DOI:
10.1039/D3RA00418J
(Paper)
RSC Adv., 2023,
13, 8564-8576
Interfacial construction of P25/Bi2WO6 composites for selective CO2 photoreduction to CO in gas–solid reactions†
Received
19th January 2023
, Accepted 23rd February 2023
First published on 14th March 2023
Abstract
Photocatalysis provides an attractive approach to convert CO2 into valuable fuels, which relies on a well-designed photocatalyst with good selectivity and high CO2 reduction ability. Herein, a series of P25/Bi2WO6 nanocomposites were synthesized by a simple one-step in situ hydrothermal method. The formation of a heterojunction between Bi2WO6, which absorbs visible light, and P25, which absorbs ultraviolet light, expands the utilization of sunlight by the catalysts, and consequently, leads to a remarkably enhanced CO2 selective photoreduction to CO. The maximum CO yield of the P25/Bi2WO6 heterojunction under simulated solar irradiation was 15.815 μmol g−1 h−1, which was 4.04 and 2.80 times higher than that of pure P25 and Bi2WO6, respectively. Our investigations verified a Z-scheme charge migration mechanism based on various characterization techniques between P25 and Bi2WO6. Furthermore, in situ DRIFTS uncovered the related reaction intermediates and CO2 photoreduction mechanism. Our work sheds light on investigating the efficacious construction of Bi2WO6-based hybrids for light-driven photocatalysis.
1 Introduction
The continuous consumption of global fossil energy and the increasing concentration of the greenhouse gas carbon dioxide (CO2) has led to a severe energy crisis and environmental pollution problems, which are not in line with the requirements of sustainable human development.1–3 Therefore, how to convert CO2 into chemical fuels has become a pressing problem.4,5 So far, CO2 reduction has usually been achieved by hydrogenation reactions at high temperature and pressure or in the presence of precious metal co-catalysts.6,7 On the other hand, inspired by plant photosynthesis, using solar energy to convert CO2 into useable chemicals is a low-cost and environmentally friendly strategy.8–10 Various photocatalysts, such as titanium dioxide (TiO2), cuprous oxide (Cu2O), carbon nitride (g-C3N4), strontium titanate (SrTiO3), bismuth chloride (BiOCl), and tungsten oxide (WO3), have been extensively studied and applied in this field of photocatalytic CO2 reduction.11–16 In general, the process of photocatalytic CO2 consists of the following steps. First, electron–hole pairs are excited by photons, where the photon energy is equal to or greater than the semiconductor bandgap. Second, light-induced carriers are separated and transported to the surface of the photocatalyst. Third, light-induced electrons react with CO2 at the surface-active center of the photocatalyst.17 However, the efficiency of most single-component photocatalysts for photocatalytic CO2 reduction is still far from practical due to the rapid compounding of electron–hole pairs and the lack of surface-active centers. In order to improve photocatalytic efficiency, one of the most effective ways is to construct heterojunction by compounding two semiconductors.18,19 This method can improve the separation and transfer of electron–hole pairs and provide more surface-active centers for photocatalytic reactions. Therefore, the construction of suitable heterojunction is essential to improve the CO2 reduction performance of photocatalysts.
TiO2 has the advantages of strong redox performance, non-toxic, low price, and high stability, and it has a broad development prospect in the field of photocatalytic CO2 reduction.20–22 Commercial TiO2 (P25) is a mixture of anatase and rutile TiO2 with forbidden bandwidths of 3.2 and 3.0 eV, respectively, and a wide band gap, which has solid photocatalytic properties under UV-vis light, and its particle size ranges from 20 to 40 nm, which has a large specific surface area.23 However, only 4% of sunlight is in the UV, and the lower light utilization and rapid compounding of electron–hole pairs limit the practical application of TiO2 photocatalytic CO2 reduction.24,25 Therefore, several methods have been used to improve the photocatalytic performance of TiO2, including doping, noble metal deposition, surface modification, and construction of heterojunction.26–29 As a representative method, combining UV-responsive semiconductor TiO2 and visible-light-responsive semiconductor to construct heterojunction facilitates carrier migration and separation. It improves the light adsorption capacity, improving its photocatalytic CO2 reduction performance.
Bismuth tungstate (Bi2WO6) is a visible light-responsive bismuth-based photocatalyst with a forbidden bandwidth of about 2.8 eV, one of the Aurivillius phase oxides, it is a complex, layered material consisting of alternating stacks of [Bi2O2]2+ layers and [WO4]2− layers.30 This layered structure facilitates the separation of electron–hole pairs and confers them to a high photocatalytic activity.31 However, Bi2WO6 exhibited poor light absorption performance and low photoconversion efficiency at wavelengths less than 450 nm.32 Combining Bi2WO6 and other semiconductor materials to construct heterojunction should be an effective strategy to solve this problem. So far, heterojunction structures have attracted significant attention in photocatalysis, and many heterojunction photocatalysts have been developed, such as Bi2WO6/MoO3, BiSn2O7/Bi2WO6, black phosphorus/Bi2WO6, and g-C3N4/Bi2WO6, etc.33–36 Combining P25 nanoparticles with Bi2WO6 can improve the photocatalytic performance. The synergistic effect between them can improve the light adsorption ability and facilitate the separation of electron–hole pairs. More importantly, the agglomeration phenomenon of P25 nanoparticles can be effectively improved. To the best of our knowledge, the application of P25/Bi2WO6 nanocomposites in photocatalytic CO2 reduction has been little studied.
In this work, we have successfully prepared P25/Bi2WO6 nanocomposites using a simple one-step in situ hydrothermal method. It was used for the photocatalytic reduction of CO2 in the absence of sacrificial agents, and the physical and photochemical properties of the material were characterized by various characterization tools. The reaction process and mechanism of the photocatalytic system were further investigated by in situ infrared testing. Compared with pure P25 and Bi2WO6, there is a strong synergy between the nanocomposites, which not only broadens the light absorption range but also suppresses the electron–hole pair recombination and has a high selectivity for carbon monoxide (CO). This study can provide new ideas for Bi2WO6-based nanocomposites in photocatalysis.
2 Experimental section
2.1. Materials
Sodium tungstate dihydrate (Na2WO4·2H2O, 99.5%) and titanium dioxide (P25) were purchased from Shanghai Macklin Biochemical Co., Ltd, China. Bismuth(III) nitrate pentahydrate (Bi(NO3)3·5H2O, 99.0%) was purchased from Sinopharm Chemical Reagent Co., Ltd, China. Cetyltrimethylammonium bromide (CTAB, 99.0%) was purchased from Damao Chemical Reagent Factory Co., Ltd, China. Sodium hydrogen carbonate (NaHCO3, 99.5%) was purchased from Guangdong Guanghua Sci-Tech Co., Ltd, China. All chemicals were used as received without further purification. The water used throughout the research is deionized water.
