of Zn-doped TiO2 is attributed to the decrease in the optical bandgap compared to pristine and other dopants. Khlyustova et al.140 synthesized Al, Cu, Mo, and W-doped TiO2 by the sol–gel method and tested the photocatalytic activity of the doped and pristine TiO2 using rhodamine B as the pollutant model under the illumination of visible light. As shown in Fig. 6c, the doped photocatalysts have enhanced photocatalytic performance compared to pristine TiO2. Thus, the doped photocatalysts have a higher kinetic rate constant than pristine TiO2, which resulted in enhanced photocatalytic performance due to the formation of a new energy level near the conduction band. However, metal doping possesses disadvantages such as photocorrosion and high cost specifically when using noble metals as a dopant. To solve these problems, nonmetal doping such as iodine, sulfur, and nitrogen were used as dopants to enhance the photocatalytic performance of TiO2. Saroja et al.141 reported the iodine-doped TiO2 synthesized through the solution combustion method and investigated the effects of iodine dopant concentration on the photocatalytic activity of TiO2 for the photodegradation of Direct Blue 199. They observed that the visible light response of iodine-doped TiO2 was enhanced as the dopant concentration increased.
Nonmetal co- and triple-doped TiO2 for the enhancement of its photocatalytic activity was also reported by different groups. For instance, Ramandi et al.142 reported C–N–S-tri doped TiO2 anatase phase synthesized b they sonochemical method. They studied the photocatalytic activity of the prepared photocatalysts using diclofenac as a model pollutant under sunlight irradiation. The photocatalytic degradation mechanism of the C–N–S triple-doped TiO2 is shown in Fig. 6d. Each nonmetal dopant has its own advantages in enhancing the photocatalytic activity of TiO2 by generating new impurity levels such as N 2p, S 2p, and C 1s. Thus, the doped C acts as a photosensitizer, which injects electrons into the conduction band (CB) under sunlight irradiation, while N and S doping modifies the electronic band structure of TiO2 by mixing the O 2p of TiO2 with N 2p and S 2p orbitals, which resulted in the narrowing of the bandgap of TiO2. According to this report, the electrons in the CB and the trapped electrons trap the adsorbed O2 molecules to generate the superoxide (O2˙−). The main oxidative and reactive species were electrons and ˙OH radicals, holes were the minor oxidative species, and O2˙− has considerable effects on the degradation of diclofenac. The ˙OH radicals can be obtained in two ways, as shown in eqn (11)–(13). In eqn (11), molecular O2 reacts with the electrons (e−) in the CB and resulted in O2˙−. Then, O2˙− reacts with water molecules to produce ˙OH radical species, as shown in eqn (12). On the other hand, as shown in eqn (13), the h+ can react with H2O on the surface of photocatalyst to produce ˙OH radical species.
|
O2˙− + 2H2O → 2˙OH + 2OH− + O2
| (12) |
The immobilization of TiO2 on carriers such as activated carbon and metallic oxides is also an effective technique to retard the fast charge recombination and extend the visible light region response of the TiO2 photocatalyst. Saqib et al.143 reported zeolite-immobilized TiO2 nanoparticles synthesized using the conventional liquid impregnation method for the efficient degradation of methylene blue dye. The presence of zeolite improves the photocatalytic degradation of methylene blue by four folds compared to pure TiO2. Coupling TiO2 with other semiconductors to develop heterojunctions is also another method for enhancing the photocatalytic activity of TiO2. Coupling TiO2 with other semiconductors expands the visible light spectrum to the near-infrared response of TiO2 as well as promotes charge separation.28 Song et al.144 synthesized a novel TiO2/Bi2MoO6 heterostructures via a solvothermal process for the partial oxidation of aromatic alkanes under visible light illumination. They observed that compared to single components TiO2 and Bi2MoO6, the heterostructures TiO2/Bi2MoO6 showed better photocatalytic performance, and the charge separation was also improved.
Heterojunction modes of metal oxide photocatalysts with a similar band structure when coupled enhance the transfers, separation, and stability of photogenerated electrons and holes. For instance, Abdel-Wahab et al.145 reported the coupled heterojunctions TiO2/Fe2O3 with the bandgap of TiO2 of ∼3.2 eV and Fe2O3 bandgap of ∼2.2 eV. When the TiO2/Fe2O3 heterojunction was irradiated, the electron–hole pairs were generated, as shown in Fig. 7. Thereafter, the photogenerated electrons transfer from the conduction band (CB) of Fe2O3 to the conduction band (CB) of TiO2, with lower potential, whereas the photogenerated holes transfer from the valence band (VB) of TiO2 to the valence band (VB) of Fe2O3 with higher potential. The photogenerated electrons eventually reacted with oxygen molecules to produce O2˙− active species, while the photogenerated holes reacted with water molecules to produce ˙OH radicals. The prepared TiO2/Fe2O3 heterojunction was applied for the degradation of paracetamol.
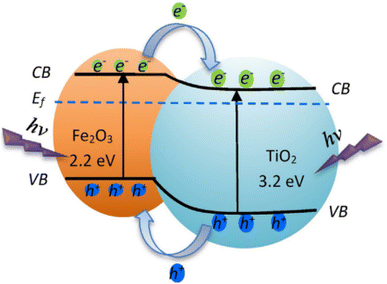 |
| Fig. 7 Schematic diagram of charge transfer in the photoexcited TiO2/Fe2O3 core–shell photocatalysis.145 | |
Generally, the comparison of pure, doped, noble metals immobilized, composites, and heterojunction of TiO2-based photocatalysts are summarized in Table 6.
Table 6 Summary of TiO2-based photocatalysts for the photodegradation of organic pollutants
Catalysts |
Synthesis methods |
Morphology |
Model pollutant |
Light |
Reaction time |
Degradation efficiency |
Ref. |
TiO2 |
In situ complexation-hydrolysis |
Hierarchical mesoporous nanoshell |
Methylene blue |
UV |
40 min |
∼100% |
146 |
Fe-doped TiO2 |
Sol–gel |
Nanoparticles |
Arsenic(III) |
Visible light |
3 h |
99% |
137 |
Fe3+-doped TiO2 |
Hydrothermal |
Nanoparticles |
Para-nitrophenol |
Visible light |
5 h |
92% |
18 |
Se4+-doped TiO2 |
Sol–gel |
Nanoparticles |
Rhodamine B |
Visible light |
30 min |
91.3% |
138 |
MWCNT/TiO2 |
Sol–gel |
Nanoparticles |
2,4-Dichlorophenol |
UV and solar light |
120 min |
93% |
147 |
MWCNT/TiO2 |
Sol–gel |
Nanoparticles |
2,4-Dichlorophenol |
Solar light |
120 min |
87% |
147 |
Zn-doped TiO2 |
Sol–gel |
Nanoparticles |
Methylene blue |
Visible light |
60 min |
99.64% |
139 |
Mo- and W-doped TiO2 |
Sol–gel method |
Nanoparticles |
Rhodamine B |
Visible light |
60 min |
96% |
140 |
Lu3+-doped TiO2 |
Sol–gel |
Microparticles |
ATP |
UV |
90 min |
— |
135 |
Ce-doped TiO2 |
Sol–gel |
Nanoparticles |
Caffeine |
Visible light |
120 min |
— |
12 |
Iodine-doped TiO2 |
Combustion method |
Nanoparticles |
Direct blue 199 |
Visible light |
— |
— |
141 |
C–N–S-tri doped TiO2 |
Sonochemical method |
Nanoparticles |
Diclofenac |
Sunlight |
180 min |
100% |
142 |
Zeolite-immobilized TiO2 |
Impregnation |
Nanoparticles |
Methylene blue |
Compact fluorescent light |
180 min |
94% |
143 |
TiO2/Bi2MoO6 |
Solvothermal |
Nanoparticles |
Oxidation of aromatic alkanes |
Visible light |
— |
— |
144 |
TiO2/ZrO2 |
Solvothermal |
Nanoparticles |
Rhodamine B |
Visible light |
180 min |
99% |
148 |
RGO-TiO2 |
Hydrothermal |
Nanoparticles |
Methyl orange |
UV |
60 min |
100% |
149 |
TiO2/Fe2O3 |
Ultrasonic-assisted sol–gel |
Core–shell nanoparticles |
Paracetamol |
Mercury lamp |
90 min |
99% |
145 |
g-C3N4/TiO2 |
Sol–gel |
Nanotubes |
Rhodamine B |
Visible light |
80 min |
∼97% |
150 |
g-C3N4/TiO2 |
Template |
Nanospheres |
Rhodamine B |
Visible light |
80 min |
92% |
150 |
g-C3N4/TiO2 |
Template |
Nanorod |
Rhodamine B |
Visible light |
80 min |
94.5% |
150 |
Z-scheme g-C3N4/TiO2 |
Impregnation/hydrothermal |
Nanotubes |
Isoniazid |
Visible light |
4 h |
90.8% |
151 |
Ag metal deposited on TiO2 |
Chemical reduction |
Nanoparticles |
Rhodamine B |
UV |
1 h |
— |
136 |
TiO2/Ag/rGO |
Hydrothermal |
Nanoparticles |
Methylene blue |
Visible light |
45 min |
100% |
152 |
rGO@TiO2/CN |
Hydrothermal |
Nanocomposite |
Rhodamine B |
Solar light |
120 min |
97% |
153 |
2.4.2 ZnO photocatalyst. Recently, many researchers are highly interested in zinc oxide (ZnO) nanoparticles due to its simple synthesis, low cost, and unique physical and chemical properties, which made it compete with TiO2 in photocatalytic activity degradation of organic pollutants. It has excellent photocatalytic activity under UV irradiation but is less stable compared to TiO2.26 ZnO is an n-type semiconductor having a wide bandgap of 3.37 eV.154 Compared to TiO2, ZnO responds to broader ranges of the light spectrum including the visible light spectrum.The photocatalytic activity of ZnO is widely studied and the synthesis method affects the specific surface area of ZnO nanoparticles, which subsequently affects the photocatalytic activity of ZnO nanoparticles. Uribe-Lopez et al.155 reported the effect of the synthesis method on the photocatalytic activity of ZnO nanoparticles synthesized by the precipitation and sol–gel method. They obtained hexagonal rod-like geometries with 50 nm diameters for the ZnO nanoparticle synthesized via the sol–gel method and quasi-spherical geometries with 100 nm diameters for ZnO nanoparticles via the precipitation method. They estimated the photocatalytic activity of the synthesized ZnO via both methods by the degradation of phenol and achieved 100% degradation after 120 min for nanoparticles via the precipitation method, whereas they achieved 80% degradation for nanoparticles via the sol–gel method after 120 min. Pure ZnO nanoparticles only show the UV light spectrum, which only covers approximately 4% of the solar spectrum; thus, it is important to enhance the visible light response because visible light covers approximately 43% of the solar spectrum, which is much larger than the UV spectrum.156 In addition to this, because of the wide bandgap of ZnO, the photogenerated electrons and holes easily recombine, which limits its photocatalytic performance.
To improve the visible light irradiation response and slow down the fast charge recombination of photogenerated electrons–holes pairs of ZnO nanoparticles, different approaches have been developed. Doping with metal ions and metals is a famous approach, in which it reduces the electron–hole recombination and expands the visible light spectrum responses. Ji et al.131 reported the Fe3+- doped, Sn4+-doped, and Fe3+ and Sn4+-codoped ZnO nanoparticles prepared by a simple low-temperature solution method and evaluated its photocatalytic activity by the degradation of methylene blue dye. The low to high photocatalytic activity sequence was ZnO, Fe0.01ZnO, Fe0.01Sn0.01ZnO, and Sn0.05ZnO, as presented in Fig. 8a. Pristine ZnO nanoparticles only achieved 45.88% of methylene blue dye degradation, whereas Sn0.05ZnO achieved the highest degradation of 99.61% of all the four materials. When Fe3+ and Sn4+ were incorporated into ZnO, an impurity level near the conduction band was formed, which allows the electrons in the valence band to transition with less energy, as shown in Fig. 8b. Consequently, the concentrations of photogenerated electrons and holes increased and also improved the photocatalytic performance of ZnO nanoparticles. Alam et al.157 synthesized different rare earth metals (La, Nd, Sm, and Dy)-doped ZnO nanoparticles by the sol–gel process and evaluated the photocatalytic degradation of methylene blue and rhodamine B by the improved photocatalyst due to the doped rare earth metals. Qi et al.158 reported transition metal ions (Mn, Fe, Co, Ni, or Cu)-doped ZnO nanoparticles synthesized via a solvothermal method. They studied the photodegradation of methylene blue dye under the simulation of visible light. As shown in Fig. 8c and d, the photocatalytic activity of the doped ZnO was increased compared to bare ZnO. Among all the doped photocatalysts, Cu-doped ZnO showed greater photocatalytic activity than bare ZnO and other doped photocatalysts. Thus, the Cu-doped ZnO has the highest rate constant than other photocatalysts, as depicted in Fig. 8d.
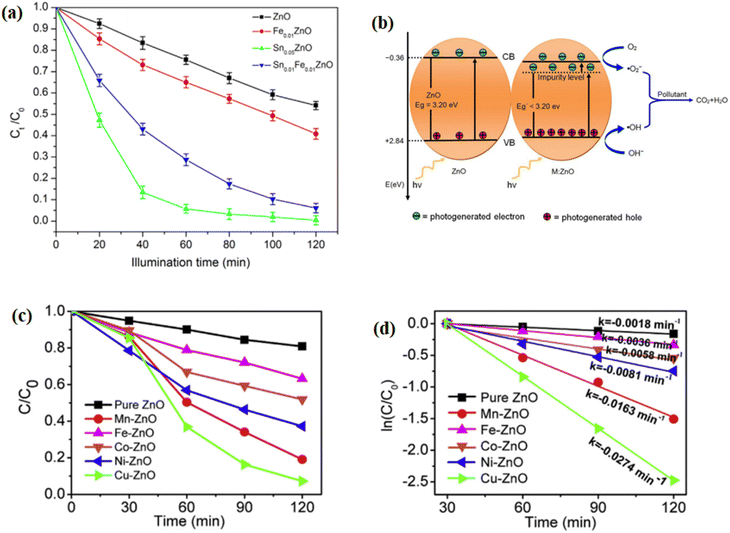 |
| Fig. 8 (a) Photodegradation of methylene blue (MB) dye with different catalysts and (b) photocatalytic schematic diagram of ZnO with the impurity level introduced;131 (c) photocatalytic activity for the decomposition of MB of the pure ZnO and transition metal-doped ZnO samples under simulated solar light irradiation (photocatalyst dosage, 0.1 g per 200 mL) and ln(C/C0) vs. time slope for the kinetic rate constant.158 | |
Nonmetal-doped ZnO photocatalyst with improved photocatalytic activity under visible light irradiation is also reported. Mirzaeifard et al.159 reported sulfur-doped ZnO synthesized by the hydrothermal method and utilized it for the degradation of rhodamine B under visible light irradiation. They optimized the dosage of the catalyst for the best degradation of rhodamine B, as shown in Fig. 9a. Among those, 0.10 g photocatalyst containing 0.5 wt% of S showed a 100% rate degradation of rhodamine B (5 ppm) at pH = 5 in 90 min.
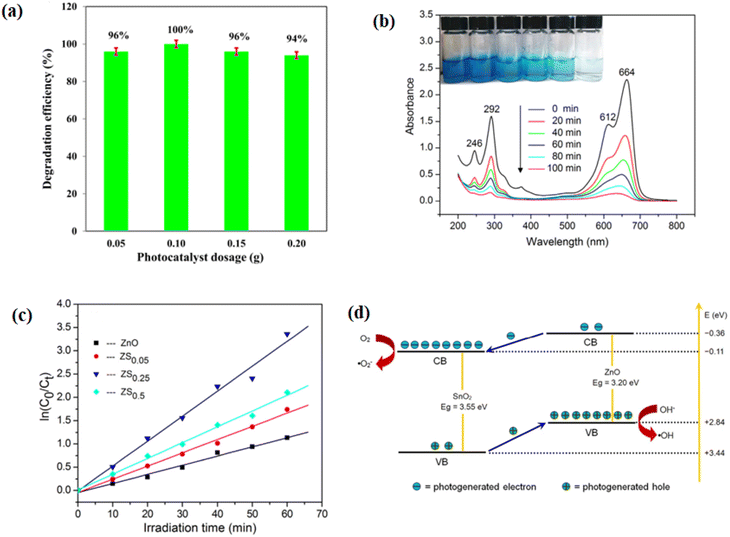 |
| Fig. 9 (a) Degradation efficiency of various dosages of the 0.5 wt% S-doped ZnO photocatalyst;159 (b) UV-vis spectra of the MB solutions at different irradiation times (the inserted image shows the color change of the MB solution), (c) kinetic linear simulation curves of MB degradation and (d) schematic of the band structure and e−–h+ separation in the ZS composite.162 | |
Immobilizing the ZnO nanoparticles on the polymer supports is another method to improve the photocatalytic activity of ZnO. Mochane et al.160 reviewed the ZnO nanoparticles immobilized in various matrices such as polymers and ceramics. They discriminated that the ZnO nanoparticles were immobilized on the polymer because of high durability, chemical inertness, and low cost. ZnO coupled with other semiconductor metal oxide nanoparticles such as SnO2, TiO2, and WO3 is also another approach to improve the photocatalytic activity of ZnO under the irradiation of visible light. Mugunthan et al.161 reported the visible light irradiation photocatalytic degradation of the pharmaceutical compound diclofenac in wastewater using a ZnO–WO3 composite semiconductor photocatalyst synthesized through a hydrothermal method. The prepared composite photocatalyst extended the optical response to the visible light spectrum and it showed better stability in retaining the degradation efficiency of 80%. Lin et al.162 also synthesized ZnO–SnO2 (ZS) nanocomposites with different compositions by a simple coprecipitation method. As shown in Fig. 9b, the aqueous solution of methylene blue dye color becomes colorless with increasing irradiation time. Among the composition of the synthesized nanocomposite, 25% SnO2 showed the optimum rate constant, as depicted in Fig. 9c. Fig. 9d shows the schematic representation of band structure and electron–hole separation ZnO–SnO2 composites. As shown in the schematic, the conduction band (CB) potential of ZnO (−0.36 eV) is lower compared to SnO2 (−0.11 eV) and the valence band (VB) potential of SnO2 (+3.44 eV) is higher than the VB potential of ZnO. When ZnO–SnO2 composites were irradiated, the photogenerated electrons flow from low potential to high potential while holes flow in the opposite direction. As a result, the electrons accumulated in the conduction band of SnO2 and the holes accumulated in the valence band of ZnO. Thus, the sufficiently separated photogenerated electrons and holes provided the enhanced photocatalytic performance of the ZnO–SnO2 composite. The general summarization of ZnO-based photocatalysts are given in Table 7.