2.2. Synthesis
2.2.1 Preparation of the P25/Bi2WO6 photocatalyst. P25/Bi2WO6 photocatalyst was prepared according to the previous reports with some modifications.37 In a typical procedure (Scheme 1), 2 mmol Bi(NO3)3·5H2O and a known mass of P25 were mixed in 40 mL deionized water, which was labeled as Solution A. 1 mmol Na2WO4·2H2O and 0.05 g CTAB were added to 40 mL of deionized water with ultrasonic for 10 min and stirring for 30 min at ambient temperature, which was labeled as Solution B. Then Solution A was slowly added into Solution B and stirring for 30 min at ambient temperature. The resulting suspension was transferred into a 100 mL Teflon-lined autoclave and heated at 120 °C for 24 h. The final products were collected by centrifugation, washed several times with ethanol and deionized water, and dried at 60 °C in vacuum for 12 h. The mass ratios of P25 to Bi2WO6 were set as 10%, 20%, 30%, and 40%, and they were labeled as P25/BWO-10, P25/BWO-20, P25/BWO-30, and P25/BWO-40, respectively.
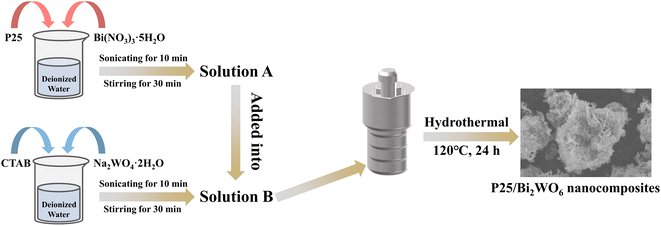 |
| Scheme 1 Schematic illustration of the preparation process of the P25/Bi2WO6 composite. | |
2.2.2 Preparation of Bi2WO6 nanosheets. For comparison, under the same experimental procedure, the pure Bi2WO6 were synthesized without adding P25, which was labelled as BWO.
2.3. Characterization
Powder X-ray diffraction (XRD, Rigaku D/MAX 2500 V, Rigaku Corporation, Japan) was used to investigate the crystal phase structure. The wavelength of the copper target was λ = 0.15418 nm, and the working current and accelerating voltage were 150 mA and 40 kV, respectively. The appearance and micro shape of the catalysts were analyzed by scanning electron microscope (SEM, Sigma 300, Carl Zeiss, Germany) and scanning transmission electron microscope (TEM, Tecnai F20, FEI, USA), respectively. The accelerating voltages of SEM and TEM were 20 kV and 200 kV, respectively. The sample's elemental distribution was detected using energy dispersive spectroscopy (EDS) of Oxford Instrument. The chemical environments of elements on the catalyst's surface were characterized by X-ray photoelectron spectroscopy (XPS, ESCALAB 250XI, Waltham, USA). Before deconvolution, calibration was performed, where all the binding energies were charged and corrected by setting the adventitious carbon signal (C 1s) to 284.8 eV. Raman spectrometer (inVia Reflex 1500080S, Nottingham, UK) was employed to characterize the surface structure of the catalysts. Fourier Transform infrared spectroscopy (FT-IR) spectra were carried out with a Fourier transform infrared spectrometer (FT-IR, Nicolet iS50, Thermo Fisher Scientific, USA). UV-vis diffuse reflectance spectra (DRS, UV-3600Plus, SHIMADZU, Japan) were obtained to investigate the light-harvesting ability of the catalysts. Photoluminescence spectra (PL, FL3C-111 TCSPC, HORIBA, Japan) was obtained to study carriers' separation of samples.
2.4. Photoelectrochemical measurement
The photocatalyst samples' photoelectrochemical (PEC) properties were evaluated using an electrochemical workstation (CHI660D, Chenhua Instrument, China) with a three-electrode system. The samples coated on pure fluorine-doped tin oxide (FTO) glasses acted as working electrodes, while Pt and Ag/AgCl electrodes acted as counter and reference electrodes, respectively, with 0.5 M Na2SO4 as the electrolyte solution. A full spectrum Xe lamp (300 W, PLS-SXE300, Perfect light, China) was adopted as the light source.
2.5. Photocatalytic CO2 reduction experiments
The photocatalytic CO2 reduction reaction (CO2RR) was realized in a 100 mL confined device equipped with circulating condensate water and a 300 W Xe lamp (UV light spectrum range of 320–780 nm). In a typical process, 20 mg photocatalyst was ultrasonically dispersed into ethanol and dispensed onto a 30 mm × 30 mm flat quartz plate. After thoroughly drying the catalyst, the flat quartz plate was placed into the reactor. Then, 40 mL 0.1 M NaHCO3 aqueous solution was injected to react. Before irradiation, the reaction mixture was purged with bubbling CO2 for 40 min in the dark to ensure adsorption–desorption equilibrium was attained on the photocatalyst surface. The reactor's temperature was controlled at 25 °C by cooling water circulation. Then the reactor was continuously irradiated with UV-vis light using a 300 W Xe lamp. After an hour of reaction, the gaseous products were analyzed by a gas chromatograph (GC-2014C, SHIMADZU, Japan) equipped with a flame ionization detector (FID) and a thermal conductivity detector (TCD).
2.6. In situ diffuse reflectance infrared Fourier transform spectra (DRIFTS) measurement
In situ diffuse reflectance infrared Fourier transform spectra (DRIFTS) were recorded on a Fourier transform infrared spectrometer (FT-IR, Nicolet iS50, Thermo Fisher Scientific, USA). The spectra in absorbance units were acquired with a resolution of 4 cm−1 using 32 scans. Firstly, samples were purged with Ar for an hour, and then a mixture of CO2 and water vapor was taken into the chamber at 5 mL min−1 for 20 min. After that, samples were under illumination (300 W Xe lamp) for 40 min. The spectra were collected every 10 min.
3 Results and discussion
3.1. Structural characterization
The crystalline phases of P25, BWO, and P25/BWO nanocomposites with different contents were analyzed by XRD. As shown in Fig. 1a, the diffraction peaks located at 28.3°, 32.8°, 47.3°, 56.1°, and 58.4° belonged to the (131), (200), (202), (133), and (262) crystalline planes of BWO (JCPDS no. 39-0256), respectively.38 The diffraction peaks located at 25.3°, 37.8°, 48.0°, 55.0°, and 62.7° assigned to the (101), (004), (200), (211), and (204) crystal planes of anatase TiO2 (JCPDS no. 21-1272), respectively. The diffraction peaks located at 27.4°, 36.0°, and 41.2° corresponded to the (110), (101), and (111) crystal planes of rutile TiO2 (JCPDS no. 21-1276), respectively.39 The peaks of pure P25 and BWO were sharp and had high intensity, indicating that the prepared samples had good crystallinity. Only the diffraction peaks of P25 and BWO appeared in the X-ray diffractograms of all four nanocomposites. The larger the percentage of P25 content, the stronger the intensity of the characteristic peaks of P25 in the nanocomposites. The results indicated that the combination of P25 and BWO had high purity and crystallinity.