Table 7 Summary of ZnO-based photocatalysts for the photodegradation of organic pollutants
Catalysts |
Synthesis methods |
Morphology |
Model pollutant |
Light |
Reaction time |
Degradation after action |
Ref. |
ZnO |
Precipitation |
Nanoparticles |
Phenol |
UV |
120 min |
100% |
155 |
ZnO |
Sol–gel |
Nanoparticles |
Phenol |
UV |
120 min |
80% |
155 |
Sn0.05ZnO |
Low-temperature solution |
Nanoparticles |
Methylene blue |
UV |
120 min |
99.61% |
131 |
Nd-doped ZnO |
Sol–gel |
Nanoparticles |
Methylene blue |
UV |
25 min |
98% |
157 |
Cu-doped ZnO |
Solvothermal |
Nanoparticles |
Methylene blue |
Simulated sunlight |
2 h |
81% |
158 |
Sulfur-doped ZnO |
Hydrothermal |
Nanoparticle |
Rhodamine B |
Visible light |
90 min |
100% |
159 |
ZnO–WO3 |
Hydrothermal |
Nanoparticle |
Diclofenac |
Visible light |
270 min |
80% |
161 |
ZnO–SnO2 |
Coprecipitation |
Nanocomposites |
Biebrich scarlet dye |
UV |
40 min |
97% |
163 |
ZnO–TiO2 |
Hydrothermal |
|
Nitrophenol |
UV |
25 min |
95% |
164 |
GO/ZnO |
Multistep solution-based |
Nanostructure |
Methylene blue |
Visible light |
70 min |
100% |
165 |
rGO/ZnO |
Hydrothermal |
Nanosheets |
Methylene blue |
Visible light |
80 min |
100% |
166 |
GO/ZnO |
Sol–gel |
Nanoparticles |
Rhodamine B |
Visible light |
70 min |
99% |
|
rGO/ZnO |
Hydrothermal |
Nanoparticles |
Methylene blue |
Visible light |
60 min |
100% |
167 |
Ag-doped ZnO/CNTs |
Microwave-assisted |
Nanoparticle |
Reactive black GR |
Solar light |
30 min |
100% |
168 |
Ag-doped ZnO/CNTs |
Microwave-assisted |
Nanoparticle |
Reactive black GR |
Visible light |
120 min |
100% |
168 |
Cu-doped ZnO/g-C3N4 |
One-pot pyrolysis |
Nanocomposite |
EBT dye |
Visible light |
90 min |
99% |
169 |
ZnO–SnO2–Sn |
— |
Nanocomposite |
Methylene blue |
UV |
90 min |
95.6% |
170 |
2.4.3 WO3 photocatalyst. The first experiment on the photocatalysis of tungsten oxide (WO3) was reported in 1976.171 Tungsten oxide is an inexpensive metal oxide semiconductor having high and promising photocatalytic performance. It has advantages such as good response to the solar spectrum due to its narrow optical bandgap (2.5–2.8 eV), tunable stoichiometric structure, earth abundance, high oxidizing of holes, and stability.28 Despite its high photocatalytic performance, the photocatalytic efficiency of WO3 is limited due to the rapid recombination of photogenerated electrons and holes and less reduction of O2 molecules because of the relatively low conduction band level that lies above the reduction potential of O2/O2˙−. These challenges have inspired researchers to find promising techniques to suppress these challenges by tuning the structure and optical bandgap of WO3. These techniques include the manipulation of particles morphology, deposition of noble metal on WO3, doping of elements, carbon-based composite, and forming heterojunctions by coupling with other semiconductors.Particle morphology control reduces the fast photogenerated electrons and holes recombination, improves charge separation, and enhances the overall photocatalytic activity. Rong and Wang synthesized novel hierarchical hollow nest-like WO3 micro/nanostructures (HNWMs) by the hydrothermal method without a template and a surfactant.172 The effects of different parameters such as time of reaction, pH, and annealing conditions have been investigated according to this report. The time-dependent experimental SEM morphology of hierarchical hollow nest-like WO3 micro/nanostructure is presented in Fig. 10a–i, and the hydrothermal treatment process is shown in Fig. 10j. This morphological structure of WO3 retained an improved photocatalytic activity toward an aqueous solution of tetracycline (TC) under visible light and possesses good stability and reusability.
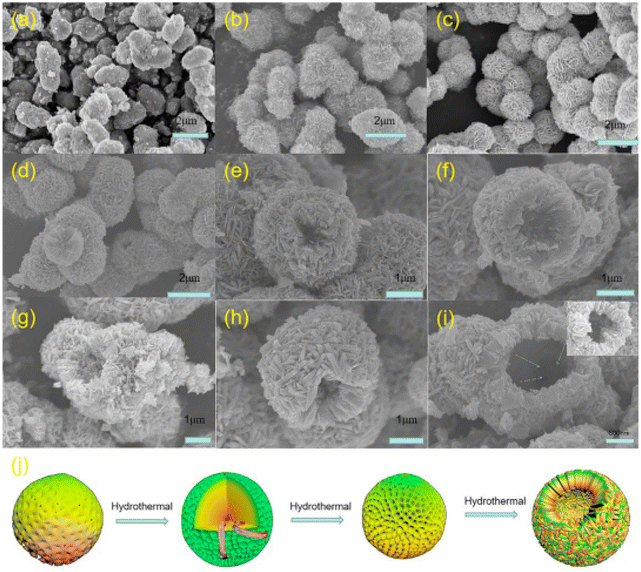 |
| Fig. 10 SEM images of the hollow nest-like WO3 micro/nanostructures obtained after hydrothermal treatment at different times: (a) 3 min; (b) 9 min; (c) 15 min; (d) 2 h; (e) 4 h; (f) 6 h; (g) 8 h; (h) 10 h; and (i) 16 h; (j) schematic diagram of the hollow nest-like WO3 micro/nanostructures formation process.172 | |
Metal doping can narrow the bandgap and retarded the fast charge recombination of the WO3 photocatalyst. Ramkumar and Rajarajan synthesized nanocrystalline Ni-doped WO3 thin film deposited by chemical bath deposition method and evaluated its photocatalytic activity by the photodegradation of methyl orange, methylene blue, and phenol.173 The photocatalytic activity of Ni-doped WO3 was remarkably improved. Noble-metal deposition and coupling with other semiconductors also enhances the photocatalytic performance of WO3 under visible light irradiation. Wang et al.174 reported the multiple heterojunctions containing WO3 nanorods and Pt and TiO2 nanoparticles for the degradation of gaseous acetaldehyde, rhodamine B dye, and phenol under the illumination of visible light. The multiheterojunction (WO3–Pt)/TiO2 photocatalyst's higher performance was attributed to the synergistic effect of efficient electrons and holes transfer at the WO3/Pt interface and WO3/TiO2 interface, respectively.
Graphene, reduced graphene oxide, and other carbon-based composites also enhance the photocatalytic performance of WO3.175,176 Jeevitha et al.175 prepared the WO3-GO nanocomposite via the ultrasonic method and investigated the photocatalytic activity, antibacterial, and anticancer of the prepared nanocomposite. The irregular spherical shape and small pores of WO3 greatly improved the photocatalytic activity. The irregular spherical shape with small pores of WO3 is shown in Fig. 11a and b. The morphology of graphene oxide (GO) is a sheet, as shown in Fig. 10c and d. The well-distributed nanosphere WO3 on the graphene sheet is shown in Fig. 11e and f. This report compared the obtained photocatalytic degradation of methylene blue dye under the illumination of visible light, as shown in Fig. 11g and h. Fig. 11g shows the photocatalytic activity of pure WO3 and in 270 min, it could not completely degrade the methylene blue dye. However, the WO3-GO nanocomposite achieved a 97.03% rate of degradation of methylene blue dye in 180 min, as presented in Fig. 11h. The summary of WO3-based photocatalysts is given in Table 8.
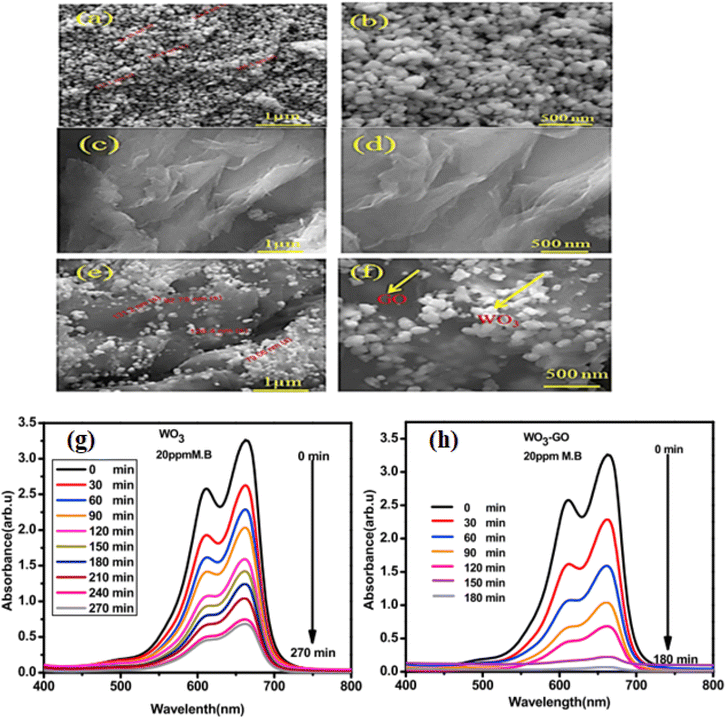 |
| Fig. 11 FE-SEM image of (a and b) WO3, (c and d) GO, and (e and f) WO3-GO nanocomposite; (g) absorbance spectra of MB by pure WO3 and (h) absorbance spectra of methylene blue dye by the WO3-GO nanocomposite.175 | |
Table 8 Summary of WO3-based photocatalysts for the photodegradation of organic pollutants
Catalysts |
Synthesis methods |
Morphology |
Model pollutant |
Light |
Reaction time |
Degradation after action |
Ref. |
WO3 |
Hydrothermal |
Hierarchical hollow nest-like |
Tetracycline |
Visible light |
60 min |
94.3% |
172 |
Ni-doped WO3 |
Chemical bath deposition |
Thin film |
Methyl orange |
Visible light |
150 min |
88% |
173 |
(WO3–Pt)/TiO2 |
Hydrothermal |
Nanoparticles |
Rhodamine B |
Visible light |
90 min |
98.8% |
174 |
WO3-GO |
Ultrasonic method |
Nanocomposite |
Methylene blue |
Visible light |
180 min |
97.03% |
175 |
rGO/WO3 nanoplate |
One-step hydrothermal |
Nanohybrids |
Methylene blue |
Sunlight irradiation |
90 min |
82.2% |
177 |
rGO/WO3 |
Hydrothermal |
Nanorod |
Rhodamine B |
Visible light |
120 min |
94% |
178 |
Ag@WO3@rG |
Hydrothermal |
Nanocomposites |
Rhodamine B |
Solar simulation |
70 min |
99.5% |
179 |
WO3/g-C3N4/h-BN |
Thermal treatment |
Lamellar structure |
Tetracycline |
Visible light |
60 min |
81.4% |
180 |
Indigo-RGO/WO3 |
Hydrothermal |
Nanosheets |
Methylene blue |
Sunlight |
120 min |
∼80 |
181 |
2.4.4 Cu2O photocatalyst. Copper oxide nanoparticle is the most probable candidate of promising photocatalytic materials for the degradation of organic pollutants. It possesses high optical absorption properties and optimal optical bandgap. Copper oxide nanoparticles can be produced at a low cost due to its precursor materials being highly abundant in the earth's crust.16 It has two semiconducting phases, namely, cupric oxide (CuO) and cuprous oxide (Cu2O), which possesses approximately 1.6 eV and 2.4 eV optical bandgap, respectively. Cu2O is a p-type metal oxide semiconductor with a 2.4 eV bandgap.188 Since its bandgap is optimal for the application of photocatalysis under visible light illumination, it is the preferable candidate for photocatalytic activity. Muthukumaran et al.16 synthesized cuprous oxide (Cu2O) nanoparticles photocatalyst via the sonochemical method for the degradation of Malachite green under the illumination of visible light. The prepared nanoparticle achieved 91.89% degradation efficiency after 45 min visible light illumination. There are various green syntheses reported on the cuprous oxide (Cu2O) nanoparticles for the photocatalytic degradation of organic pollutants. Kerour et al.182 synthesized Cu2O nanoparticles by a green method using aloe vera leaves plants extracted for the degradation of methylene blue dye and were able to completely degrade methylene blue after 10 min of visible light illumination. Yadav et al.183 used sugarcane bagasse to synthesize ecofriendly Cu2O nanoparticles for the degradation of methyl red, Congo red, methylene blue, and methyl orange organic dyes.Despite the achievement and simple green synthesized Cu2O nanoparticles, the rate of recombination of photogenerated electrons and holes, stability, and photocorrosion are challenging for photocatalytic applications.127 For instance, during the photocatalytic process, when Cu2O is irradiated, it can be converted into CuO through photooxidation, which shows the deactivation of the photocatalytic process.189 To suppress these challenges and improve the photocatalytic activity of Cu2O, different ideas have been generated by researchers. The preparation of Cu2O nanoparticles with good control on the morphology significantly influences its chemical and physical properties in using them as photocatalyst materials.190 Prado-Chay et al.184 synthesized hierarchical Cu2O microspheres by a simple chemical method at room temperature with enhanced photocatalytic activity for the degradation of methyl orange under visible light irradiation.
Coupling with other metal oxides to create heterojunctions is another method to enhance the photocatalytic performance of Cu2O. Wang et al.185 reported ZnO/Cu2O heterojunctions designed by the self-templating method for the degradation of rhodamine B and achieved 96% within 40 min under visible light irradiation. Making composite with carbon-based materials is also another strategy to boost the photocatalytic activity of Cu2O. K. Polat produced a 2D thin film catalyst Cu2O/2D graphene on copper foil having photocatalytic activity under the visible light spectrum.186 Karthikeyan et al.187 reported hierarchical Cu2O nanospheres and Cu2O/rGO nanocomposites for the degradation of 4-chlorophenol and production of H2 under the illumination of visible light, which is attributable to enhanced visible absorption. Z-scheme modification is also the most effective technique to improve the photocatalytic activity of Cu2O. Chen et al.191 synthesized a Z-scheme Cu2O-(rGO-TiO2) photocatalyst for the degradation of 2,2′,4,4′-tretrabromodiphenyl ether. The reported Z-scheme photocatalytic performance by this group was greater than either single or composite of Cu2O and TiO2 under the same reaction condition. Different Cu2O-based photocatalysts are summarized in Table 9.
Table 9 Summary of Cu2O-based photocatalysts for the photodegradation of organic pollutants
Catalysts |
Synthesis methods |
Morphology |
Model pollutant |
Light |
Reaction time |
Degradation after action |
Ref. |
Cu2O |
Sonochemical |
Nanoparticles |
Malachite green |
Visible light |
45 min |
91.89% |
16 |
Cu2O |
Green synthesis |
Nanoparticles |
Methylene blue |
Solar simulator |
10 min |
100% |
182 |
Cu2O |
Biogenically synthesized |
Nanoparticles |
Methyl orange |
Visible light |
80 min |
77.39% |
183 |
Cu2O |
Chemical solution |
Hierarchical nanoparticles |
Methyl orange |
Visible light |
120 min |
76% |
184 |
ZnO/Cu2O |
Self-templating |
|
Rhodamine B |
Visible light |
40 min |
96% |
185 |
Cu2O/graphene/Cu |
Magnetron sputtering |
Thin film |
Hydrogen peroxide |
Visible light |
— |
— |
186 |
Cu2O/rGO |
Self-assembly |
Pompon Dahlia-like |
4-Chlorophenol |
Visible light |
1 h |
95% |
187 |
2.4.5 CuO photocatalyst. Because of its desirable properties, such as easy preparation low cost, and nontoxicity, CuO nanoparticles acquired great interest in the research field such as photocatalysis, solar cells, supercapacitors, biodiesel, water pollutant removal, and electrocatalysis.27 CuO nanoparticles are an outstanding and efficient photocatalytic material for the degradation of organic pollutants. However, similar to Cu2O, CuO also has the limitation that comes from rapid photogenerated recombination and photocorrosion. Hence, researchers have employed various techniques to address these concerns. Among these, the CuO composite with carbon-based materials has attracted great attention. Banu et al.192 synthesized GO/CuO nanocomposite by the hydrothermal method for the photocatalytic degradation of azure-B dye with an efficiency of 95%. The enhanced photocatalytic activity of the prepared nanocomposite was attributed to the small size and high surface area of GO/CuO. Coupling it with Cu2O and graphene oxide also enhances its photocatalytic performance. Zhang et al.193 reported CuO–Cu2O/GO nanocomposite for the degradation of tetracycline and methyl orange and achieved 90% and 95% degradation efficiency after 120 min under visible light irradiation.Coupling with other metal oxide semiconductors also enhances the photocatalytic performance of CuO nanoparticles. Sakib et al.194 synthesized CuO/ZnO nanocomposite through the mechanochemical combustion method for the degradation of methylene blue under visible light irradiation. They achieved methylene blue degradation of 81% and 98% using ZnO and CuO/ZnO, respectively. Forming a p–n junction heterostructure has the advantages of widening the light harvesting and retarding the fast recombination of the photogenerated electrons and holes. Malwal and Gopinath synthesized the 3D hierarchical nanostructured n-type ZnO and Fe3O4 deposited on p-type CuO via thermal oxidation, followed by microwave-assisted deposition for the formation of p–n heterojunctions.195 The prepared p–n heterojunctions enhanced the photocatalytic activity for the degradation of Congo red dye under solar light irradiation. The enhanced photocatalytic performance was attributed to the effective and sufficient charge separation formation of the p–n junction heterostructures at the interface of p-type (CuO) and n-type (ZnO/Fe3O4) semiconductors, as depicted in Fig. 12. The charge separation creates an electric field at the interfaces. For dye degradation, the photosensitization and p–n junction-mediated photocatalytic mechanisms are involved, as shown in Fig. 12.