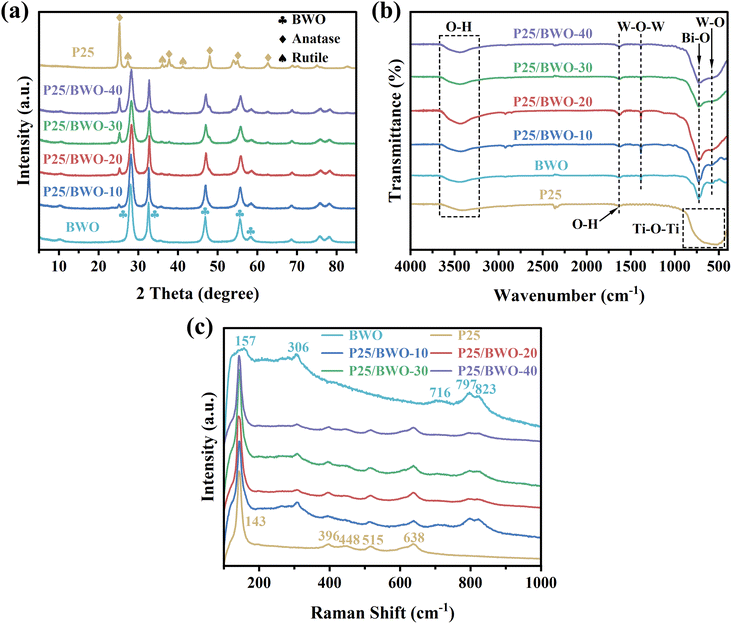 |
| Fig. 1 (a) XRD patterns, (b) FT-IR spectra, and (c) Raman spectra of the as-prepared samples. | |
FT-IR investigated the specific functional groups of all samples. As shown in Fig. 1b, in the FT-IR spectra of BWO, the peaks at 3447 and 1636 cm−1 were caused by O–H vibrations. In addition, the peaks at 1389, 728, and 576 cm−1 corresponded to W–O–W stretching, Bi–O stretching, and W–O stretching, respectively.40 In the FT-IR spectra of P25, the stretching vibrational band from 850 to 433 cm−1 belonged to the Ti–O–Ti bond of P25.21 The four P25/BWO nanocomposites with different contents had both the characteristic absorption peaks of P25 and BWO. Meanwhile, their FT-IR spectra were more similar to that of BWO, which may be because BWO was the main component in the nanocomposites. The above experimental results can prove the successful synthesis of P25/BWO nanocomposites.
Raman spectra were used to investigate the structure of the samples further. Fig. 1c shows the Raman spectra of P25, BWO, and P25/BWO nanocomposites with different contents of wavenumber in the range of 100 to 1000 cm−1. Generally, the typical characteristic peaks of Raman spectra of BWO were located at 157, 306, 716, 797, and 823 cm−1. Among them, the peak at 157 cm−1 was attributed to the external vibration of WO66−.41 The vibrational peaks in the range of 600 to 1000 cm−1 were attributed to the vibrational stretching mode of the W–O bond. In contrast, the vibrational peaks near 306 cm−1 were mainly attributed to the vibrational stretching mode between Bi3+ and WO66−.42 The typical characteristic peaks of Raman spectra of anatase TiO2 were located at 143, 396, 515, and 638 cm−1, and rutile TiO2 was located at 448 cm−1. The O–Ti–O variable angle vibration peak with the highest intensity was 143 cm−1. In the Raman spectra of the four P25/BWO nanocomposites with different contents, only the typical characteristic peaks of P25 and BWO appeared. The results indicated that there was a strong interaction between P25 and BWO.
3.2. Morphological characterization
SEM and TEM analyzed the morphology and microstructure of the samples. As shown in Fig. 2a–d, pure P25 was a nanoparticle with agglomerated morphology, and BWO was a nanosheet structure with a hierarchical structure. The formation of this hierarchical structure may be due to the anisotropy and self-assembly of BWO nanosheets. For the P25/BWO-20 heterojunction, many P25 nanoparticles can be uniformly distributed in the BWO nanosheets (Fig. 2e and f). From the high-resolution electron microscopy images (Fig. 2j), it can be seen that the two components, P25 and BWO, were in close contact, forming a clear heterojunction interface rather than a simple physical mixing. The lattice spacing of 0.27 nm was assigned to the (020) crystal plane of BWO,43 and the lattice spacing of 0.352 nm corresponded to the (101) plane of anatase TiO2.44 Elemental EDS spectral analysis (Fig. 2k and S1†) revealed the elemental composition and distribution in the P25/BWO-20 heterojunction, and the elemental profiles of Bi, W, Ti, and O were uniformly distributed, indicating that the P25 nanoparticles are uniformly distributed in the nanocomposites. These results indicated that we successfully constructed P25/BWO heterojunction.