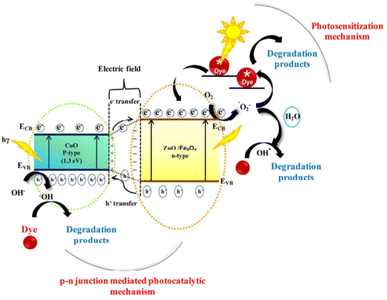 |
| Fig. 12 Proposed mechanisms for the photodegradation of Congo red dye under solar light irradiation using p–n heterojunctions (p-type CuO and n-type ZnO/Fe3O4) semiconductor.195 | |
Metal doping is another effective strategy to improve the photocatalytic performance of CuO. George et al.196 synthesized nanoflower-like 3D transition metal-doped CuO nanostructure by the sonication method for the degradation of methylene blue dye under visible light illumination. The 3D transition metal incorporated into CuO played a great role in tuning the structure, morphology, and optical properties. Maraj et al.197 reported Mo-doped CuO nanoparticles synthesized by the sol–gel process and utilized it for the degradation of methylene blue under visible light irradiation. Islam et al.198 synthesized Ce-doped CuO nanoparticles through the sol–gel autocombustion method and utilized it for the degradation of methylene blue under the illumination of visible light. Ce-doped CuO nanoparticles exhibited better photocatalytic activity than undoped CuO nanoparticles. The general summary of different CuO nanoparticles and CuO-based photocatalysts are summarized in Table 10.
Table 10 Summary of CuO-based photocatalysts for the photodegradation of organic pollutants
Catalysts |
Synthesis methods |
Morphology |
Model pollutant |
Light |
Reaction time |
Degradation efficiency |
Ref. |
CuO |
Mechanochemical |
Nanoparticles |
Methyl green |
Sunlight |
60 min |
65% |
199 |
CuO |
Green synthesis |
Nanoparticles |
Rhodamine B |
UV |
90 min |
98% |
200 |
CuO |
Microwave-assisted |
Nanoparticles |
Methylene blue |
Sunlight |
90 min |
99% |
201 |
GO/CuO |
Hydrothermal |
Lamellar sheets |
Azure-B dye |
Tungsten lamp |
120 min |
95% |
192 |
CuO-Cu2O/GO |
Hydrothermal |
— |
Tetracycline |
Visible |
120 min |
90% |
193 |
CuO-Cu2O/GO |
Hydrothermal |
— |
Methyl orange |
Visible light |
120 min |
95% |
193 |
CuO/ZnO |
Mechanochemical combustion |
Nanoparticles |
Methylene blue |
Solar irradiation |
2 h |
98% |
194 |
ZnO |
Mechanochemical combustion |
Nanoparticles |
Methylene blue |
Solar irradiation |
2 h |
81% |
194 |
ZnO@CuO |
Microwave-assisted |
Hierarchical nanostructures |
Congo red dye |
Visible light |
300 min |
90% |
195 |
Mo-doped CuO |
Sol–gel |
Nanoparticles |
Methylene blue |
Visible light |
120 min |
90% |
197 |
Ce-doped CuO |
Sol–gel |
Nanoparticles |
Methylene blue |
Visible light |
3 h |
99% |
198 |
CuO/g-C3N4 |
Pyrolysis |
Nanosheets |
Salicylic acid |
Visible light |
80 min |
100% |
202 |
ZnO/CuO/g-C3N4 |
Cocrystallization |
Sheet-like |
Methylene blue |
Visible light |
50 min |
97.46% |
203 |
ZnO/CuO/g-C3N4 |
Cocrystallization |
Sheet-like |
Congo red |
Visible light |
50 min |
91.73% |
203 |
2.4.6 SnO2 photocatalyst. Tin dioxide (SnO2) is an n-type semiconductor and has a wide range of applications in areas such as catalysts, gas sensors, transistors, batteries, and transparent electrodes.204 SnO2 in its nanoparticles has a high surface-to-volume ratio that enhances the active sites and is expected to exhibit good photocatalytic activity. Pure SnO2 is rarely studied because of the simultaneous formations of Sn2+ and Sn0 during the photocatalysis process. Thus, it can be presented as SnO2, SnO, or Sn in the catalyst. Moreover, in photocatalytic applications, it is found as a composite form with other semiconductors to suppress instability, and the composite of SnO2 with other semiconductors has been widely reported. SnO2 is also an ultraviolet-active metal oxide semiconductor due to its wide bandgap of 3.6 eV similar to TiO2.205 To date, numerous efforts such as building heterojunctions, morphology control, metal or nonmetal doping, and carbon-based materials composite have been developed to suppress these challenges. G. Devi and R. Shyamala synthesized SnO2 and α-Fe2O3 by the sol–gel method and combined them by mixing and grinding to form a SnO2–α-Fe2O3 composite.206 They studied the photocatalytic activity of the prepared composite using phenol as a pollutant model. Due to the redshifts in the bandgap, the prepared composite showed higher extent absorption in the visible light region.Combining SnO2 with other metal oxide and carbon-based materials also enhances its visible light activity. Yao et al.207 reported SnO2/TiO2/RGO nanocomposite synthesized by the hydrothermal method with enhanced photocatalytic activity under visible light illumination for the degradation of rhodamine B. The superior photocatalytic activity of the synthesized nanocomposite is attributed to the great efficiency of light absorption and outstanding charge separation and transfers. Wang et al.208 synthesized micron-sized spherical SnO2 (Fig. 13a) and flower In2O3 (Fig. 13c) by the hydrothermal method and prepared the In2O3/SnO2 composite by the two-step method. Fig. 13e shows the morphology of In2O3/SnO2, and Fig. 13b, d, and f show the magnified morphology of the SnO2, In2O3, and In2O3/SnO2 composite, respectively. According to this report, the bandgap of the prepared materials in decreasing order is SnO2, In2O3, and In2O3/SnO2, respectively, as presented in Fig. 13g. For the photocatalytic degradation study of the prepared photocatalyst, they used rhodamine B as a model organic pollutant, and the In2O3/SnO2 composite showed the highest degradation rate of 97% after 240 min irradiation (Fig. 13h), which was twice that of pure SnO2 or In2O3. The photocatalytic mechanism of the prepared composite is shown in Fig. 13i. In this report, the superoxide (O2˙−) and holes play a great role in the degradation of rhodamine B, while ˙OH played an auxiliary role. The energy band positions of the two compounds matched well, as shown in Fig. 13i, in which the photogenerated electrons and holes separated effectively. An electron (e−) was generated in the conduction band and a hole (h+) was generated in the valence band.
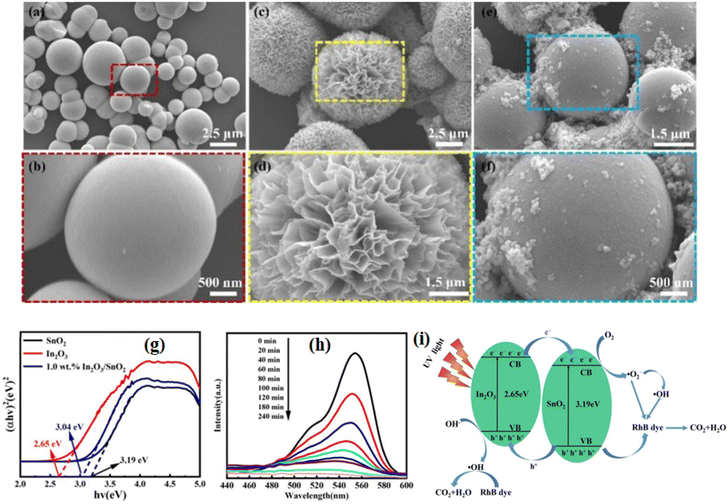 |
| Fig. 13 SEM images: (a and b) of the pure SnO2; (c and d) of the prepared In2O3; (e and f) of the prepared 1.0 wt% In2O3/SnO2 composite photocatalysts; (g) the bandgap; (h) degradation kinetics of rhodamine B. (i) Schematic illustration of the separation and migration mechanism of photogenerated electron–hole pairs of the In2O3/SnO2 composite.208 | |
When the two compounds were irradiated, electrons and holes were generated, as shown in eqn (14) and (15). The photogenerated holes of SnO2 transferred to the valence band of In2O3 (eqn (16)) and the photogenerated electrons of In2O3 transferred to the conduction band of SnO2 (eqn (17)). Thereafter, e− reacts with O2 to form superoxide (O2˙−) (eqn (18)), and the photodegradation process of rhodamine B is shown in eqn (19)–(21).208
|
SnO2 + hν → SnO2(h+)VB + SnO2(e−)CB
| (14) |
|
In2O3 + hν → In2O3(h+)VB + In2O3(e−)CB
| (15) |
|
SnO2(h+)VB → In2O3(h+)VB
| (16) |
|
In2O3(e−)CB → SnO2(e−)CB
| (17) |
|
SnO2(e−)CB + O2 → O2˙−
| (18) |
|
Rhodamine B + O2˙− → H2O + CO2
| (19) |
|
Rhodamine B + h+ → H2O + CO2
| (20) |
|
Rhodamine B + ˙OH → H2O + CO2
| (21) |
Combining SnO2 with noble metals also enhances its photocatalytic activity and its visible light response. Ansari et al.209 synthesized Ag–SnO2 nanocomposites in water at room temperature using electrochemically-active biofilm and utilized it for the degradation of methylene blue, methyl orange, 2-chlorophenol, and 4-nitrophenol under visible light irradiation. The prepared nanocomposite showed enhanced photocatalytic activity compared to pure SnO2. Doping with metals is also an effective method to slow down the photogenerated electron–holes recombination and expand the visible light activity of SnO2. Ran et al.210 reported hollow-structured Ti-doped SnO2 prepared using silica microspheres as templates for the decomposition of methylene blue under UV and visible-light irradiation. Compared with pristine SnO2 hollow spherical, Ti-doped SnO2 showed enhanced photocatalytic activity with 92% rate of degradation under UV light irradiation and 54% under visible light irradiation decomposition of methylene blue within 135 min of degradation time. Ali Baig et al.211 synthesized a Zn-doped SnO2 photocatalyst by the hydrothermal method for the degradation of methylene blue under visible light illumination. The effects of Zn-doping are clearly understood to prolong the photoabsorption to the visible region and decreasing electron–hole recombination. The summary of SnO2-based photocatalysts for the photodegradation are given in Table 11.
Table 11 Summary of SnO2-based photocatalysts for the photodegradation of organic pollutants
Catalysts |
Synthesis methods |
Morphology |
Model pollutant |
Light |
Reaction time |
Degradation efficiency |
Ref. |
SnO2 |
Precipitation |
Nanoparticles |
Methylene blue |
UV |
180 min |
79% |
204 |
SnO2/TiO2/RGO |
Hydrothermal |
Nanoparticle |
Rhodamine B |
Visible light |
120 min |
83.84% |
207 |
In2O3/SnO2 |
Hydrothermal |
Nanoparticle |
Rhodamine B |
Visible light |
240 min |
97% |
208 |
Ag–SnO2 nanocomposites |
Electrochemically active biofilm |
Nanoparticle |
Methylene blue, methyl orange |
Visible light |
6 h for MO and 5 h for MB |
100% |
209 |
Ti-doped SnO2 |
Templates |
Nanoparticle |
Methylene blue |
UV |
135 min |
92% |
210 |
Ti-doped SnO2 |
Templates |
Nanoparticle |
Methylene blue |
Visible light |
135 min |
54% |
210 |
Zn-doped SnO2 |
Precipitation |
Nanoparticle |
Methylene blue |
Visible light |
120 min |
93.03% |
211 |
SnO2/g-C3N4 |
Solution ball milling-baking |
Layer structure |
Trichlorophenol |
Visible light |
5 h |
85% |
212 |
SnO2/GL-C3N4 |
Hydrothermal |
Layer structure |
Rhodamine B |
Visible light |
3 h |
92% |
213 |
SnO2/TiO2 |
Hydrothermal |
Nanoparticle |
methylene blue dye |
Solar light |
50 min |
∼90% |
214 |
La2O3/SnO2 |
Coprecipitation |
Nanoparticles |
Methylene blue |
UV |
70 min |
92% |
215 |
2.4.7 Fe2O3 photocatalyst. Iron oxide can be present in different phases such as maghemite (ν-Fe2O3), magnetite (Fe3O4), hematite (α-Fe2O3), and wustite (FeO). Among these phases, Fe2O3 and Fe3O4 are the most common phases of iron oxide in nature. Because of the easy manipulation of morphology, structures, and dimension orientation to provide stability, Fe2O3 is applied in various industrial applications.118 Fe2O3 has four polymorphic phases, namely, ε-Fe2O3, γ-Fe2O3, β-Fe2O3, and α-Fe2O3 (hematite).102 Among these crystallographic phases, α-Fe2O3 (hematite) is the most stable and widely used in different applications such as sorbents, catalysts, biomedical devices, and solar cells.216 Nanosized α-Fe2O3 has better magnetic behavior (ferromagnetic) than ferum (Fe).217,218 This magnetic behavior helps to separate the photocatalyst materials from the solution after the photocatalytic treatment.Moreover, α-Fe2O3 has been used as a photocatalyst material due to its energy bandgap being appropriate for the photocatalysis process. However, pure Fe2O3 is limited in its application in photocatalysis because of disadvantages such as lower minority charge carriers mobility, sluggish surface kinetics, the short diffusion length of photogenerated carriers, and high recombination rate of photogenerated electron–holes pairs.219 Different strategies have been developed to address these problems. The suitably positioned bandgap of α-Fe2O3 allows it to form a composite with other metal oxides to reduce the recombination of photogenerated electron–hole pairs. Senthil et al.220 reported α-Fe2O3/WO3 composite photocatalyst designed by a simple physical mixing process and utilized it for the photodegradation of methylene blue under visible light irradiation. The prepared composite exhibited excellent photocatalytic activity, which was attributed to the strong absorption of α-Fe2O3/WO3 composite and slow down recombination of electron–hole pairs. The polymer coating is also another method to enhance the photocatalytic activity of α-Fe2O3. Zhang et al.221 synthesized nitrogen-rich crosslinked copolymer, poly(1,4,8,11-cyclotetradecane [2,2-bipyridine]-5,5-dicarboxamine) (PNH). This polymer covered Fe2O3 by moderate hydrothermal treatment to form Fe2O3@PNH, which showed excellent photocatalytic activity for the degradation of tetracycline with catalytic performance of ∼90% under visible light illumination, which was three times greater than that of Fe2O3.
Doping is another effective strategy to address the aforementioned problems that generate electron trap centers. Li et al.219 synthesized different metal-doped Fe2O3 nanoparticles using fabric filter dust as starting materials for the degradation of methyl orange. The different metal-doped materials showed a 82.99% degradation rate under the illumination of visible light, whereas the undoped Fe2O3 achieved only 38.94%. Nonmetal doping can also improve the photocatalytic activity of Fe2O3 nanoparticles. Mendiola-Alvarez et al.222 synthesized phosphorus-doped Fe2O3–TiO2 by the sol–gel method and utilized it for the degradation of sulfamethazine under visible light irradiation. The doped composite has shown improved photocatalytic performance compared to either the Fe2O3–TiO2 composite or TiO2, which was attributed to the reduced bandgap, small crystallite size, increased existence of hydroxyl on the catalyst surface, and high surface area. Graphene is a type of carbonaceous material having excellent transparency, lightweight, high electrical conductivity, unique structure, high adsorption capacity, and high specific surface area properties, which enhances the photocatalytic performance of α-Fe2O3 when it made the hybrid. S. Frindy and M. Sillanpää synthesized α-Fe2O3 hybridized with graphene by the hydrothermal method for the degradation of rhodamine B under visible light irradiation and it showed high catalyst activity of ∼98%.223 They demonstrated that the incorporation of graphene into α-Fe2O3 nanoparticles resulted in high photocatalytic activity compared to pure α-Fe2O3 or α-Fe2O3 loaded on other carbonaceous materials. The summary of Fe2O3-based photocatalysts for photocatalytic degradation is given in Table 12.
Table 12 Summary of Fe2O3-based photocatalysts for photocatalytic degradation
Catalysts |
Synthesis methods |
Morphology |
Model pollutant |
Light |
Reaction time |
Degradation efficiency |
Ref. |
Fe2O3 |
Chemical solution |
Porous nanorods |
Rhodamine B |
Simulated solar light |
270 min |
86.4% |
224 |
α-Fe2O3/WO3 |
Hydrothermal |
Nanoparticles |
Methylene blue |
Visible light |
60 min |
100% |
220 |
Fe2O3@PNH |
Condensed polymerization |
— |
Tetracycline |
Visible light |
120 min |
90% |
221 |
Al and Ti doped Fe2O3 |
From fabric filter dust |
Nanoparticles |
Methyl orange |
Visible light |
180 min |
82.99% |
219 |
P-doped Fe2O3–TiO2 |
Microwave-assisted sol–gel |
Nanoparticles |
Sulfamethazine |
Visible light |
300 min |
100% |
222 |
α-Fe2O3-Gr |
Hydrothermal |
Nanoparticle |
Rhodamine B |
Visible light |
75 min |
98% |
223 |
α-Fe2O3@TiO2 |
Coprecipitation |
Nanocomposites |
Methylene blue |
Visible light |
120 min |
92% |
225 |
TiO2/Fe2O3 |
Hydrothermal |
Nanocomposites |
Cr(VI) |
Visible light |
160 min |
100% |
226 |
α-Fe2O3/g-C3N4 |
Heat treatment |
Nanocomposites |
Diazinon |
UV |
50 min |
100% |
227 |
GO/α-Fe2O3 |
Coprecipitation |
Nanoparticles |
Methylene blue |
Visible light |
90 min |
90% |
228 |
Fe2O3/TiO2 |
Atomic layer deposition |
Nanoparticles |
Methyl orange |
Visible light |
90 min |
97.4% |
229 |
Fe3O4@Bi2O3-RGO |
Self-assembly |
Core–shell |
Ciprofloxacin |
Visible light |
240 min |
98.3% |
230 |
2.4.8 Bi2O3 photocatalyst. Bismuth oxide (Bi2O3) is found in various crystals forms such as triclinic (ω), orthorhombic (ε), face-centered cubic (δ), body-centered cubic (γ), monoclinic (α), tetragonal (β), and hexagonal assembly.21 Bi2O3 has an excellent photocatalytic activity for wastewater treatment, CO2 reduction, nitrogen fixation, and water splitting under the irradiation of visible light solar spectrum. However, Bi2O3 is now confronted with a larger recombination rate of the electron–hole pairs, which significantly reduces its photocatalytic activity for the degradation of organic pollutants.232 To boost the overall photocatalytic efficiency of Bi2O3, many strategies including composition with other materials, morphology, shape, microstructure, surface structure, interface-engineering, oxygen vacancy, and doping with metal and nonmetal have been developed. For instance, when it is coupled with other semiconductors, its photocatalytic activity becomes more favored. Hsieh et al.237 synthesized the Bi2O3/CeO2 composite via the hydrothermal method and compared the photocatalytic performance of pristine Bi2O3, CeO2, and Bi2O3/CeO2 composite, in which the composite showed excellent catalytic performance under visible light illumination for the degradation of Orange II dye. The CuCo2S4/Bi2WO6 heterojunction used for the degradation of tetracycline hydrochloride has also reported by Wang et al.245Doping is also an effective and efficient strategy to reduce the large recombination rate of electron–hole pairs. Li et al.232 reported the La-doped Bi2O3 prepared via the sol–gel method in which La3+ doping creates oxygen vacancies on the surface of Bi2O3 and also as an efficient scavenger to capture the photoinduced electrons. The prepared material was used for the degradation of methyl orange (MO) and phenol and showed an enhanced photocatalytic activity, which was attributed to the strong synergistic effects of La3+ doping and oxygen vacancies (OVs), as shown in Fig. 14. According to this report, the photocatalytic mechanism for the degradation of methyl orange and phenol are as follows.
|
La-Bi2O3 + hν → h+ + (e−)CB
| (22) |
|
La-Bi2O3 + hν → h+ + (e−)OVs
| (23) |
|
La3+(5d06s0) + (e−)OVs → La2+(5d06s1) + h+
| (24) |
|
La2+(5d06s1) + O2 → La3+(5d06s0) + O2˙−
| (25) |
|
MO/phenol + O2˙−/h+ → CO2 + H2O +…
| (26) |
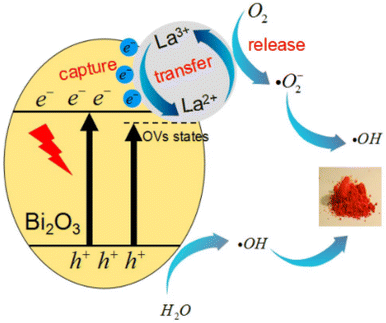 |
| Fig. 14 Proposed schematic photodegradation mechanisms for MO over 2% La-Bi2O3 sample under visible light irradiation.232 | |
Singh and Sharma also reported the Ni-doped Bi2O3 synthesized by the chemical precipitation method.233 They used the doped photocatalyst for the degradation of methylene blue under the illumination of visible light, and Ni dopant served as a trapping energy site for the photogenerated charge carriers, which resulted in greatly enhanced photocatalytic activity of the Bi2O3 photocatalyst. Different Bi2O3-based photocatalysts are summarized in Table 13.
Table 13 Summary of Bi2O3 based photocatalysts for photodegradation of organic pollutants
Catalysts |
Synthesis methods |
Morphology |
Model pollutant |
Light |
Reaction time |
Degradation efficiency |
Ref. |
α-β Bi2O3 |
Solvothermal |
Nanoparticles |
Rhodamine B |
Visible light |
120 min |
99.6% |
231 |
La-doped Bi2O3 |
Sol–gel |
Nanoparticles |
Methyl orange |
Visible light |
100 min |
98% |
232 |
La-doped Bi2O3 |
Sol–gel |
Nanoparticles |
Phenol |
Visible light |
100 min |
73% |
232 |
Ni-doped Bi2O3 |
Chemical precipitation |
Nanoparticles |
Methylene blue |
Visible light |
270 min |
81% |
233 |
Bi2O3/Bi2O4 |
Calcining raw materials |
Flower-like nanoparticles |
17α-ethinyl estradiol |
Visible light |
12 min |
∼100% |
234 |
TiO2/Bi2O3 |
Template |
Nanoparticles (hollow) |
Tetracycline |
Visible light |
140 min |
100% |
235 |
Cs2O–Bi2O3–ZnO |
Combustions |
Nanoparticles (sponge-like structure) |
4-Chlorophenol |
Simulated sunlight |
80 min |
98.5% |
236 |
Bi2O3/CeO2 |
Hydrothermal |
Nanoparticles |
Orange II dye |
Visible light |
5 h |
— |
237 |
Bi2O3/g-C3N4 |
Heat treatment |
Nanoparticles (spheroidal) |
Amido black 10B dye |
Xenon |
120 min |
88.71% |
238 |
2.4.9 Pb-based oxide photocatalysts. Lead oxide has attracted attention due to its good reactivity, cost-effectiveness, experimental simplicity, and recyclability.40,246 Lead oxide exists in Pb4+ (PbO2) and Pb2+ (PbO) oxidation states. PbO2 is mainly used as electrode materials in lead–acid battery, which is used in motor vehicles as internal combustion engines.247 On the other hand, PbO2 and PbO possess good chemical stability, optical transparency, and micro-hardness, which make them suitable for optoelectronic industry applications.241,248,249 Lead oxide can also be found in the form including Pb3O4, PbO2, Pb2O3 (α, β, and amorphous), and PbO (α, β).250 PbO nanoparticles have a wide range of applications including energy storage devices, luminescence materials, gas sensors, modifiers in glass, lead crystals, pigments, nanoelectronic devices, lead glazes, UV blockers, pigments, and in decorative potteries.37,41,251–253 It was also used as a shielding material for gamma radiation protection.254Lead oxide nanoparticles can also be used in photocatalytic degradation.31–33 However, for photocatalytic applications, PbO is not reported alone but coupled PbO with other semiconductors have been widely reported. The PbO composite with different metal oxide semiconductors has advantages such as shifting the ultraviolet activity of materials to visible light activity by controlling the bandgap and modifying the structure and morphology of the newly formed composite photocatalyst. For instance, Bhachu et al.239 deposited PbO nanoclusters onto titanium dioxide (anatase) polycrystalline films on a glass substrate by the chemical vapor deposition method to form the PbO/TiO2 composite. The PbO-modified TiO2 introduced new electronic states above the valence band maximum of TiO2, which resulted in the enhancement of the photocatalytic activity of TiO2 in the visible light region. Leelavathi et al.240 designed PbO quantum dot dispersed on anatase TiO2 to form heterostructures by combining nonhydrolytic sol–gel and combustion methods for the separation of photogenerated charge carriers. They observed that the bare PbO showed very poor photocatalytic activity. They obtained 77% and 50% of the photodegradation percentage of orange G for TiO2/PbO and TiO2, respectively, within 2 h.
PbO has also been used in different metal oxide nanoparticles to modify their photocatalytic activity. Mebed et al.241 prepared PbO–Al2O3 nanocomposite by the microwave irradiation method and utilized it for the removal of methylene blue from wastewater under UV-visible or visible light irradiations. Al2O3 possesses a very wide bandgap of approximately 9 eV. However, compositing it with PbO made it responsive to visible light irradiation and improves the overall physicochemical properties. Wang et al.242 reported Sb2O3/PbO-coupled semiconductor photocatalyst synthesized by the hydrothermal method for the degradation of carbamazepine (CBZ). They selected PbO for coupling with Sb2O3 due to its high stiffness, high thermal stability, and high tensile strength. Wang et al.5 synthesized PbO/TiO2 and Sb2O3/TiO2 photocatalysts for the degradation of UV filter benzophenone-3 under UV-C irradiation, and PbO/TiO2 completely degraded the UV filter benzophenone-3 within 120 min. They studied the effect of pH by varying it from 3 to 11, and the degradation efficiency at varied pH is shown in Fig. 15a. The degradation rate increased as the pH value increased from 3 to 9 for the photocatalyst with PbO ratio with TiO2 (1
:
2). However, the degradation rate slightly decreased as pH increased to 11 compared to 9. Fig. 15b represents the schematic of the photocatalytic mechanism of PbO/TiO2 nanocomposite. Reactive holes (h+) generated on the surface of PbO and reactive electrons (e−) generated on the surface of TiO2 under UV-C irradiation. The photogenerated electrons reduced the dissolved O2 to superoxide radicals (O2˙−). Then, O2˙− radicals undergo the oxidation process with the photogenerated h+ to produce 1O2, as shown in eqn (27)–(29).
|
PbO/TiO2 + hν → TiO2(e−) + PbO(h+)
| (27) |
|
(TiO2)e− + O2 → O2˙−
| (28) |
|
(PbO)h+ + O2˙− → 1O2
| (29) |
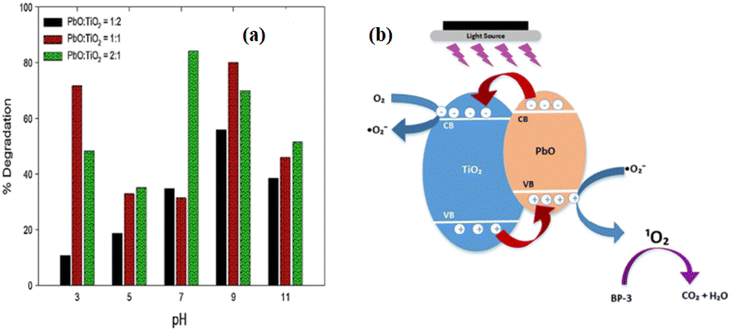 |
| Fig. 15 (a) The photocatalytic degradation of BP-3 by varying the initial pH PbO/TiO2; (b) mechanism of photocatalytic degradation of BP-3.5 | |
Pb-containing metal oxides photocatalyst have also been reported by different groups. For instance, Pourmasoud et al.71 reported PbWO4 synthesized by the coprecipitation method for the degradation of methyl orange from wastewater under UV irradiation and achieved a 97% rate of degradation. Gyawali et al.98 reported an Ag-PbMoO4 photocatalyst synthesized by the sonochemical method for the degradation of Indigo carmine dye under simulated solar light irradiation.
Perovskites containing Pb in their compositions have a potential application in solar energy conversions, but recently, they were studied to use be as photocatalysts. In photocatalysis, perovskites are used as means of energy production and water purification.255 The combination of PbO with perovskite photocatalysts has also been reported by different scholars. Liu et al.243 synthesized the PbBiO2I/PbO nanocomposite using the hydrothermal method and used it as a photocatalyst for the degradation of crystal violet (CV) dye. According to this report, the formation of a composite between PbBiO2I and PbO suppresses the rapid recombination of photogenerated electrons–holes pairs and improves the photocatalytic activity of the individual PbBiO2I and PbO by 3 and 20 times, respectively. The charge carrier transfers and photocatalytic mechanism of the prepared PbBiO2I/PbO are shown in Fig. 16. As one can observe from the depicted Fig. 16, the valence band maximum (VBM) and conduction band minimum (CBM) of PbBiO2I was higher than that of PbO. Therefore, the photogenerated electrons that occur on the surface of PbBiO2I could be transferred to the PbO conduction band, whereas holes existing on the surface of PbO can transfer to PbBiO2I. Thus, the charge transfer between the two semiconductors effectively hinders electron–hole recombination, hence improving the photocatalytic efficiency. When electrons and holes reached the CB and VB of PbBiO2I/PbO, the active oxygen species, which degrades the CV, is formed (eqn (30) and (31)). As observed in Fig. 16, in the photosensitized and photocatalytic process, photogenerated and photosensitized electrons react with O2 on the surface of the photocatalyst and produced superoxide (O2˙−). 1O2 is also generated upon the reaction between O2 and photocatalyst. The combination of perovskites, PbO, and other semiconductors to form heterojunctions has also been reported. Wang et al.256 synthesized PbBiO2Br/PbO/g-C3N4 heterojunctions by the hydrothermal method without using a template, and they demonstrated that the enhancement of the photocatalytic activity of this photocatalyst corresponded to the heterojunctions formed between PbBiO2Br, PbO, and g-C3N4. Thus, PbO contributes to the enhancement of the photocatalytic activities of this heterojunction.
|
(organic pollutant)CV + /1O2/h+ → degraded compounds
| (30) |
|
(organic pollutant)CV+˙ + O2˙−/1O2/h+ → degraded compounds
| (31) |
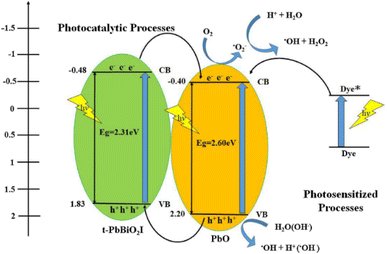 |
| Fig. 16 Schematic illustration of the bandgap structures of t-PbBiO2I/PbO.243 | |
Pb-containing composition of the compounds has also been used for the removal of microbiological contaminants from drinking water. Li et al.257 synthesized Pb-BiFeO3/rGO photocatalyst via the hydrothermal method and evaluated the photocatalytic disinfection efficiencies toward Gram-negative Escherichia coli (E. coli) and Gram-positive Staphylococcus aureus (S. aureus) under the illumination of visible light. According to their report, the complete inactivation of E. coli and S. aureus was achieved within 30 min and 90 min, respectively. In this report, the intercalated Pb coordinate with Bi3+ was to provide actives sites for the reaction, hence improving the photocatalytic activity of BiFeO3. Different Pb-based oxide photocatalysts for the photocatalytic degradation of organic pollutants are summarized in Table 14.
Table 14 Summary of Pb-based photocatalysts for the photodegradation of organic pollutants
Catalysts |
Synthesis methods |
Morphology |
Model pollutant |
Light |
Reaction time |
Degradation efficiency |
Ref. |
PbO/TiO2 |
Chemical vapour deposition |
Films |
Stearic acid |
Visible light |
71 h |
70% |
239 |
TiO2/PbO |
Sol–gel combustion |
Nanocomposites |
Orange G |
Metal halide lamp |
2 h |
77% |
240 |
PbO–Al2O3 |
Microwave irradiation |
— |
Methylene blue |
UV |
105 min |
28% |
241 |
Sb2O3/PbO |
Hydrothermal |
— |
Carbamazepine |
UV |
120 min |
— |
242 |
PbO/TiO2 |
— |
— |
Benzophenone-3 under UV |
|
120 min |
100% |
5 |
PbWO4 |
Coprecipitation |
Nanoparticles |
Methyl orange |
UV |
100 min |
97% |
71 |
Ag-PbMoO4 |
Sonochemical |
Nanoparticles |
Indigo carmine |
Simulated solar light |
120 min |
— |
98 |
PbBiO2I/PbO |
Hydrothermal |
— |
Crystal violet |
Visible light |
24 h |
99% |
243 |
Sn doped α-PbO |
Chemical precipitation |
Nanoparticles |
Methylene blue dye |
Visible light |
100 min |
∼100% |
244 |
2.4.10 Density functional theory (DFT). DFT is a modern calculation widely used in fields such as condensed matter physics, quantum chemistry, and materials science, and it is a practical electronic-structure calculation computational method.198 DFT is primarily a theory of electronic structure calculations at ground states couched in terms of electron density.258 The DFT framework provides a computationally easy and controllable way to solve and approximate many-body problems for electrons both in the excited state and ground state.259 DFT simulation is applied for the study of metal oxides and to understand the improvement of the properties of metal oxides by applying different strategies for different technological applications. To understand the enhancement of metal oxide properties by the DFT method, strategies such as doping with metal or nonmetal, oxygen vacancy, and coupling with other semiconductors to form heterojunctions are among the intensively studied. For instance, Iwaszuk and Nolan studied the PbO and PbO2 nanoclusters supported on TiO2 anatase (001) and rutile (110) surface by the first principles DFT method for the modification of the TiO2 photocatalyst.260 They investigated the effects of Pb oxidation states on the PbOx modifications of TiO2 photocatalysts, which showed a dramatic impact on the nature of band-edge modification of TiO2 and on the electron–hole separation mechanism. New states were introduced in the pure rutile and anatase when PbO and PbO2 was adsorbed on the surface of TiO2. Thus, the adsorbed PbO and PbO2 nanoclusters induced the visible-light activation of the TiO2 photocatalyst and predicted the reduction of fast charge recombination.Islam et al.198 used DFT calculation to investigate the effects of Ce doping into the CuO photocatalyst. After replacing Ce in the place of Cu, the crystal structure of CuO was distorted, and the symmetry of the system was reduced. Due to the distortion of the structure and lack of symmetry, the centers of the negative and positive charges can get separated. Thus, the photocatalytic performance of the Ce-doped CuO was enhanced due to the reduced recombination of the photogenerated electron–hole pairs. The Ce 4f orbital greatly affected the valence and conduction band by introducing defect states near the valence and conduction band. The introduced defect state trapped the photogenerated charges and reduced the fast recombination of photogenerated electrons and holes. They also calculated the real part and imaginary part of the dielectric constant and concluded that the higher the real part of the dielectric, the lower the recombination of photogenerated electrons and holes.