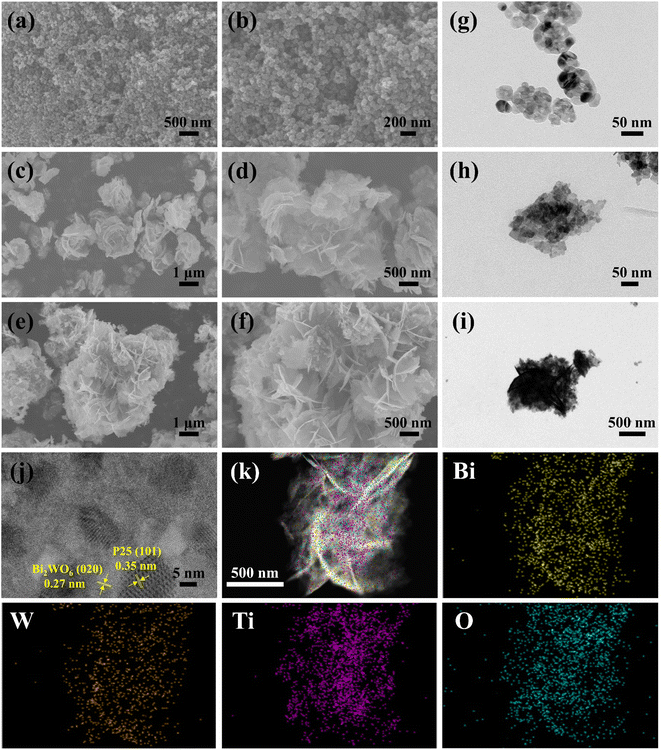 |
| Fig. 2 (a–f) SEM images, and (g–i) TEM images of P25, BWO and P25/BWO-20 heterojunction; (j) HRTEM image, and (k) STEM and the corresponding elemental mapping images of P25/BWO-20 heterojunction. | |
3.3. Surface and composition analysis
The chemical composition and surface valence states of P25, BWO, and P25/BWO-20 heterojunction were investigated by XPS. Full-spectrum analysis of different samples (Fig. S2†) showed that the P25/BWO-20 heterojunction was mainly composed of four elements, Bi, W, Ti, and O. In order to elucidate the interfacial interaction between P25 and BWO, the high-resolution XPS spectra of different samples of Bi 4f, W 4f, Ti 2p, and O 1s were also investigated. The Bi 4f spectrum of BWO (Fig. 3a) had two peaks at 159.40 and 164.70 eV, which were attributed to Bi 4f7/2 and Bi 4f5/2 of the Bi3+ ion, respectively. As displayed in Fig. 3b clearly, the peaks at 35.70 and 37.80 eV of the W 4f spectrum corresponded to W 4f7/2 and W 4f5/2 of the W6+ ion in BWO, respectively.45 As shown in Fig. 3d, the characteristic peaks with binding energies of 458.45 and 464.25 eV were designated as Ti 2p3/2 and Ti 2p1/2, respectively, indicating the presence of Ti4+.21 Compared to the pristine BWO, the P25/BWO-20 heterojunction's binding energies were negatively shifted by 0.1 and 0.15 eV for Bi 4f and W 4f, respectively. Specifically, the negative shift in binding energy indicates an increase in electron density.46 Therefore, the migration path of electrons in the composite can be determined by shifting the XPS binding energy. In this case, the negative shifts of the binding energy of Bi 4f and W 4f indicated that BWO acts as an electron acceptor in the P25/BWO-20 heterojunction. In order to balance the electron redistribution in the P25/BWO-20 heterojunction, Ti in P25 will be electron deficient. The latter was confirmed by the positive shift of the Ti 2p binding energy in the P25/BWO-20 heterojunction, which implied a decrease in electron density. In addition, the O 1s spectrum of the P25/BWO-20 heterojunction (Fig. 3c) can be divided into two peaks at 530.15 and 531.00 eV, which were attributed to the lattice oxygen and surface hydroxyl group, respectively.47,48 By observing the binding energy, the results indicated that the photogenerated electrons migrate from P25 to BWO during photoexcitation, where P25 was the electron donor and BWO was the electron acceptor. The detailed mechanism of the electron transfer pathway in the P25/BWO-20 heterojunction will be further elaborated later.
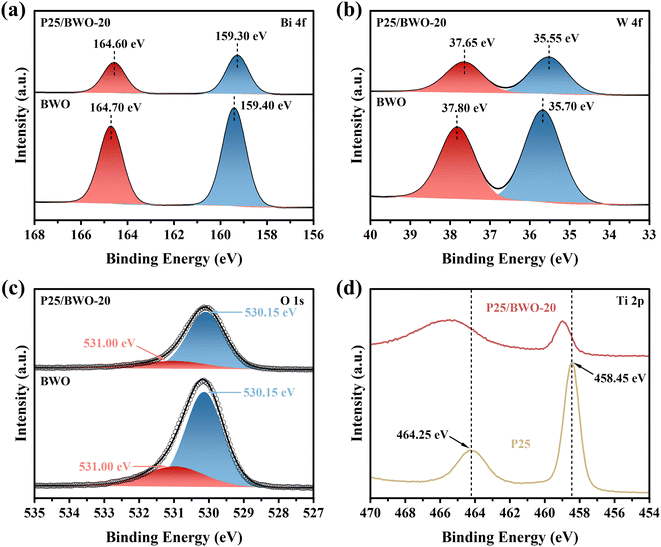 |
| Fig. 3 High-resolution XPS spectra of (a) Bi 4f, (b) W 4f, and (c) O 1s in P25/BWO-20 heterojunction and BWO, and (d) Ti 2p in P25/BWO-20 heterojunction and P25. | |
3.4. Optical properties and energy band structure
The optical absorbance and energy band structure of P25, BWO, and P25/BWO nanocomposites with different contents were investigated by UV-vis DRS. As shown in Fig. 4a and S3,† P25/BWO-20 heterojunction exhibits a feature intermediate between its constituent components, and the absorption edges of P25 and BWO are 414 nm and 449 nm, respectively. Compared with BWO, the absorption edges of the P25/BWO-20 heterojunction showed a slight red-shift and steep curve due to the band gap jump between P25 and BWO. The visible absorption range was extended to 464 nm, indicating that the synthesized nanocomposites had significantly higher light utilization and could absorb solar energy more efficiently and generate electron–hole pairs for photocatalytic CO2 reduction. Furthermore, based on the Kubelka–Munk equation:49where α is the absorption coefficient, ℏ is Planck's constant, ν is the incident light frequency, k is a constant, Eg is the bandgap energy, and the value of n for the indirect bandgap semiconductors is 1. Fig. 4b shows the relationship between (αℏν)1/2 and ℏν. It was inferred that the band gap widths of P25, BWO, and P25/BWO-20 heterojunction were 3.18, 2.75, and 2.62 eV, respectively. In summary, the enhanced light absorption and narrower forbidden bandwidths were favorable to improve the photocatalytic activity.
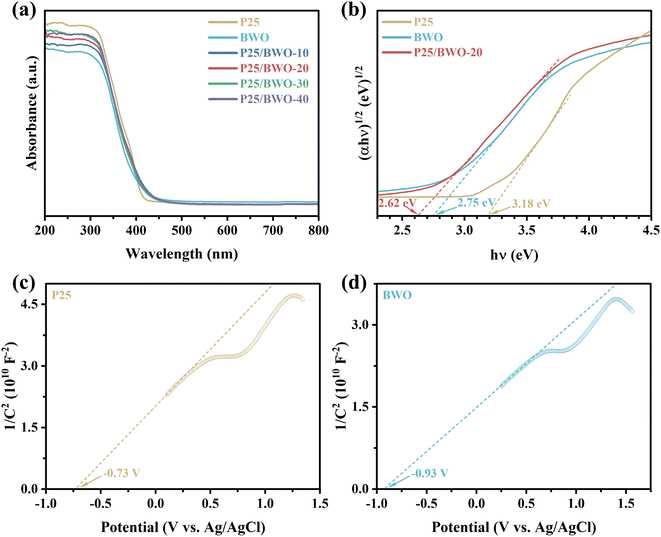 |
| Fig. 4 (a) UV-vis DRS, and (b) Tauc plots of P25, BWO and P25/BWO-20 heterojunction; (c and d) Mott–Schottky plots of P25 and BWO. | |
Mott–Schottky analysis was performed by impedance-potential testing in a typical three-electrode system to determine the semiconductor type and electronic energy band structure of the sample, where Mott–Schottky diagrams were plotted according to the following equations:50
For p-type semiconductors:
|
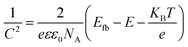 | (2) |
For n-type semiconductors:
|
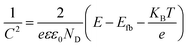 | (3) |
where
C is the capacitance of the space charge layer,
e is the electronic charge (
e = 1.602 × 10
−19 C),
ε is the dielectric constant of the semiconductor,
ε0 is the permittivity in vacuum (
ε0 = 8.8510
−14 F cm
−1),
NA and
ND are the numbers of acceptors and donors in p-type and n-type semiconductors, respectively,
E is the applied potential,
Efb is the flat-band potential,
KB is the Boltzmann's constant, and
T is the absolute temperature. Therefore,
Efb can be estimated by extrapolating the Mott–Schottky curves to the
x-axis (
i.e., 1/
C2 = 0).