Kitchamsetti et al.261 also used first-principles DFT calculation to identify the formation of O2 radicals on the surface of NiO (110), which was believed to initiate the degradation of rhodamine B dye. Huang and Hart studied the capability of photocatalytic water splitting of various tungstate oxides by DFT calculations.262 Singh and Sharma used DFT calculations to investigate the effects of Ni doping into α-Bi2O3 on its photocatalytic activities.233 Pure α-Bi2O3 possesses a direct bandgap, as shown in Fig. 17a. However, after Ni was doped, an indirect bandgap bandstructure was obtained, as shown in Fig. 17b. Thus, the Ni-doped α-Bi2O3 has an increased charge separation and retarded electron–hole pair's recombination, which was attributed to the time elongation for the charge transit conduction band to the valence band.
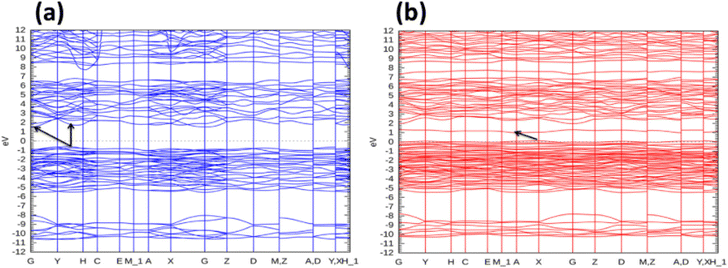 |
| Fig. 17 Electronic bandstructure of (a) α-Bi2O3 (b) Ni doped α-Bi2O3 at 300 K.233 | |
Different semiconducting heterojunctions are also studied using DFT to obtain a better understanding of its photocatalytic activities. The construction of heterojunction structures is among the most effective method for improving the efficiency of photocatalysts by accelerating the separation and transport of the electron–hole. When the heterojunction is formed, a potential difference is created between the conduction band and the valence band at the interface. When irradiated electrons can be easily excited, they immediately form electron–hole pairs. Hence, the photogenerated electrons are transferred to the conduction band of other semiconductors rather than recombining with the holes in the valence band. Yao et al.263 reported the DFT studied g-C3N4/BiOBr(001) heterojunction for a better understanding of its photocatalytic activity by analyzing the electronic and geometric structures. According to their study, they presented ultrathin g-C3N4/BiOBr(001) heterojunction formed by stacking g-C3N4 on the top of BiOBr with 3.55 Å of the interlayer distance, as shown in Fig. 18a. In the complete relaxation of the heterojunction, the interaction between the g-C3N4 monolayer and the surface of BiOBr resulted in g-C3N4 flakes with 2.95 Å interlayer distance, which is the van der Waals distance, as shown in Fig. 18b. Fig. 18c shows the electron localization function of the relaxed g-C3N4/BiOBr(001) heterojunction. The strongest position of electron localization is shown by the yellow-colored area. To investigate the reason for a high photocatalytic activity, they calculated the density of states, as shown in Fig. 18d. The optical bandgap of g-C3N4 and BiOBr was 2.7 eV and 2.8 eV, respectively. However, the bandgap of the g-C3N4/BiOBr(001) heterojunctions was reduced to 1.68 eV, which indicated the high efficiency of charge transfer from the valence band to the conduction band g-C3N4/BiOBr. As shown in Fig. 18e, the valence band maxima (VBM) of BiOBr(001) is lower than that of g-C3N4, whereas the conduction band minima (CBM) g-C3N4 is higher than that of BiOBr(001), as shown in Fig. 18f, which indicates that the g-C3N4/BiOBr(001) heterojunction has a typical type-II energy band alignment. This is reasonable for the greater photoresponse to visible light of the g-C3N4/BiOBr(001) heterojunction compared to g-C3N4 and BiOBr(001).
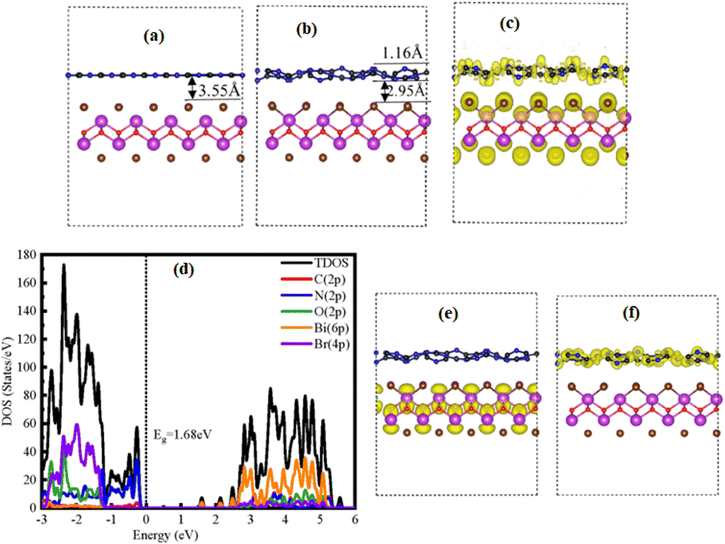 |
| Fig. 18 (a) The heterojunction structure of g-C3N4/BiOBr(001) before geometry optimization and (b) after geometry optimization; (c) the electron localization function of g-C3N4/BiOBr(001); (d) the calculated TDOS and PDOS of the g-C3N4/BiOBr(001) van der Waals heterojunctions; (e) partial charge density of the valence band maxima (VBM) for g-C3N4/BiOBr(001) heterojunction and (f) the conduction band minima (CBM) for the g-C3N4/BiOBr(001) heterojunction.263 | |
Moreover, the energy band structure before and after the formation of the heterojunction is shown in a schematic diagram in Fig. 19.263 As shown in the left of Fig. 19, the energy band edges of both g-C3N4 and BiOBr(001) are given before contact formation. Electrons excited from the VB to the CB for g-C3N4 and the BiOBr(001) layers under visible light irradiation. As shown in the right of Fig. 19, the g-C3N4/BiOBr(001) heterojunction absorbs visible light and electrons excited from the VB to CB, leaving holes behind in the valence band. Some of the photogenerated electrons by the g-C3N4 layer were transferred to the CB of BiOBr(001), while some of the photogenerated holes by BiOBr(001) were transferred to the VB of g-C3N4. This process resulted in the effective separation of photogenerated electrons and holes at the different surfaces by the built-in electric field. Thus, the fast recombination of photogenerated electrons and holes is reduced, and the photocatalytic performance will be improved efficiently. Oktavianti et al.264 also used the DFT method to study the adsorption of methylene blue dye on Ni-doped ZnO clusters. They investigated the adsorption interaction between methylene blue dye and pure ZnO or Ni-doped ZnO in terms of bond length, energy gap, adsorption energy, and Mulliken charge transfer to evaluate the adsorption of ZnO or Ni-doped ZnO toward methylene blue dye.
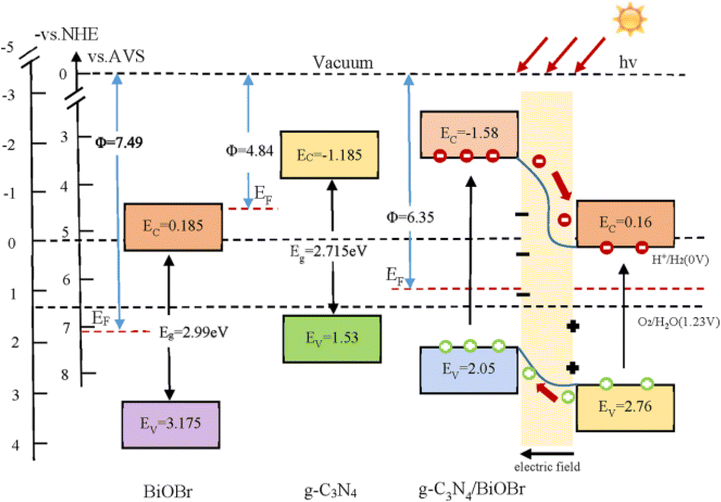 |
| Fig. 19 Schematic diagram of the energy band edge positions before and after the contact between g-C3N4 and BiOBr(001) and the charge separation at the interface of C3N4/BiOBr(001) under visible light irradiation, where Φ is the work function, EC is the bottom of the CB, EV is the top of the VB, and Eg is the bandgap.263 | |
2.5 Parameters effecting the photocatalytic degradation
2.5.1 Effect of pH. The pH of the reaction in the photocatalysis process significantly affects the photodegradation rate of pollutants. The pH of the reaction solution affects the photocatalysts' surface charge, adsorption of contaminants to the photocatalyst's surface, the degree of ionizations, photocatalysts valence band oxidation potential, and agglomeration.265 If the pH of the solution increases beyond the isoelectronic point of the catalyst, the negative charge is induced onto the photocatalyst surface and the negatively charged photocatalyst surface attracts cationic pollutants from the aqueous solution. This affects the concentration of electrons transferred between the catalysts and pollutants for degradation. However, if the pH decreases below the zero-point charge of the photocatalyst, the photocatalyst surface is positively charged and attracts anion pollutants. Adeel et al.6 varied the pH of the solution in the range of 2–10 by fixing the CoZnO dosage to 0.05 g and methyl orange solution to 100 mg L−1. Below the pH point of zero charge, the surface of ZnO is negatively charged, whereas above the point of zero charge, it is positively charged. Methyl orange is an anion in acidic conditions; therefore, the lower pH value is favored for the adsorption of methyl orange on the surface of positively-charged ZnO surface due to electrostatic attraction. The high percentage of methyl orange is degraded at lower pH, whereas the percentage of degradation decreased at higher pH due to electrostatic repulsion. At very low pH, the strongly acidic condition dissolves ZnO; hence, the percentage degradation decreases.Shelar et al.266 studied the effects of pH on the degradation of methylene blue dye of 50 mg L−1 concentration in the pH range from 2 to 12 in the presence of 1 g L−1 Ag-doped ZnO photocatalyst, as shown in Fig. 20. The photodegradation efficiency increases as the pH value increases from 2 to 8. At pH 8, the highest photodegradation efficiency is observed. However, as the pH value further increases from 9 to 12, the photodegradation efficiency decreases, as observed in Fig. 20. Thus, pH 8 is the optimum value for the highest degradation of methylene blue dye for the Ag-doped ZnO photocatalyst. At pH of zero-point charge = 7.6, the catalyst has zero charges; hence, the surface catalyst becomes positively charged at pH less than 7.6 and becomes negatively charged at pH greater than 7.6. Methylene blue dye is a cation dye; therefore, it is attracted to the surface of negatively-charged catalysts for the photodegradation to be higher. For a pH greater than 8, the photocatalytic degradation is decreased because there is electrostatic repulsion between the negatively charged catalyst surface and hydroxide anions. This could inhibit the generation of ˙OH radicals, hence decreasing the percentage degradation of methylene blue dye.
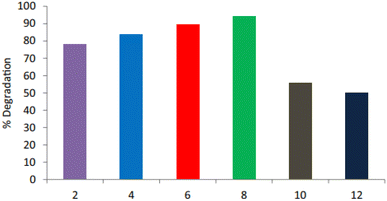 |
| Fig. 20 Effect of pH on the photocatalytic degradation of Methylene blue dye.266 | |
Mohagheghian et al.267 investigated the effects of pH on the percentage degradation of Acid Orange 7 (10 mg L−1) using tungsten oxide nanopowder (1 g L−1) by varying the pH values from 3 to 9, as shown in Fig. 21. At pH zero, point charge was 5.09 and pH greater than 5.09 makes the catalyst surface negatively charged, whereas pH lower than 5.09 makes the surface of catalyst positively charged. The negatively charged surface prevents the adsorption of anion dyes and hydroxide anions onto the catalyst surface. Therefore, the highest degradation efficiency of Acid Orange 7 was observed at pH 3.
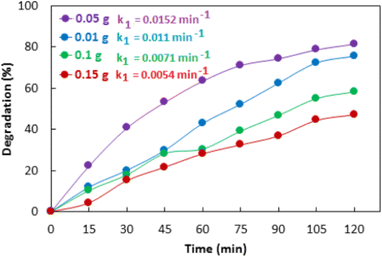 |
| Fig. 21 Effect of pH on the photocatalytic degradation of Acid Orange 7.267 | |
2.5.2 Effect of catalyst dosage. Photocatalyst dosages also have significant effects on the photodegradation rates of pollutants. To avoid the unnecessary utilization of photocatalysts, optimizing the amount of catalyst is essentially important. By increasing the catalyst dosage, the amount of photodegradation of dye increases. Thus, the number of active species increases with increasing catalyst dosage. Beyond a certain limit of catalyst dosage, the aqueous solution becomes cloudy and blocks the light radiation, and the photodegradation efficiency of the dye decreases. Adeel et al.6 reported Co–ZnO synthesized by the precipitation method and utilized for the degradation of methyl orange under visible light. They reported the effects of catalyst dose, pH, temperature, and dye concentration on the percentage degradation of methyl orange. Among these parameters, the effect of catalyst dosage is shown in Fig. 22. They varied the catalyst dosage in the range of 0.01–0.15 g for the photodegradation of 100 mg L−1 concentration methyl orange for 120 min. As shown in Fig. 22, the percentage degradation of methyl orange increases as the catalyst dosage increases from 0.01 to 0.05 g and decreases as the catalyst dosage further increases. The decrease in the percentage degradation while the catalyst dosage increases is attributed (i) to the nonhomogeneity of the reaction mixture at a given speed of agitation and (ii) inhibits penetration light to the interior of the solution. Hence, the number of active centers is nonaccessible; consequently, the percentage degradation decreases. Among all catalyst dosages, 0.05 g obtained the largest kinetic rate constant; therefore, it is the optimum dosage for the photodegradation of methyl orange with 100 mg L−1 concentration.
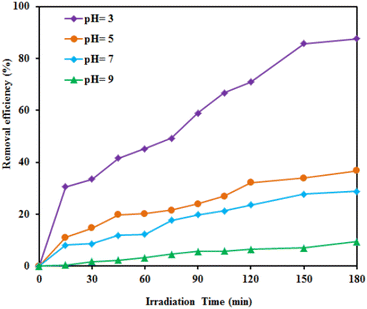 |
| Fig. 22 Effect of the catalyst dose on the photodegradation of methyl orange catalyzed by Co–ZnO.6 | |
2.5.3 Effect of dye concentration. The photocatalysis process is dependent on the pollutants adsorbed onto the photocatalyst surface. In photodegradation, only the amount of dyes adsorbed onto the surface of the photocatalyst contributes to the photocatalysis process.268 As the concentration of the dye increases, large amounts of dyes are adsorbed onto the photocatalyst surface, while less number of lights reach the photocatalyst surface. Thus, lesser number of active oxidation species is generated, and the photodegradation efficiency decreases. I. Ahmad synthesized different metals (Al, Mg, Ni, Cu, and Ag)-doped ZnO/g-C3N4 via a one-pot pyrolysis technique and utilized it for the degradation of EBT dye.169 He named his samples as pure ZnO, g-C3N4, Al-doped ZnO/g-C3N4, Mg-doped ZnO/g-C3N4, Ni-doped ZnO/g-C3N4, Cu doped ZnO/g-C3N4, and Ag-doped ZnO/g-C3N4 with sample coding PZO, GCN, AZGC1, MZGC2, NZGC3, CZGC4, and AZGC5, respectively. He studied the effects of different parameters such as different catalyst effects, catalyst dosage effects, and effects of dye concentration on the photodegradation efficiency of EBT dye. He evaluated the photocatalytic performance as a function of EBT dye by varying concentrations 50–300 mg L−1 while keeping other experimental conditions constant. As shown in Fig. 23, the percentage of photodegradation decreases as the dye concentration increases in all the samples. Thus, the adsorption of a large number of dyes on the photocatalyst surface decreases the adsorption of OH− and also decreases the penetration length of light, which consequently decreases the generation of ˙OH radical, hence decreasing the percentage degradation.
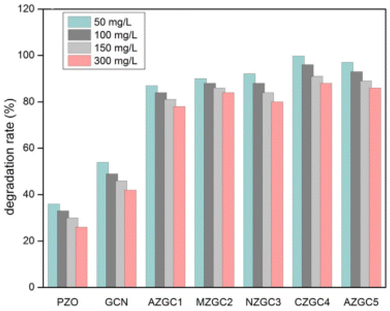 |
| Fig. 23 Effect of dye concentration on photocatalytic EBT dye degradation catalyzed by metals-doped ZnO/g-C3N4.169 | |
2.5.4 Effect of irradiation time. It is obvious that if irradiation time increases the rate of degradation efficiency increases. Fig. 24 shows the percentage degradation of methylene blue dye under UV light irradiation time from 0 to 150 min in the presence of ZnO-Fex (x & 0, 0.05, 0.075, 0.1 mol%) nanoparticles. In Fe dopant concentration, the percentage degradation of methylene blue dye increases as the irradiation time increases.269 Among all the doped materials, Fe (0.075%)-doped ZnO shows the highest degradation efficiency in a short time compared to other Fe dopant concentrations.
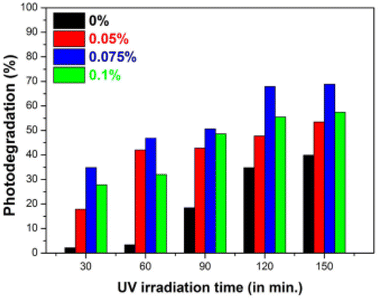 |
| Fig. 24 Influence on the photodegradation of methylene blue dye Fe-doped ZnO catalyst at different irradiation times.269 | |
2.5.5 Effect of oxidizing agent. Oxidizing agents such as ammonium persulphate, potassium bromate, and hydrogen peroxide have a great influence on the photocatalytic degradation of organic pollutants.270 Saquib et al.271 demonstrated the influence of H2O2, KBrO3, and (NH4)2S2O8 oxidizing agents on the photocatalytic degradation of Patent Blue VF and Fast Green FCF in the presence of titanium dioxide (Hombikat UV100 and Degussa P25) as the photocatalysts. Their result revealed that Hombikat UV100 photocatalyst had more efficient degradation for Fast green FCF in the presence of KBrO3 and (NH4)2S2O8 as oxidizing agents, whereas Degussa P25 for the better degradation of Patent Blue VF. They used these three chemicals as electron acceptors.
2.5.6 Effect of intensity of light. The intensity and wavelength of light influence the photocatalytic degradation of dyes in aqueous solution in the presence of photocatalysts.272 For a photocatalytic reaction to take place, the semiconductor used as a photocatalyst must absorb light to initiate the reaction. The bandgap of the photocatalyst should be equal to or greater than the irradiated light. With the increase in the intensity of light, there is a chance for more electrons to be generated, which increases the number of oxidation species. The irradiated light is either UV or visible light. The UV light has high intensity and is hugely applied in the photocatalytic degradation of textile dyes. It is more utilized for photocatalysts with wide optical bandgap such as TiO2 and ZnO. However, due to its cost-effectiveness and abundance, visible light is used as an alternative to UV light. Neppolian et al.273 used both UV and visible for the degradation of Reactive Red 2, Reactive Yellow 17, and Reactive Blue 4 dyes catalyzed by TiO2. They identified that UV irradiation was more effective than solar irradiation in dye degradation because UV has larger energy than solar light; hence, the electron–hole recombination is suppressed in UV irradiation. Galedari et al.274 varied the intensity of light from 16 to 48 W to examine the influence of light intensity on the degradation of methylene blue dye using ZnO@SiO2 photocatalyst under UV light irradiation. They found an increase in the photodegradation efficiency with an increase in illumination intensity and wavelength of the light source, as shown in Fig. 25. The increase in the degradation efficiency with the intensity of light can be due to the efficiency number of electrons stimulated, which leads to an increase in the generation of hydroxyl radicals.
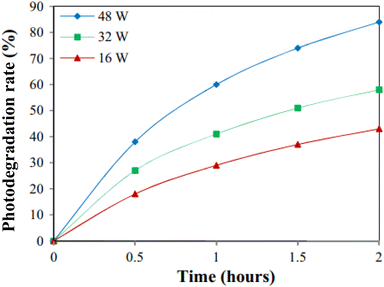 |
| Fig. 25 Effect of light intensity on the photocatalytic degradation rate of phenol using the ZnO@SiO2 photocatalyst.274 | |
3 Conclusion and future perspective
In this review, various metal oxide nanoparticles studied by experimental and computational methods for photocatalysis applications are comprehensively overviewed. It is obvious that in this century, the energy crisis and environmental pollution are the main problems the world faces. Nevertheless, researchers around the world are searching for a way to solve these two problems. Photocatalysis technology is a promising technique to overcome the energy crisis and environmental pollution due to its simple, ecofriendly, and green method. In photocatalytic technology, metal oxides are used as a catalyst for the breakdown or decomposition of different organic contaminants, various dyes, and harmful viruses and fungi using ultraviolet or visible light solar spectrum irradiations. One of the attractive properties of oxide materials for photocatalysis is their stability in various conditions. In addition to this, their bandgap can be easily engineered using simple techniques such as doping, composite, and heterojunction, which makes them highly selective for photocatalytic applications. Generally, their light absorption properties, biocompatibility, stability, electronic structure, charge transfer behaviors, and capability of generating charge carriers of the metal oxides have made them selective for photocatalytic applications. Despite the successful achievements of using metal oxides as a photocatalyst, the major drawbacks including the high recombination rate of photogenerated electron–hole pairs, the ultraviolet light activity limitations, and low surface coverage are the bottlenecks. Various strategies such as doping with metals or nonmetals, combining photocatalysts with other materials, morphology controlling, immobilizing with polymer or noble metals, Z-scheme heterostructures, and compositing with carbon-based materials are used to solve the aforementioned drawbacks. However, further work is needed to improve the existing or to discover new materials for photocatalytic applications. In addition to this, among the listed strategies, the most effective one for the practical application of photocatalysis is needed to be identified. On the other hand, the ecotoxicity of the photocatalysts and the clear separation of the photocatalysts from the treated water need more attention. Another issue that needs to be identified is the type of reactive species during the photocatalytic activity. Thus, many articles used scavengers to identify the type of active species that participated in the photocatalytic degradation process; however, it needs more investigation for the identification of active species during the photodegradation process. Generally, for organic pollutant degradation, an important concern that needs to be addressed is improving the response under the irradiation of visible solar light. Thus, for the degradation of organic contaminants efficiently, economically viable, greener, and faster approaches required a significant development in the sustainable degradation of organic pollutants and creating a clean environment for living creatures. Finally, the density functional theory (DFT) method can be used to understand and discover new materials for photocatalytic applications because it is a simple method to search for new materials in photocatalytic applications without using chemicals as raw materials that reduce the cost. Therefore, DFT is recommended to search for new materials that will be used as photocatalyst materials or predicting the way to improve the discovered metal oxides by understanding the properties of materials. Finally, since metal oxide-based heterojunction photocatalysts are showing promising efficiency under visible light, it is suggested that researchers should develop and design new oxide-based heterojunction photocatalysts for photocatalytic degradations.
Author contributions
Fikadu Takele Geldasa: conceptualization and writing – original draft. Mesfin Abayneh Kebede: supervision, Megersa Wodajo Shura: provisioning resources. Fekadu Gashaw Hone: conceptualization, supervision and editing.
Conflicts of interest
There are no conflicts to declare.
Acknowledgements
We acknowledge Adama Science and Technology University and Oda Bultum University for their financial support. Dr Fekadu Gashaw thanks Addis Ababa University for thematic research project (Grant ref. LT/PY-242/2021) grant.
References
- U. Qumar, J. Z. Hassan, R. A. Bhatti, A. Raza, G. Nazir, W. Nabgan and M. Ikram, J. Mater. Sci. Technol., 2022, 131, 122–166 CrossRef.
- A. Touati, T. Hammedi, W. Najjar, Z. Ksibi and S. Sayadi, J. Ind. Eng. Chem., 2016, 35, 36–44 CrossRef CAS.
- N. Olama, M. Dehghani and M. Malakootian, Appl. Water Sci., 2018, 8, 1–12 CrossRef CAS.
- A. Kushniarou, I. Garrido, J. Fenoll, N. Vela, P. Flores, G. Navarro, P. Hellín and S. Navarro, Chemosphere, 2019, 214, 839–845 CrossRef CAS.
- Z. Wang, A. Deb, V. Srivastava, S. Iftekhar, I. Ambat and M. Sillanpää, Sep. Purif. Technol., 2019, 228, 1–13 Search PubMed.
- M. Adeel, M. Saeed, I. Khan, M. Muneer and N. Akram, ACS Omega, 2021, 6, 1426–1435 CrossRef CAS.
- Q. Li, Z. Chen, H. Wang, H. Yang, T. Wen, S. Wang, B. Hu and X. Wang, Sci. Total Environ., 2021, 792, 148546 CrossRef CAS PubMed.
- H. Ben Slama, A. C. Bouket, Z. Pourhassan, F. N. Alenezi, A. Silini, H. Cherif-Silini, T. Oszako, L. Luptakova, P. Golińska and L. Belbahri, Appl. Sci., 2021, 11, 1–21 Search PubMed.
- R. Al-Tohamy, S. S. Ali, F. Li, K. M. Okasha, Y. A. G. Mahmoud, T. Elsamahy, H. Jiao, Y. Fu and J. Sun, Ecotoxicol. Environ. Saf., 2022, 231, 113160 CrossRef CAS PubMed.
- M. T. Uddin, M. E. Hoque and M. Chandra Bhoumick, RSC Adv., 2020, 10, 23554–23565 RSC.
- M. Mehrjouei, S. Müller and D. Möller, Chem. Eng. J., 2015, 263, 209–219 CrossRef CAS.
- V. O. Ndabankulu, S. Maddila and S. B. Jonnalagadda, Can. J. Chem., 2019, 1–10 Search PubMed.
- M. Zhou, X. Tian, H. Yu, Z. Wang, C. Ren, L. Zhou, Y. W. Lin and L. Dou, ACS Omega, 2021, 6, 26439–26453 CrossRef CAS PubMed.
- M. Mishra and D. M. Chun, Appl. Catal., A, 2015, 498, 126–141 CrossRef CAS.
- M. M. Khan, S. F. Adil and A. Al-Mayouf, J. Saudi Chem. Soc., 2015, 19, 462–464 CrossRef.
- M. Muthukumaran, G. Gnanamoorthy, P. Varun Prasath, M. Abinaya, G. Dhinagaran, S. Sagadevan, F. Mohammad, W. C. Oh and K. Venkatachalam, Mater. Res. Express, 2020, 7, 015038 CrossRef CAS.
- Y. Boyjoo, H. Sun, J. Liu, V. K. Pareek and S. Wang, Chem. Eng. J., 2017, 310, 537–559 CrossRef CAS.
- S. Sood, A. Umar, S. K. Mehta and S. K. Kansal, J. Colloid Interface Sci., 2015, 450, 213–223 CrossRef CAS PubMed.
- D. Zhu and Q. Zhou, Environ. Nanotechnol., Monit. Manage., 2019, 12, 100255 Search PubMed.
- A. Mehtab, J. Ahmed, S. M. Alshehri, Y. Mao and T. Ahmad, Nanotechnology, 2022, 33, 142001 CrossRef.
- A. H. Zahid and Q. Han, Nanoscale, 2021, 13, 17687–17724 RSC.
- J. Luo, S. Zhang, M. Sun, L. Yang, S. Luo and J. C. Crittenden, ACS Nano, 2019, 13, 9811–9840 CrossRef CAS PubMed.
- T. Ma, C. Yang, L. Guo, R. A. Soomro, D. Wang, B. Xu and F. Fu, Appl. Catal., B, 2023, 330, 122643 CrossRef CAS.
- D. Ayodhya and G. Veerabhadram, Mater. Today Energy, 2018, 9, 83–113 CrossRef.
- S. Yadav, K. Shakya, A. Gupta, D. Singh, A. R. Chandran, A. Varayil Aanappalli, K. Goyal, N. Rani and K. Saini, Environ. Sci. Pollut. Res., 2022, 30, 71912–71932 CrossRef PubMed.
- A. B. Djurišić, Y. H. Leung and A. M. Ching Ng, Mater. Horiz., 2014, 1, 400–410 RSC.
- S. Sukumar, A. Rudrasenan and D. Padmanabhan Nambiar, ACS Omega, 2020, 5, 1040–1051 CrossRef CAS PubMed.
- M. S. S. Danish, L. L. Estrella, I. M. A. Alemaida, A. Lisin, N. Moiseev, M. Ahmadi, M. Nazari, M. Wali, H. Zaheb and T. Senjyu, Metals, 2021, 11, 80 CrossRef CAS.
- C. Siriwong, N. Wetchakun, B. Inceesungvorn, D. Channei, T. Samerjai and S. Phanichphant, Prog. Cryst. Growth Charact. Mater., 2012, 58, 145–163 CrossRef CAS.
- M. Nafees, M. Ikram and S. Ali, Appl. Nanosci., 2017, 7, 399–406 CrossRef CAS.
- A. V. Borhade, B. K. Uphade and D. R. Tope, J. Chem. Sci., 2013, 125, 583–589 CrossRef CAS.
- A. V Borhade, D. R. Tope and B. K. Uphade, E-J. Chem., 2012, 9, 705–715 CrossRef.
- W. Li, H. Yang and Q. Liu, J. Nanomater., 2017, 2017, 1–8 Search PubMed.
- V. Mirzajani, K. Farhadi and S. M. Pourmortazavi, J. Therm. Anal. Calorim., 2018, 131, 937–948 CrossRef CAS.
- M. S. Chavali and M. P. Nikolova, SN Appl. Sci., 2019, 1, 1–30 CrossRef.
- S. Wang, Z. Wang and Z. Zha, Dalton Trans., 2009, 9363 RSC.
- A. Aliakbari, E. Najafi, M. M. Amini and S. W. Ng, Monatsh. Chem., 2014, 145, 1277–1285 CrossRef CAS.
- İ. K. Akbay, A. Güngör, R. Genç and T. Özdemir, Selcuk Univ. J. Eng. Science Technol., 2019, 7, 298–308 Search PubMed.
- A. Güngör, R. Genç and T. Özdemir, J. Turk. Chem. Soc., Sect. A, 2017, 4, 1017–1030 CrossRef.
- R. Tayebee, B. Maleki and M. sabeti, J. Iran. Chem. Soc., 2017, 14, 1179–1188 CrossRef CAS.
- S. Yazdani Darki, M. Eslami-Kalantari, H. Zare and Z. Shahedi, Mater. Chem. Phys., 2021, 262, 124305 CrossRef CAS.
- Y. Wang and Y. Xia, Nano Lett., 2004, 4, 2047–2050 CrossRef CAS.
- N. Baig, I. Kammakakam, W. Falath and I. Kammakakam, Mater. Adv., 2021, 2, 1821–1871 RSC.
- V. Galstyan, M. P. Bhandari, V. Sberveglieri, G. Sberveglieri and E. Comini, Chemosensors, 2018, 6, 1–21 CrossRef.
- D. Bokov, A. Turki Jalil, S. Chupradit, W. Suksatan, M. Javed Ansari, I. H. Shewael, G. H. Valiev and E. Kianfar, Adv. Mater. Sci. Eng., 2021, 2021, 1–21 CrossRef.
- E. M. Modan and A. G. Plăiaşu, Ann. “Dunarea Jos” Univ. Galati. Fascicle IX, Metall. Mater. Sci., 2020, 43, 53–60 CAS.
- P. G. Jamkhande, N. W. Ghule, A. H. Bamer and M. G. Kalaskar, J. Drug Delivery Sci. Technol., 2019, 53, 101174 CrossRef CAS.
- A. Pohl, Water, Air, Soil Pollut., 2020, 231, 503 CrossRef CAS.
- D. A. Alromi, S. Y. Madani and A. Seifalian, Polymers, 2021, 13, 1–26 CrossRef.
- R. Medhi, M. D. Marquez and T. R. Lee, ACS Appl. Nano Mater., 2020, 3, 6156–6185 CrossRef CAS.
- H. Chakhtouna, H. Benzeid, N. Zari, A. el kacem Qaiss and R. Bouhfid, Environ. Sci. Pollut. Res., 2021, 28, 44638–44666 CrossRef CAS PubMed.
- M. Parashar, V. K. Shukla and R. Singh, J. Mater. Sci.: Mater. Electron., 2020, 31, 3729–3749 CrossRef CAS.
- R. Mahdavi and S. S. A. Talesh, Adv. Powder Technol., 2017, 28, 1418–1425 CrossRef CAS.
- M. V. Arularasu, SN Appl. Sci., 2019, 1, 393 CrossRef CAS.
- S. Liu, X. Wei, S. Lin and M. Guo, J. Sol-Gel Sci. Technol., 2021, 99, 122–131 CrossRef CAS.
- F. Heshmatpour and R. B. Aghakhanpour, Powder Technol., 2011, 205, 193–200 CrossRef CAS.
- H. Siddiqui, M. R. Parra, M. M. Malik and F. Z. Haque, Opt. Quantum Electron., 2018, 50, 260 CrossRef.
- L. F. de S. Lima, C. R. Coelho, G. H. M. Gomes and N. D. S. Mohallem, J. Sol-Gel Sci. Technol., 2020, 93, 168–174 CrossRef CAS.
- M. Patel, S. Mishra, R. Verma and D. Shikha, Discov. Mater., 2022, 2, 1 CrossRef.
- S. Ahmadi, N. Asim, M. A. Alghoul, F. Y. Hammadi, K. Saeedfar, N. A. Ludin, S. H. Zaidi and K. Sopian, Int. J. Photoenergy, 2014, 2014, 1–21 CrossRef.
- A. Serrà and E. Vallés, Catalysts, 2018, 8, 395 CrossRef.
- J. Abel, A. Pramothkumar, N. Senthilkumar, K. Jothivenkatachalam, P. Fermi Hilbert Inbaraj and J. Joseph prince, Phys. Rev. B, 2019, 572, 117–124 CrossRef.
- S. Jamil, S. R. Khan, B. Sultana, M. Hashmi, M. Haroon and M. R. S. A. Janjua, J. Cluster Sci., 2018, 29, 1099–1106 CrossRef CAS.
- M. G. Kotresh, M. K. Patil and S. R. Inamdar, Optik, 2021, 243, 167506 CrossRef CAS.
- P. Yadav, R. Saini and A. Bhaduri, Environ. Sci. Pollut. Res., 2022, 30, 71439–71453 CrossRef PubMed.
- A. Helal, S. M. El-Sheikh, J. Yu, A. I. Eid, S. A. El-Haka and S. E. Samra, J. Nanopart. Res., 2020, 22, 132 CrossRef CAS.
- M. Kandasamy, A. Seetharaman, D. Sivasubramanian, A. Nithya, K. Jothivenkatachalam, N. Maheswari, M. Gopalan, S. Dillibabu and A. Eftekhari, ACS Appl. Nano Mater., 2018, 1, 5823–5836 CrossRef CAS.
- D. Liang, S. Liu, Z. Wang, Y. Guo, W. Jiang, C. Liu, H. Wang, N. Wang, W. Ding, M. He, L. Wang and S. Xu, J. Mater. Sci.: Mater. Electron., 2019, 30, 12619–12629 CrossRef CAS.
- B. Uma, K. S. Anantharaju, L. Renuka, S. Malini, S. S. More, Y. S. Vidya and S. Meena, Ceram. Int., 2021, 47, 10355–10369 CrossRef CAS.
- B. A. Lemecho, F. K. Sabir, D. M. Andoshe, N. S. Gultom, D. H. Kuo, X. Chen, E. Mulugeta, T. D. Desissa and O. A. Zelekew, Bioinorg. Chem. Appl., 2022, 2022, 1–10 CrossRef PubMed.
- S. Pourmasoud, M. Eghbali-Arani, F. Ahmadi and M. Rahimi-Nasrabadi, J. Mater. Sci.: Mater. Electron., 2017, 28, 17089–17097 CrossRef CAS.
- W. Buraso, V. Lachom, P. Siriya and P. Laokul, Mater. Res. Express, 2018, 5, 1–10 Search PubMed.
- W. M. Rangel, R. A. A. Boca Santa and H. G. Riella, J. Mater. Res. Technol., 2020, 9, 994–1004 CrossRef CAS.