As shown in Fig. 4c and d, the slopes of both P25 and BWO are positive, indicating that they were both n-type semiconductors. The experimental potential measured relative to the Ag/AgCl reference electrode can be converted to the standard hydrogen electrode potential by the Nernst equation:51
|
Efb(vs. NHE) = Efb(vs. Ag/AgCl) + E0Ag/AgCl + 0.059pH
| (4) |
where
Efb (
vs. NHE) is the standard hydrogen electrode potential,
Efb (
vs. Ag/AgCl) is the experimental potential measured against the Ag/AgCl reference electrode and
E0Ag/AgCl is the standard potential of Ag/AgCl at 298 K (0.1976 V). Considering that the conduction band edge of n-type semiconductors is generally 0.3 V lower than the standard hydrogen electrode potential,
52 the conduction bands of P25 and BWO were calculated to be −0.42 and −0.62 eV, respectively, and the valence bands can be determined by eqn
(5):
53
The results showed that the valence bands of P25 and BWO were 2.76 and 2.13 eV, respectively.
3.5. Carriers' separation
In addition to the semiconductor energy band structure, the effective electron–hole pair separation is also one of the critical factors affecting photocatalytic efficiency. The samples' charge separation and transfer capability were investigated using transient photocurrent response spectra and AC impedance spectra. Firstly, the transient photocurrent response of the samples was examined by the on–off cycle of simulated solar irradiation. Briefly, photogenerated electrons were transferred to the back contact to generate the photocurrent response.54,55 Simultaneously, holes migrate to the interface between the photocatalyst and the electrolyte and are then captured by the reduced species in the electrolyte under illumination. Since the electrolyte and electrodes used are the same each time, the instantaneous photocurrent intensity exhibited by the sample reflects the efficiency of photogenerated carrier generation and separation.56,57 As shown in Fig. 5a, the P25/BWO-20 heterojunction exhibited a higher photocurrent response than pure P25 and BWO, indicating that the P25/BWO-20 heterojunction had better charge separation and transfer efficiency. It was worth mentioning that the photocurrent intensities of the samples were consistent with their results in photocatalytic CO2 reduction experiments, indicating that separating electron–hole pairs is a decisive factor in photocatalytic performance. In addition, in the absence of light, the photocurrent intensity of the samples was decaying, which can be attributed to the slow discharge of the accumulated charge in the middle layer of the electrodes.58 The AC impedance spectra can reflect the charge transfer kinetics at the electrode/electrolyte interface. The smaller the arc radius of the curve, the lower the reaction electron transfer resistance and the faster the photogenerated carrier transfer rate, leading to a more superior interfacial charge transfer process.59,60 As shown in Fig. 5b, the P25/BWO-20 heterojunction had the smallest arc radius, indicating a faster charge transfer rate at the interface. The results of transient photocurrent response and AC impedance spectra indicated that the presence of heterojunction in the P25/BWO nanocomposites facilitates the electron transfer process, which effectively inhibited the recombination of electron–hole pairs and significantly improved the photocatalytic activity of the samples. Steady-state photoluminescence spectra further verified the charge separation and transferred efficiency of the samples after excitation at 308 nm. As shown in Fig. 5c, pure P25 and BWO had strong fluorescence emissions at ∼468 nm, indicating a severe recombination of carriers. However, the fluorescence intensity significantly decreased after forming P25/BWO heterojunction, indicating that the formation of a heterojunction can effectively suppress the recombination of electron–hole pairs and facilitate the photocatalytic CO2 reduction.61 Therefore, these results suggested that the construction of heterojunction is an effective strategy to improve photocatalytic activity.
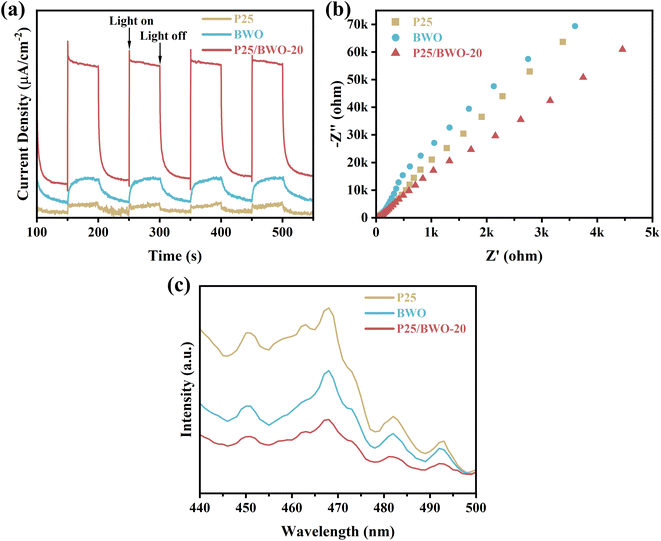 |
| Fig. 5 (a) Transient photocurrent, (b) EIS plots, and (c) PL spectra of P25, BWO and P25/BWO-20 heterojunction. | |
3.6. Photocatalytic activities
The photocatalytic CO2 reduction reaction (CO2RR) was carried out in a closed quartz glass reaction apparatus without adding any co-catalysts, photosensitizers, or sacrificial agents, and the photocatalytic reactions of all samples were studied in the gas–solid system under simulated solar irradiation. As shown in Fig. 6a, pure P25, and BWO photocatalytic activities were not high due to poor charge separation and severe charge recombination. Meanwhile, the main products of both P25 and BWO were CO. When the P25 nanoparticles and BWO nanosheets were compounded, the P25/BWO nanocomposites exhibited stronger photocatalytic activity. The maximum yield of CO reached 15.815 μmol g−1 h−1 on the P25/BWO nanocomposites, which was 4.04 and 2.80 times higher than that of P25 and BWO, respectively, indicating the formation of a heterojunction between the P25 and BWO interfaces. In addition, the content of P25 in the P25/BWO nanocomposites was optimized, and the results showed that the photocatalytic activity of the samples was enhanced with the increase of P25 content in the nanocomposites, showing a volcanic trend, and the optimal content of P25 in the nanocomposites could be concluded to be 20%. Optimizing the content of P25 can improve the light absorption efficiency of the P25/BWO heterojunction. However, the excessive P25 will block the catalytic active center of the heterojunction and reduce the photocatalytic activity. The product selectivity can observe the effect of the loading of P25 in the nanocomposites on the reduction of CO2. Based on the number of electrons transferred to produce each reduction product (2e− for CO and 8e− for CH4), the selectivity of CO is defined as follows:62 |
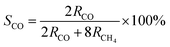 | (6) |
where SCO is the selectivity of CO, RCO and RCH4 are the yields of CO and CH4, respectively. Fig. 6b shows the calculated selectivity of CO and CH4 for all samples. The selectivity of pure P25 and BWO for CO was 85.69% and 94.58%, respectively. For the P25/BWO nanocomposites, the selectivity of CO was ∼90%. The results indicated that P25/BWO nanocomposites have a high selectivity for CO, mainly due to the easy desorption of CO and the need for more complex intermediates for CH4 generation. In addition, a series of control experiments (Fig. 6c) were performed in the absence of light, CO2, or photocatalyst, where the product yields were negligible, which could indicate that light is the driving force of the CO2 reduction process and that the carbon source of the product CO was derived from CO2.