- Y. X. Gan, A. H. Jayatissa, Z. Yu, X. Chen and M. Li, J. Nanomater., 2020, 2020, 1–3 CrossRef.
- S. K. Godlaveeti, A. R. Somala, S. S. Sana, M. Ouladsmane, A. A. Ghfar and R. R. Nagireddy, J. Cluster Sci., 2022, 33, 1635–1644 CrossRef CAS.
- A. M. Shahat, F. M. El-Hossary, A. Ghitas, A. M. Abd El-Rahman and A. A. Ebnalwaled, IOP Conf. Ser.: Mater. Sci. Eng., 2021, 1171, 012008 Search PubMed.
- M. R. Abhilash, A. Gangadhar, J. Krishnegowda, M. Chikkamadaiah and S. Srikantaswamy, RSC Adv., 2019, 9, 25158–25169 RSC.
- M. Khashaei, L. Kafi-Ahmadi, S. Khademinia, A. Poursattar Marjani and E. Nozad, Sci. Rep., 2022, 12, 1–15 CrossRef PubMed.
- W. Wei, X. Yue, H. Cui, X. Lü and J. Xie, J. Mater. Res., 2013, 28, 3408–3416 CrossRef CAS.
- B. R. Bade, S. R. Rondiya, Y. V. Hase, M. P. Nasane, S. B. Jathar, S. V. Barma, K. B. Kore, D. S. Nilegave, S. R. Jadkar and A. M. Funde, AIP Conf. Proc., 2021, 2335, 100001 CrossRef CAS.
- S. Suthakaran, S. Dhanapandian, N. Krishnakumar and N. Ponpandian, Mater. Res. Express, 2019, 6, 0850i3 CrossRef CAS.
- S. S. Low, M. Yew, C. N. Lim, W. S. Chai, L. E. Low, S. Manickam, B. T. Tey and P. L. Show, Ultrason. Sonochem., 2022, 82, 105887 CrossRef CAS PubMed.
- B. Savun-Hekimoğlu, Acoustics, 2020, 2, 766–775 CrossRef.
- M. A. Dheyab, A. A. Aziz and M. S. Jameel, Molecules, 2021, 26, 2453 CrossRef CAS PubMed.
- S. Stankic, S. Suman, F. Haque and J. Vidic, J. Nanobiotechnol., 2016, 14, 1–20 CrossRef PubMed.
- K. Wongwailikhit and S. Horwongsakul, Mater. Lett., 2011, 65, 2820–2822 CrossRef CAS.
- Y. Shlapa, V. Sarnatskaya, I. Timashkov, L. Yushko, I. Antal, B. Gerashchenko, I. Nychyporenko, A. Belous, V. Nikolaev and M. Timko, Appl. Phys. A: Mater. Sci. Process., 2019, 125, 1–10 CrossRef CAS.
- Y. Du, W. Wang, X. Li, J. Zhao, J. Ma, Y. Liu and G. Lu, Mater. Lett., 2012, 68, 168–170 CrossRef CAS.
- Ö. A. Yildirim and C. Durucan, J. Alloys Compd., 2010, 506, 944–949 CrossRef.
- M. H. Zori, J. Inorg. Organomet. Polym. Mater., 2011, 21, 81–90 CrossRef.
- A. Kumar, A. Saxena, A. De, R. Shankar and S. Mozumdar, RSC Adv., 2013, 3, 5015–5021 RSC.
- J. Guo, S. Zhu, Z. Chen, Y. Li, Z. Yu, Q. Liu, J. Li, C. Feng and D. Zhang, Ultrason. Sonochem., 2011, 18, 1082–1090 CrossRef CAS PubMed.
- M. Mousavi-Kamazani and F. Azizi, Ultrason. Sonochem., 2019, 58, 104695 CrossRef CAS PubMed.
- M. J. Kishor Kumar and J. T. Kalathi, J. Alloys Compd., 2018, 748, 348–354 CrossRef CAS.
- M. T. Noman, M. Petru, J. Militky, M. Azeem and M. A. Ashraf, Materials, 2020, 13, 14 CrossRef CAS PubMed.
- V. Ramasamy Raja, D. R. Rosaline, A. Suganthi and M. Rajarajan, Ultrason. Sonochem., 2018, 44, 310–318 CrossRef CAS PubMed.
- A. Manivel, G. J. Lee, C. Y. Chen, J. H. Chen, S. H. Ma, T. L. Horng and J. J. Wu, Mater. Res. Bull., 2015, 62, 184–191 CrossRef CAS.
- G. Gyawali, R. Adhikari, B. Joshi, T. H. Kim, V. Rodríguez-González and S. W. Lee, J. Hazard. Mater., 2013, 263, 45–51 CrossRef CAS PubMed.
- R. Arunadevi, B. Kavitha, M. Rajarajan and A. Suganthi, Chem. Phys. Lett., 2019, 715, 252–262 CrossRef CAS.
- A. Hassanjani-Roshan, M. R. Vaezi, A. Shokuhfar and Z. Rajabali, Particuology, 2011, 9, 95–99 CrossRef CAS.
- C. N. C. Hitam and A. A. Jalil, J. Environ. Manage., 2020, 258, 110050 CrossRef CAS PubMed.
- W. S. Koe, J. W. Lee, W. C. Chong, Y. L. Pang and L. C. Sim, Environ. Sci. Pollut. Res., 2020, 27, 2522–2565 CrossRef CAS PubMed.
- P. O. Oladoye, T. O. Ajiboye, E. O. Omotola and O. J. Oyewola, Results Eng., 2022, 16, 100678 CrossRef CAS.
- A. Krishna Moorthy, B. Govindarajan Rathi, S. P. Shukla, K. Kumar and V. Shree Bharti, Environ. Toxicol. Pharmacol., 2021, 82, 103552 CrossRef CAS PubMed.
- S. Dardouri and J. Sghaier, Korean J. Chem. Eng., 2017, 34, 1037–1043 CrossRef CAS.
- S. A. Ong, E. Toorisaka, M. Hirata and T. Hano, J. Hazard. Mater., 2005, 124, 88–94 CrossRef CAS PubMed.
- M. Saeed, M. A. Jamal, A. U. Haq, M. Ilyas, M. Younas and M. A. Shahzad, Int. J. Chem. React. Eng., 2016, 14, 45–51 CrossRef CAS.
- I. Khan, K. Saeed, I. Zekker, B. Zhang, A. H. Hendi, A. Ahmad, S. Ahmad, N. Zada, H. Ahmad, L. A. Shah, T. Shah and I. Khan, Water, 2022, 14, 242 CrossRef CAS.
- M. Khodaie, N. Ghasemi, B. Moradi and M. Rahimi, J. Chem., 2013, 2103, 1–7 Search PubMed.
- R. R. Ramsay, C. Dunford and P. K. Gillman, Br. J. Pharmacol., 2007, 152, 946–951 CrossRef CAS PubMed.
- J. Fito, S. Abrham and K. Angassa, Int. J. Environ. Res., 2020, 14, 501–511 CrossRef CAS.
- A. M. McDonnell, I. Rybak, M. Wadleigh and D. C. Fisher, J. Oncol. Pharm. Pract., 2012, 18, 436–439 CrossRef CAS PubMed.
- P. K. Gillman, Anaesthesia, 2006, 61, 1007–1019 CrossRef PubMed.
- R. Prashant and S. Jyothi, J. Anaesthesiol., Clin. Pharmacol., 2010, 26, 517–520 CrossRef.
- S. Shakoor and A. Nasar, Groundw. Sustain. Dev., 2017, 5, 152–159 CrossRef.
- X. He and C. Zhang, J. Mater. Sci., 2019, 54, 8831–8851 CrossRef CAS.
- A. Ajmal, I. Majeed, R. N. Malik, H. Idriss and M. A. Nadeem, RSC Adv., 2014, 4, 37003–37026 RSC.
- T. Velempini, E. Prabakaran and K. Pillay, Mater. Today Chem., 2021, 19, 100380 CrossRef CAS.
- H. Tong, S. Ouyang, Y. Bi, N. Umezawa, M. Oshikiri and J. Ye, Adv. Mater., 2012, 24, 229–251 CrossRef CAS PubMed.
- J. Zhu and M. Zäch, Curr. Opin. Colloid Interface Sci., 2009, 14, 260–269 CrossRef CAS.
- M. Nazim, A. A. P. Khan, A. M. Asiri and J. H. Kim, ACS Omega, 2021, 6, 2601–2612 CrossRef CAS PubMed.
- P. Sangpour, F. Hashemi and A. Z. Moshfegh, J. Phys. Chem. C, 2010, 114, 13955–13961 CrossRef CAS.
- Q. Xiao, J. Zhang, C. Xiao, Z. Si and X. Tan, Sol. Energy, 2008, 82, 706–713 CrossRef CAS.
- K. V. Kumar, K. Porkodi and F. Rocha, Catal. Commun., 2008, 9, 82–84 CrossRef CAS.
- N. G. Asenjo, R. Santamaría, C. Blanco, M. Granda, P. Álvarez and R. Menéndez, Carbon, 2013, 55, 62–69 CrossRef CAS.
- H. Anwer, A. Mahmood, J. Lee, K. H. Kim, J. W. Park and A. C. K. Yip, Nano Res., 2019, 12, 955–972 CrossRef CAS.
- A. M. Mohammed, S. S. Mohtar, F. Aziz, S. A. Mhamad and M. Aziz, J. Environ. Chem. Eng., 2021, 9, 105138 CrossRef CAS.
- I. Ahmad, Y. Zou, J. Yan, Y. Liu, S. Shukrullah, M. Y. Naz, H. Hussain, W. Q. Khan and N. R. Khalid, Adv. Colloid Interface Sci., 2023, 311, 102830 CrossRef CAS PubMed.
- Y. Liu, W. Wang, X. Xu, J. P. Marcel Veder and Z. Shao, J. Mater. Chem. A, 2019, 7, 7280–7300 RSC.
- X. Lin, D. Fu, L. Hao and Z. Ding, J. Environ. Sci., 2013, 25, 2150–2156 CrossRef CAS PubMed.
- Z. Ji, Z. Luo, J. Li and P. Li, Phys. Status Solidi A, 2019, 216, 1–9 CrossRef.
- T. Leijtens, G. E. Eperon, A. J. Barker, G. Grancini, W. Zhang, J. M. Ball, A. R. S. Kandada, H. J. Snaith and A. Petrozza, Energy Environ. Sci., 2016, 9, 3472–3481 RSC.
- B. Peng, X. Meng, F. Tang, X. Ren, D. Chen and J. Ren, J. Phys. Chem. C, 2009, 113, 20240–20245 CrossRef CAS.
- K. Pradeev raj, K. Sadaiyandi, A. Kennedy, S. Sagadevan, Z. Z. Chowdhury, M. R. Bin Johan, F. A. Aziz, R. F. Rafique, R. Thamiz Selvi and R. Rathina bala, Nanoscale Res. Lett., 2018, 13, 229 CrossRef CAS PubMed.
- N. Sakaguchi Miyamoto, R. Miyamoto, E. Giamello, T. Kurisaki and H. Wakita, Res. Chem. Intermed., 2018, 44, 4577–4594 CrossRef CAS.
- T. Narkbuakaew and P. Sujaridworakun, Opt. Mater., 2019, 98, 109407 CrossRef CAS.
- J. I. Garza-Arévalo, I. García-Montes, M. H. Reyes, J. L. Guzmán-Mar, V. Rodríguez-González and L. H. Reyes, Mater. Res. Bull., 2016, 73, 145–152 CrossRef.
- W. Xie, R. Li and Q. Xu, Sci. Rep., 2018, 8, 1–10 CAS.
- P. Karuppasamy, N. Ramzan Nilofar Nisha, A. Pugazhendhi, S. Kandasamy and S. Pitchaimuthu, J. Environ. Chem. Eng., 2021, 9, 105254 CrossRef CAS.
- A. Khlyustova, N. Sirotkin, T. Kusova, A. Kraev, V. Titov and A. Agafonov, Mater. Adv., 2020, 1, 1193–1201 RSC.
- S. Saroj, L. Singh and S. V. Singh, J. Photochem. Photobiol., A, 2020, 396, 112532 CrossRef CAS.
- S. Ramandi, M. H. Entezari and N. Ghows, Ultrason. Sonochem., 2017, 38, 234–245 CrossRef CAS PubMed.
- N. U. Saqib, R. Adnan and I. Shah, Mater. Res. Express, 2019, 6, 095506 CrossRef CAS.
- L. N. Song, F. Ding, Y. K. Yang, D. Ding, L. Chen, C. T. Au and S. F. Yin, ACS Sustainable Chem. Eng., 2018, 6, 17044–17050 CrossRef CAS.
- A. M. Abdel-Wahab, A. S. Al-Shirbini, O. Mohamed and O. Nasr, J. Photochem. Photobiol., A, 2017, 347, 186–198 CrossRef CAS.
- G. Dong, Y. Wang, H. Lei, G. Tian, S. Qi and D. Wu, J. Cleaner Prod., 2020, 253, 120021 CrossRef CAS.
- M. Mohammadi and S. Sabbaghi, Environ. Nanotechnol., Monit. Manage., 2014, 1–2, 24–29 Search PubMed.
- J. Abdi, M. Yahyanezhad, S. Sakhaie, M. Vossoughi and I. Alemzadeh, J. Environ. Chem. Eng., 2019, 7, 103096 CrossRef CAS.
- Y. Zhang, X. Hou, T. Sun and X. Zhao, Ceram. Int., 2017, 43, 1150–1159 CrossRef CAS.
- D. Monga and S. Basu, Adv. Powder Technol., 2019, 30, 1089–1098 CrossRef CAS.
- W. K. Jo and T. S. Natarajan, Chem. Eng. J., 2015, 281, 549–565 CrossRef CAS.
- E. Vasilaki, I. Georgaki, D. Vernardou, M. Vamvakaki and N. Katsarakis, Appl. Surf. Sci., 2015, 353, 865–872 CrossRef CAS.
- M. Kocijan, L. Curkovi, T. Radoševi and M. Podlogar, Catal. Artic., 2021, 11, 1–16 Search PubMed.
- K. P. Ghoderao, S. N. Jamble and R. B. Kale, Optik, 2018, 156, 758–771 CrossRef CAS.
- M. C. Uribe-López, M. C. Hidalgo-López, R. López-González, D. M. Frías-Márquez, G. Núñez-Nogueira, D. Hernández-Castillo and M. A. Alvarez-Lemus, J. Photochem. Photobiol., A, 2021, 404, 112866 CrossRef.
- M. M. Ba-Abbad, A. A. H. Kadhum, A. B. Mohamad, M. S. Takriff and K. Sopian, Chemosphere, 2013, 91, 1604–1611 CrossRef CAS PubMed.
- U. Alam, A. Khan, D. Ali, D. Bahnemann and M. Muneer, RSC Adv., 2018, 8, 17582–17594 RSC.
- K. Qi, X. Xing, A. Zada, M. Li, Q. Wang, S. yuan Liu, H. Lin and G. Wang, Ceram. Int., 2020, 46, 1494–1502 CrossRef CAS.
- Z. Mirzaeifard, Z. Shariatinia, M. Jourshabani and S. M. Rezaei Darvishi, Ind. Eng. Chem. Res., 2020, 59, 15894–15911 CrossRef CAS.
- M. J. Mochane, M. T. Motloung, T. C. Mokhena and T. G. Mofokeng, Catalysts, 2022, 12, 1439 CrossRef CAS.
- E. Mugunthan, M. B. Saidutta and P. E. Jagadeeshbabu, J. Photochem. Photobiol., A, 2019, 383, 111993 CrossRef CAS.
- J. Lin, Z. Luo, J. Liu and P. Li, Mater. Sci. Semicond. Process., 2018, 87, 24–31 CrossRef CAS.
- S. Begum, S. R. Mishra and M. Ahmaruzzaman, Environ. Sci. Pollut. Res., 2022, 29, 87347–87360 CrossRef CAS PubMed.
- P. Cheng, Y. Wang, L. Xu, P. Sun, Z. Su, F. Jin, F. Liu, Y. Sun and G. Lu, Mater. Lett., 2016, 175, 52–55 CrossRef CAS.
- S. P. Lonkar, V. Pillai and A. Abdala, Appl. Surf. Sci., 2019, 465, 1107–1113 CrossRef CAS.
- W. M. Liu, J. Li and H. Y. Zhang, Optik, 2020, 207, 163778 CrossRef CAS.
- P. Van Tuan, T. T. Phuong, V. T. Tan, S. X. Nguyen and T. N. Khiem, Mater. Sci. Semicond. Process., 2020, 115, 105114 CrossRef CAS.
- M. Ahmad, I. Ahmad, E. Ahmed, M. S. Akhtar and N. R. Khalid, J. Mol. Liq., 2020, 311, 113326 CrossRef CAS.
- I. Ahmad, Sep. Purif. Technol., 2020, 251, 117372 CrossRef CAS.
- O. Długosz and M. Banach, Appl. Nanosci., 2021, 11, 1707–1719 CrossRef.
- M. A. Butler, R. D. Nasby and R. K. Quinn, Solid State Commun., 1976, 19, 1011–1014 CrossRef CAS.
- R. Rong and L. Wang, J. Alloys Compd., 2021, 850, 156742 CrossRef CAS.
- S. Ramkumar and G. Rajarajan, Appl. Phys. A: Mater. Sci. Process., 2017, 123, 1–8 CrossRef CAS.
- C. Wang, X. Zhang, B. Yuan, Y. Wang, P. Sun, D. Wang, Y. Wei and Y. Liu, Chem. Eng. J., 2014, 237, 29–37 CrossRef CAS.
- G. Jeevitha, R. Abhinayaa, D. Mangalaraj and N. Ponpandian, J. Phys. Chem. Solids, 2018, 116, 137–147 CrossRef CAS.
- B. Esencan Türkaslan, A. K. Çelik, A. Dalbeyler and N. Fantuzzi, Materials, 2022, 15, 8019 CrossRef PubMed.
- V. Ramar and K. Balasubramanian, ACS Appl. Nano Mater., 2021, 4, 5512–5521 CrossRef CAS.
- T. Govindaraj, C. Mahendran, V. S. Manikandan, J. Archana, M. Shkir and J. Chandrasekaran, J. Alloys Compd., 2021, 868, 159091 CrossRef CAS.
- V. A. Tran, T. P. Nguyen, V. T. Le, I. T. Kim, S. W. Lee and C. T. Nguyen, J. Sci.: Adv. Mater. Devices, 2021, 6, 108–117 CAS.
- Y. Yang, B. Liu, J. Xu, Q. Wang, X. Wang, G. Lv and J. Zhou, ACS Omega, 2022, 7, 6035–6045 CrossRef CAS PubMed.
- M. Y. Khan, M. Ahmad, S. Sadaf, S. Iqbal, F. Nawaz and J. Iqbal, J. Mater. Res. Technol., 2019, 8, 3261–3269 CrossRef CAS.
- A. Kerour, S. Boudjadar, R. Bourzami and B. Allouche, J. Solid State Chem., 2018, 263, 79–83 CrossRef CAS.
- S. Yadav, M. Chauhan, D. Mathur, A. Jain and P. Malhotra, Environ. Dev. Sustain., 2021, 23, 2071–2091 CrossRef.
- D. A. Prado-Chay, M. A. Cortés-Jácome, C. Angeles-Chávez, R. Oviedo-Roa, J. M. Martínez-Magadán, C. Zuriaga-Monroy, I. J. Hernández-Hernández, P. R. Mayoral, D. R. Gómora-Herrera and J. A. Toledo-Antonio, Top. Catal., 2020, 63, 586–600 CrossRef CAS.
- Y. Wang, J. Gao, X. Wang, L. Jin, L. Fang, M. Zhang, G. He and Z. Sun, J. Sol-Gel Sci. Technol., 2018, 88, 172–180 CrossRef CAS.
- K. Polat, Thin Solid Films, 2020, 709, 138254 CrossRef CAS.
- S. Karthikeyan, K. Ahmed, A. Osatiashtiani, A. F. Lee, K. Wilson, K. Sasaki, B. Coulson, W. Swansborough-Aston, R. E. Douthwaite and W. Li, ChemCatChem, 2020, 12, 1699–1709 CrossRef CAS.
- M. V. Kangralkar, V. A. Kangralkar, N. Momin and J. Manjanna, Environ. Nanotechnol., Monit. Manage., 2019, 12, 100265 Search PubMed.
- S. Masudy-Panah, R. Katal, N. D. Khiavi, E. Shekarian, J. Hu and X. Gong, J. Mater. Chem. A, 2019, 7, 22332–22345 RSC.
- H. Gao, J. Zhang and M. Wang, Mater. Res. Bull., 2013, 48, 3431–3437 CrossRef CAS.
- K. Chen, X. Wang, P. Xia, J. Xie, J. Wang, X. Li, Y. Tang and L. Li, Chemosphere, 2020, 254, 126806 CrossRef CAS PubMed.
- R. Banu, N. Salvi, S. Gupta, C. Ameta, R. Ameta and P. B. Punjabi, Arabian J. Sci. Eng., 2022, 47, 365–378 CrossRef CAS.
- Z. Zhang, L. Sun, Z. Wu, Y. Liu and S. Li, New J. Chem., 2020, 44, 6420–6427 RSC.
- A. A. M. Sakib, S. M. Masum, J. Hoinkis, R. Islam and M. A. I. Molla, J. Compos. Sci., 2019, 3, 91 CrossRef.
- D. Malwal and P. Gopinath, Catal. Sci. Technol., 2016, 6, 4458–4472 RSC.
- A. George, D. Magimai Antoni Raj, X. Venci, A. Dhayal Raj, A. Albert Irudayaraj, R. L. Josephine, S. John Sundaram, A. M. Al-Mohaimeed, D. A. Al Farraj, T. W. Chen and K. Kaviyarasu, Environ. Res., 2022, 203, 111880 CrossRef CAS PubMed.
- M. Maraj, A. Raza, X. Wang, J. Chen, K. N. Riaz and W. Sun, Catalysts, 2021, 11, 1–10 CrossRef.
- M. R. Islam, M. Saiduzzaman, S. S. Nishat, A. Kabir and S. F. U. Farhad, Colloids Surf., A, 2021, 617, 126386 CrossRef CAS.
- S. Aroob, S. A. C. Carabineiro, M. B. Taj, I. Bibi, A. Raheel, T. Javed, R. Yahya, W. Alelwani, F. Verpoort, K. Kamwilaisak, S. Al-Farraj and M. Sillanpää, Catalysts, 2023, 13, 502 CrossRef CAS.
- M. Rafique, F. Shafiq, S. S. Ali Gillani, M. Shakil, M. B. Tahir and I. Sadaf, Optik, 2020, 208, 164053 CrossRef CAS.
- K. Kannan, D. Radhika, S. Vijayalakshmi, K. K. Sadasivuni, A. A. Ojiaku and U. Verma, Int. J. Environ. Anal. Chem., 2022, 102, 1095–1108 CrossRef CAS.
- Y. Duan, Mater. Res. Bull., 2018, 105, 68–74 CrossRef CAS.
- M. A. Hanif, J. Akter, Y. S. Kim, H. G. Kim, J. R. Hahn and L. K. Kwac, Catalysts, 2022, 12, 151 CrossRef CAS.
- S. P. Kim, M. Y. Choi and H. C. Choi, Mater. Res. Bull., 2016, 74, 85–89 CrossRef CAS.
- Y. Li, Q. Yang, Z. Wang, G. Wang, B. Zhang, Q. Zhang and D. Yang, Inorg. Chem. Front., 2018, 5, 3005–3014 RSC.
- L. G. Devi and R. Shyamala, Mater. Chem. Front., 2018, 2, 796–806 RSC.
- S. Yao, S. Zhou, J. Wang, W. Li and Z. Li, Photochem. Photobiol. Sci., 2019, 18, 2989–2999 CrossRef CAS PubMed.
- C. Wang, G. Guo, C. Zhu, Y. Li, Y. Jin, B. Zou, H. He and A. Wang, Nanomaterials, 2022, 12, 3151 CrossRef CAS PubMed.
- S. A. Ansari, M. M. Khan, M. O. Ansari, J. Lee and M. H. Cho, RSC Adv., 2014, 4, 26013–26021 RSC.
- L. Ran, D. Zhao, X. Gao and L. Yin, CrystEngComm, 2015, 17, 4225–4237 RSC.
- A. B. Ali Baig, V. Rathinam and V. Ramya, Adv. Compos. Hybrid Mater., 2021, 4, 114–126 CrossRef CAS.
- Q. Wang, X. Chen, J. Tian, L. Wei, Y. Liu and C. Yang, J. Mater. Sci.: Mater. Electron., 2020, 31, 16991–17002 CrossRef CAS.
- H. Ji, Y. Fan, J. Yan, Y. Xu, X. She, J. Gu, T. Fei, H. Xu and H. Li, RSC Adv., 2017, 7, 36101–36111 RSC.
- S. Kavitha, N. Jayamani and D. Barathi, Bull. Mater. Sci., 2021, 44, 26 CrossRef CAS.
- M. Kumar and A. Rahman, J. Inst. Eng.: Ser. E, 2023, 104, 95–108 CAS.
- L. Machala, J. Tuček and R. Zbořil, Chem. Mater., 2011, 23, 3255–3272 CrossRef CAS.
- Y. Wang, S. Wang, Y. Wu, Z. Wang, H. Zhang, Z. Cao, J. He, W. Li, Z. Yang, L. Zheng, D. Feng, P. Pan, J. Bi, H. Li, J. Zhao and K. Zhang, J. Alloys Compd., 2021, 851, 156733 CrossRef CAS.
- M. A. Hoque, M. R. Ahmed, G. T. Rahman, M. T. Rahman, M. A. Islam, M. A. Khan and M. K. Hossain, Results Phys., 2018, 10, 434–443 CrossRef.
- N. Li, Y. Jiang, Y. long He, L. Gao, Z. zhou Yi, F. rui Zhai and K. Chattopadhyay, J. Mater. Res. Technol., 2021, 15, 810–820 CrossRef CAS.
- R. A. Senthil, A. Priya, J. Theerthagiri, A. Selvi, P. Nithyadharseni and J. Madhavan, Ionics, 2018, 24, 3673–3684 CrossRef CAS.
- T. Zhang, J. Liu, F. Zhou, S. Zhou, J. Wu, D. Chen, Q. Xu and J. Lu, ACS Appl. Nano Mater., 2020, 3, 9200–9208 CrossRef CAS.
- S. Y. Mendiola-Alvarez, A. Hernández-Ramírez, J. L. Guzmán-Mar, M. L. Maya-Treviño, A. Caballero-Quintero and L. Hinojosa-Reyes, Catal. Today, 2019, 328, 91–98 CrossRef CAS.
- S. Frindy and M. Sillanpää, Mater. Des., 2020, 188, 108461 CrossRef CAS.
- X. Liu, K. Chen, J. J. Shim and J. Huang, J. Saudi Chem. Soc., 2015, 19, 479–484 CrossRef.
- H. Mansour, K. Omri, R. Bargougui and S. Ammar, Appl. Phys. A: Mater. Sci. Process., 2020, 126, 1–10 CrossRef.
- Q. Mei, F. Zhang, N. Wang, Y. Yang, R. Wu and W. Wang, RSC Adv., 2019, 9, 22764–22771 RSC.
- T. J. Al-Musawi, R. Asgariyan, M. Yilmaz, N. Mengelizadeh, A. Asghari, D. Balarak and M. Darvishmotevall, Magnetochemistry, 2022, 8, 137 CrossRef CAS.
- B. Mandal, J. Panda and B. Tudu, AIP Conf. Proc., 2018, 1953, 030173 CrossRef CAS.
- Y. Q. Cao, T. Q. Zi, X. R. Zhao, C. Liu, Q. Ren, J. Bin Fang, W. M. Li and A. D. Li, Sci. Rep., 2020, 10, 1–10 CrossRef CAS PubMed.
- Y. Zhu, J. Xue, T. Xu, G. He and H. Chen, J. Mater. Sci.: Mater. Electron., 2017, 28, 8519–8528 CrossRef CAS.
- K. K. Bera, M. Chakraborty, M. Mondal, S. Banik and S. K. Bhattacharya, Ceram. Int., 2020, 46, 7667–7680 CrossRef CAS.
- T. Li, S. Quan, X. Shi, L. Yang and C. Liu, Catal. Lett., 2020, 150, 640–651 CrossRef CAS.
- S. Singh and R. Sharma, Sol. Energy Mater. Sol. Cells, 2018, 186, 208–216 CrossRef CAS.
- Y. Long, L. Li, S. Wang, Y. Chen, L. Wang, S. Zhang, L. Luo and F. Jiang, Catal. Lett., 2018, 148, 3608–3617 CrossRef CAS.
- S. Zhang, S. Cao, Q. Shi, Y. Zhang, D. Sun, T. Tang and X. Zhang, Inorg. Chem., 2020, 59, 18131–18140 CrossRef PubMed.
- A. Hezam, K. Namratha, D. Ponnamma, Q. A. Drmosh, A. M. N. Saeed, C. Cheng and K. Byrappa, ACS Omega, 2018, 3, 12260–12269 CrossRef CAS PubMed.
- S. H. Hsieh, A. Manivel, G. J. Lee and J. J. Wu, Mater. Res. Bull., 2013, 48, 4174–4180 CrossRef CAS.
- Y. Cui, X. Zhang, R. Guo, H. Zhang, B. Li, M. Xie, Q. Cheng and X. Cheng, Sep. Purif. Technol., 2018, 203, 301–309 CrossRef CAS.
- D. S. Bhachu, S. Sathasivam, C. J. Carmalt and I. P. Parkin, Langmuir, 2014, 30, 624–630 CrossRef CAS PubMed.
- A. Leelavathi, B. Mukherjee, C. Nethravathi, S. Kundu, M. Dhivya, N. Ravishankar and G. Madras, RSC Adv., 2013, 3, 20970–20977 RSC.
- A. M. Mebed, A. M. Abd-Elnaiem, A. H. Alshammari, T. A. Taha, M. Rashad and D. Hamad, Catalysts, 2022, 12, 142 CrossRef CAS.
- Z. Wang, V. Srivastava, S. Iftekhar, I. Ambat and M. Sillanpää, Chem. Eng. J., 2018, 354, 663–671 CrossRef CAS.
- F. Y. Liu, J. H. Lin, Y. M. Dai, L. W. Chen, S. T. Huang, T. W. Yeh, J. L. Chang and C. C. Chen, Catal. Today, 2018, 314, 28–41 CrossRef CAS.
- F. T. Geldasa, M. Kebede, M. W. Shura, D. M. Andoshe, N. Tegegne and F. Gashaw Hone, Phys. Scr., 2023, 98, 065701 CrossRef.
- Y. Wang, C. Yang, K. Zhang, L. Guo, R. Li, A. Zaheer, F. Fu, B. Xu and D. Wang, Colloids Surf., A, 2022, 634, 127965 CrossRef CAS.
- F. T. Geldasa, M. A. Kebede, M. W. Shura and F. G. Hone, AIP Adv., 2022, 12, 115302 CrossRef CAS.
- C. G. Poll and D. J. Payne, Electrochim. Acta, 2014, 156, 283–288 CrossRef.
- J. Li, M. Guo, Y. Shao, H. Yu and K. Ni, ACS Omega, 2021, 6, 5436–5444 CrossRef CAS PubMed.
- E. Najafi, M. M. Amini, F. Behmagham, N. Shaabani and N. Shojaei, Chem. Rev. Lett., 2019, 2, 13–20 Search PubMed.
- H. Karami, M. A. Karimi, S. Haghdar, A. Sadeghi, R. Mir-Ghasemi and S. Mahdi-Khani, Mater. Chem. Phys., 2008, 108, 337–344 CrossRef CAS.
- K. T. Arulmozhi and N. Mythili, AIP Adv., 2013, 3, 122122 CrossRef.
- S. K. Noukelag, H. E. A. Mohamed, L. C. Razanamahandry, S. K. O. Ntwampe and C. J. Arendse, Mater. Today: Proc., 2019, 36, 421–426 Search PubMed.
- S. K. Behzad, E. Najafi, M. M. Amini, A. Tadjarodi, A. Dehghani and B. Notash, Monatsh. Chem., 2015, 146, 35–45 CrossRef CAS.
- K. H. H. Al-Attiyah, A. Hashim and S. F. Obaid, Int. J. Plast. Technol., 2019, 23, 39–45 CrossRef CAS.
- F. Temerov, Y. Baghdadi, E. Rattner and S. Eslava, ACS Appl. Energy Mater., 2022, 5, 14605–14637 CrossRef CAS PubMed.
- Y. C. Wang, A. H. Lee and C. C. Chen, J. Taiwan Inst. Chem. Eng., 2018, 93, 315–328 CrossRef CAS.
- Y. Li, J. Zhao, G. Zhang, L. Zhang, S. Ding, E. Shang and X. Xia, Water Res., 2019, 161, 251–261 CrossRef CAS PubMed.
- W. Kohn, A. D. Becke and R. G. Parr, J. Phys. Chem., 1996, 100, 12974–12980 CrossRef CAS.
- X. Andrade, C. Das Pemmaraju, A. Kartsev, J. Xiao, A. Lindenberg, S. Rajpurohit, L. Z. Tan, T. Ogitsu and A. A. Correa, J. Chem. Theory Comput., 2021, 17, 7447–7467 CrossRef CAS PubMed.
- A. Iwaszuk and M. Nolan, Catal. Sci. Technol., 2013, 3, 2000–2008 RSC.
- N. Kitchamsetti, M. S. Ramteke, S. R. Rondiya, S. R. Mulani, M. S. Patil, R. W. Cross, N. Y. Dzade and R. S. Devan, J. Alloys Compd., 2021, 855, 157337 CrossRef CAS.
- B. Huang and J. N. Hart, Phys. Chem. Chem. Phys., 2020, 22, 1727–1737 RSC.
- W. Yao, D. Li, S. Wei, X. Liu, X. Liu and W. Wang, ACS Omega, 2022, 7, 36479–36488 CrossRef CAS PubMed.
- N. I. Oktavianti, G. R. Anindika, A. L. Ivansyah, H. Juwono and Y. Kusumawati, AIP Conf. Proc., 2021, 2349, 020063 CrossRef CAS.
- R. Gusain, N. Kumar and S. S. Ray, in Photocatalysts in Advanced Oxidation Processes for Wastewater Treatment, 2020, pp. 229–270 Search PubMed.
- S. G. Shelar, V. K. Mahajan, S. P. Patil and G. H. Sonawane, SN Appl. Sci., 2020, 2, 1–10 CrossRef.
- A. Mohagheghian, S. A. Karimi, J. K. Yang and M. Shirzad-Siboni, J. Adv. Oxid. Technol., 2015, 18, 61–68 CAS.
- A. Rafiq, M. Ikram, S. Ali, F. Niaz, M. Khan, Q. Khan and M. Maqbool, J. Ind. Eng. Chem., 2021, 97, 111–128 CrossRef CAS.
- M. Senthil Kumar and C. Arunagiri, J. Mater. Sci.: Mater. Electron., 2021, 32, 17925–17935 CrossRef CAS.
- U. G. Akpan and B. H. Hameed, J. Hazard. Mater., 2009, 170, 520–529 CrossRef CAS PubMed.
- M. Saquib, M. A. Tariq, M. Faisal and M. Muneer, Adv. Sci. Lett., 2010, 3, 512–517 CrossRef.
- K. M. Reza, A. Kurny and F. Gulshan, Appl. Water Sci., 2017, 7, 1569–1578 CrossRef CAS.
- B. Neppolian, H. C. Choi, S. Sakthivel, B. Arabindoo and V. Murugesan, J. Hazard. Mater., 2002, 89, 303–317 CrossRef CAS PubMed.
- N. A. Galedari, M. Rahmani and M. Tasbihi, Environ. Sci. Pollut. Res., 2017, 24, 12655–12663 CrossRef CAS PubMed.
|
This journal is © The Royal Society of Chemistry 2023 |