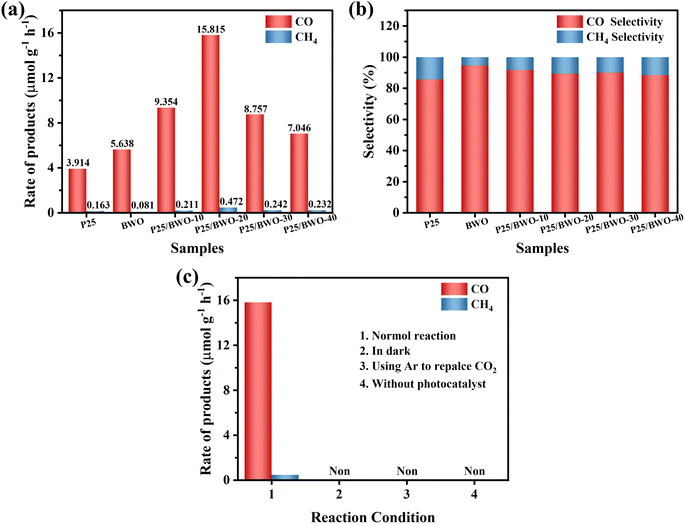 |
| Fig. 6 (a) Photocatalytic activity test, and (b) selectivity of the as-prepared samples; (c) photocatalysis under different conditions performance chart of P25/BWO-20 heterojunction. | |
3.7. Photocatalytic mechanism of CO2 reduction
Based on the results above, the charge transfer path at the P25/BWO heterojunction interface may follow the Z-scheme mechanism. As shown in Scheme 2, under simulated solar irradiation, P25 and BWO produce photogenerated electrons and holes in their conduction band (CB) and valence band (VB) positions, respectively, under photoexcitation, where the photogenerated electrons in the CB of P25 will be transferred to the VB of BWO and recombine with the holes produced in the VB. In contrast, the holes in the VB of P25 will react with water (H2O) to form hydroxyl groups (·OH) and protons (H+). The photogenerated electrons are then retained in the CB of BWO, which can convert CO2 into CO and CH4. This Z-scheme charge transfer mechanism enables the P25/BWO nanocomposites to have an efficient charge separation capability, which significantly improves the photocatalytic CO2 reduction performance of the P25/BWO nanocomposites. It is well known that the different structural characteristics and reaction conditions of catalysts lead to different reaction products. In the photocatalytic CO2 reduction reaction (CO2RR), eight electrons (8e−) are required to generate one CH4 molecule, while only two electrons (2e−) are required to generate one CO molecule. Therefore, it will be more challenging to generate CH4. Converting CO2 to CO may be more kinetically advantageous in our photocatalytic reaction system. Therefore, in the present work, the P25/BWO nanocomposites can convert CO2 into CO with very high selectivity.
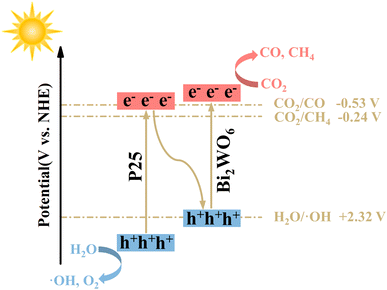 |
| Scheme 2 Proposed mechanism of CO2 photoreduction due to heterojunction formation within over P25/BWO nanocomposites. | |
In order to explore the process and intermediates of efficient and selective reduction of CO2 to CO for photocatalysts in more detail, in situ diffuse reflectance infrared Fourier transform spectra (DRIFTS) was carried out. Fig. 7a, b and S4† showed the carbon-based groups' dynamic changes on the photocatalysts' surface within 0–40 min of illumination. Several characteristic peaks attributed to bicarbonate (HCO3−, 1456, 1419, and 1396 cm−1), monodentate carbonate (m-CO32−, 1559, 1338, and 1298 cm−1), bidentate carbonate (b-CO32−, 1636 and 1520 cm−1), carboxylate (COO−, 1714 and 1243 cm−1), and active ·CO2− intermediates (1695 cm−1) were detected. Hence, the photocatalytic CO2 reduction can be significantly enhanced due to those important intermediates. In comparison, the signal changes of carbonate and bicarbonate species during reaction processes in the spectra for P25 and BWO are much weaker, indicating a poor ability of CO2 conversion under the illumination of P25 and BWO. Moreover, the significant peak absorbance of CO* (2077 cm−1) supports the formation of CO during photocatalytic CO2 reduction.7,63–65
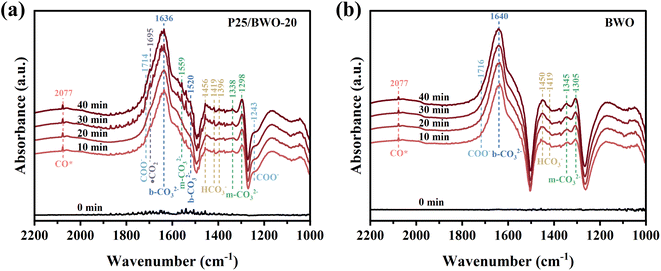 |
| Fig. 7 (a and b) In situ DRIFTS spectra of surface adsorbed CO2 species and photocatalytic CO2 reduction intermediates of P25/BWO-20 heterojunction and BWO. | |
4 Conclusions
In summary, we prepared P25/BWO nanocomposites by a one-step in situ hydrothermal method for efficient photocatalytic CO2 reduction. Their respective interlaced energy band structures promote charge separation and inhibit charge recombination. The P25/BWO nanocomposites exhibited strong synergy between the semiconductor components, leading to good activity and selectivity for the photoreduction of CO2 to CO under simulated solar irradiation. The samples had the highest activity when the content of P25 in the nanocomposites is 20%, when maximization of the heterojunction interface may be achieved. The CO yield of the P25/BWO-20 heterojunction reached 15.815 μmol g−1 h−1, which was 4.04 and 2.80 times higher than that of pure P25 and BWO, respectively. In addition, the P25/BWO-20 heterojunction showed a high selectivity of 89.33% for CO, mainly due to the easy desorption of CO and the need for more complex intermediates for CH4 generation. This work presents an effective strategy to facilitate charge separation by constructing Z-scheme heterojunction. This strategy opens up new opportunities for designing and developing other novel Bi2WO6-based photocatalysts.
Ethical approval
The experimental protocols were not involved by any animals or human care.
Data availability
The datasets used and/or analyzed during the current study are available from the corresponding author on reasonable request.
Author contributions
D. Liu: Investigation, Data Curation, writing – original draft; M. Zeng: validation, formal analysis, writing – review & editing; Z. Li: methodology; Z. Zhu: resources; Y. Chen: data curation; K. Thummavichai: supervision; O. Ola: investigation, formal analysis; N. Wang: project administration; Y. Zhu: conceptualization, funding acquisition.
Conflicts of interest
The authors have no conflicts of interest to declare.
Acknowledgements
This work was supported by National Natural Science Foundation (51972068), Natural Science Foundation of Guangxi Province (2021GXNSFBA076003), Guangxi Key Laboratory of Manufacturing Systems and Advanced Manufacturing Technology (20-065-40S007), the Interdisciplinary Scientific Research Foundation of Guangxi University (Grant No. 2022JCA002).
References
- S. Cestellos-Blanco, H. Zhang, J. M. Kim, Y.-x. Shen and P. Yang, Photosynthetic semiconductor biohybrids for solar-driven biocatalysis, Nat. Catal., 2020, 3, 245–255 CrossRef CAS
. - X. Wang, J. He, J. Li, G. Lu, F. Dong, T. Majima and M. Zhu, Immobilizing perovskite CsPbBr3 nanocrystals on Black phosphorus nanosheets for boosting charge separation and photocatalytic CO2 reduction, Appl. Catal., B, 2020, 277, 119230 CrossRef CAS
. - Y. Yamazaki, M. Miyaji and O. Ishitani, Utilization of Low-Concentration CO2 with Molecular Catalysts Assisted by CO2-Capturing Ability of Catalysts, Additives, or Reaction Media, J. Am. Chem. Soc., 2022, 144(15), 6640–6660 CrossRef CAS PubMed
. - S. Yoshino, T. Takayama, Y. Yamaguchi, A. Iwase and A. Kudo, CO2 Reduction Using Water as an Electron Donor over Heterogeneous Photocatalysts Aiming at Artificial Photosynthesis, Acc. Chem. Res., 2022, 55(7), 966–977 CrossRef CAS PubMed
. - W. Zhang, A. R. Mohamed and W.-J. Ong, Z-Scheme Photocatalytic Systems for Carbon Dioxide Reduction: Where Are We Now?, Angew. Chem., Int. Ed., 2020, 59(51), 22894–22915 CrossRef CAS PubMed
. - P. Zhou, Y. Chao, F. Lv, J. Lai, K. Wang and S. Guo, Designing noble metal single-atom-loaded two-dimension photocatalyst for N2 and CO2 reduction via anion vacancy engineering, Sci. Bull., 2020, 65(9), 720–725 CrossRef CAS
. - X.-X. Li, L. Zhang, L. Yuan, T. Wang, L.-Z. Dong, K. Huang, J. Liu and Y.-Q. Lan, Constructing crystalline redox catalyst to achieve efficient CO2 photoreduction reaction in water vapor, Chem. Eng. J., 2022, 442, 136157 CrossRef CAS
. - T. Inoue, A. Fujishima, S. Konishi and K. Honda, Photoelectrocatalytic reduction of carbon dioxide in aqueous suspensions of semiconductor powders, Nature, 1979, 277, 637–638 CrossRef CAS
. - X. Cui, J. Wang, B. Liu, S. Ling, R. Long and Y. Xiong, Turning Au Nanoclusters Catalytically Active for Visible-Light-Driven CO2 Reduction through Bridging Ligands, J. Am. Chem. Soc., 2018, 140(48), 16514–16520 CrossRef CAS
. - F. Zhang, Y.-H. Li, M.-Y. Qi, Z.-R. Tang and Y.-J. Xu, Boosting the activity and stability of Ag-Cu2O/ZnO nanorods for photocatalytic CO2 reduction, Appl. Catal., B, 2020, 268, 118380 CrossRef CAS
. - J. Hao, B. Qi, J. Wei, D. Li and F. Zeng, A Z-scheme Cu2O/WO3 heterojunction for production of renewable hydrocarbon fuel from carbon dioxide, Fuel, 2021, 287, 119439 CrossRef CAS
. - C. B. Hiragond, J. Lee, H. Kim, J.-W. Jung, C.-H. Cho and S.-I. In, A novel N-doped graphene oxide enfolded reduced titania for highly stable and selective gas-phase photocatalytic CO2 reduction into CH4: an in-depth study on the interfacial charge transfer mechanism, Chem. Eng. J., 2021, 416, 127978 CrossRef CAS
. - N. Lin, Y. Lin, X. Qian, X. Wang and W. Su, Construction of a 2D/2D WO3/LaTiO2N Direct Z-Scheme Photocatalyst for Enhanced CO2 Reduction Performance Under Visible Light, ACS Sustainable Chem. Eng., 2021, 9(40), 13686–13694 CrossRef CAS
. - L. O. Paulista, J. Albero, R. J. E. Martins, R. A. R. Boaventura, V. J. P. Vilar, T. F. C. V. Silva and H. García, Turning Carbon Dioxide and Ethane into Ethanol by Solar-Driven Heterogeneous Photocatalysis over RuO2- and NiO-co-Doped SrTiO3, Catalysts, 2021, 11(4), 461 CrossRef CAS
. - S. Gong, F. Rao, W. Zhang, Q.-U. Hassan, Z. Liu, J. Gao, J. Lu, M. Hojamberdiev and G. Zhu, Au nanoparticles loaded on hollow BiOCl microstructures boosting CO2 photoreduction, Chin. Chem. Lett., 2022, 33(9), 4385–4388 CrossRef CAS
. - M. K. Hussien, A. Sabbah, M. Qorbani, M. H. Elsayed, P. Raghunath, T.-Y. Lin, S. Quadir, H.-Y. Wang, H.-L. Wu, D.-L. M. Tzou, M.-C. Lin, P.-W. Chung, H.-H. Chou, L.-C. Chen and K.-H. Chen, Metal-free four-in-one modification of g-C3N4 for superior photocatalytic CO2 reduction and H2 evolution, Chem. Eng. J., 2022, 430, 132853 CrossRef
. - S. Cao, B. Shen, T. Tong, J. Fu and J. Yu, 2D/2D Heterojunction of Ultrathin MXene/Bi2WO6 Nanosheets for Improved Photocatalytic CO2 Reduction, Adv. Funct. Mater., 2018, 28(21), 1800136 CrossRef
. - C. Gao, J. Wang, H. Xu and Y. Xiong, Coordination chemistry in the design of heterogeneous photocatalysts, Chem. Soc. Rev., 2017, 46(10), 2799–2823 RSC
. - S. Wageh, A. A. Al-Ghamdi, R. Jafer, X. Li and P. Zhang, A new heterojunction in photocatalysis: S-scheme heterojunction, Chin. J. Catal., 2021, 42(5), 667–669 CrossRef CAS
. - C.-W. Huang, C.-H. Liao, J. C. S. Wu, Y.-C. Liu, C.-L. Chang, C.-H. Wu, M. Anpo, M. Matsuoka and M. Takeuchi, Hydrogen generation from photocatalytic water splitting over TiO2 thin film prepared by electron beam-induced deposition, Int. J. Hydrogen Energy, 2010, 35(21), 12005–12010 CrossRef CAS
. - Z. Xiong, Y. Zhao, J. Zhang and C. Zheng, Efficient photocatalytic reduction of CO2 into liquid products over cerium doped titania nanoparticles synthesized by a sol-gel auto-ignited method, Fuel Process. Technol., 2015, 135, 6–13 CrossRef CAS
. - L. Wang, Z. Zhang, Q. Han, Y. Liu, J. Zhong, J. Chen, J. Huang, H. She and Q. Wang, Preparation of CdS-P25/ZIF-67 composite material and its photocatalytic CO2 reduction performance, Appl. Surf. Sci., 2022, 584, 152645 CrossRef CAS
. - T. Wang, Y. Zhang, J. Pan, B. Li, L. Wu and B. Jiang, Hydrothermal reduction of commercial P25 photocatalysts to expand their visible-light response and enhance their performance for photodegrading phenol in high-salinity wastewater, Appl. Surf. Sci., 2019, 480, 896–904 CrossRef CAS
. - K. Nakata and A. Fujishima, TiO2 photocatalysis: Design and applications, J. Photochem. Photobiol., C, 2012, 13(3), 169–189 CrossRef CAS
. - M. Pelaez, N. T. Nolan, S. C. Pillai, M. K. Seery, P. Falaras, A. G. Kontos, P. S. M. Dunlop, J. W. J. Hamilton, J. A. Byrne, K. O'Shea, M. H. Entezari and D. D. Dionysiou, A review on the visible light active titanium dioxide photocatalysts for environmental applications, Appl. Catal., B, 2012, 125, 331–349 CrossRef CAS
. - J. Low, J. Yu and W. Ho, Graphene-Based Photocatalysts for CO2 Reduction to Solar Fuel, J. Phys. Chem. Lett., 2015, 6(21), 4244–4251 CrossRef CAS
. - P. Kar, S. Farsinezhad, N. Mahdi, Y. Zhang, U. Obuekwe, H. Sharma, J. Shen, N. Semagina and K. Shankar, Enhanced CH4 yield by photocatalytic CO2 reduction using TiO2 nanotube arrays grafted with Au, Ru, and ZnPd nanoparticles, Nano Res., 2016, 9(11), 3478–3493 CrossRef CAS
. - S. Kawamura, H. Zhang, M. Tamba, T. Kojima, M. Miyano, Y. Yoshida, M. Yoshiba and Y. Izumi, Efficient volcano-type dependence of photocatalytic CO2 conversion into methane using hydrogen at reaction pressures up to 0.80 MPa, J. Catal., 2017, 345, 39–52 CrossRef CAS
. - K. Li, C. Teng, S. Wang and Q. Min, Recent Advances in TiO2-Based Heterojunctions for Photocatalytic CO2 Reduction With Water Oxidation: A Review, Front. Chem., 2021, 9, 637501 CrossRef CAS PubMed
. - N. Kim, R.-N. Vannier and C. P. Grey, Detecting Different Oxygen-Ion Jump Pathways in Bi2WO6 with 1- and 2-Dimensional 17O MAS NMR Spectroscopy, Chem. Mater., 2005, 17(8), 1952–1958 CrossRef CAS
. - N. Zhang, R. Ciriminna, M. Pagliaro and Y.-J. Xu, Nanochemistry-derived Bi2WO6 nanostructures: towards production of sustainable chemicals and fuels induced by visible light, Chem. Soc. Rev., 2014, 43(15), 5276–5287 RSC
. - M. Sun, X. Dong, B. Lei, J. Li, P. Chen, Y. Zhang and F. Dong, Graphene oxide mediated co-generation of C-doping and oxygen defects in Bi2WO6 nanosheets: a combined DRIFTS and DFT investigation, Nanoscale, 2019, 11(43), 20562–20570 RSC
. - M. Li, L. Zhang, X. Fan, Y. Zhou, M. Wu and J. Shi, Highly selective CO2 photoreduction to CO over g-C3N4/Bi2WO6 composites under visible light, J. Mater. Chem. A, 2015, 3(9), 5189–5196 RSC
. - J. Hu, D. Chen, Z. Mo, N. Li, Q. Xu, H. Li, J. He, H. Xu and J. Lu, Z-Scheme 2D/2D Heterojunction of Black Phosphorus/Monolayer Bi2WO6 Nanosheets with Enhanced Photocatalytic Activities, Angew. Chem., Int. Ed., 2019, 58(7), 2073–2077 CrossRef CAS PubMed
. - H. Salari, Facile template-free synthesis of 3D flower-like Bi2WO6/MoO3 nanocomposites with ultra-thin sheets and their associated photocatalytic properties under visible light irradiation, J. Photochem. Photobiol., A, 2019, 385, 112069 CrossRef CAS
. - Y. Zhang, C. Xu, F. Wan, D. Zhou, L. Yang, H. Gu and J. Xiong, Synthesis of flower-like Bi2Sn2O7/Bi2WO6 hierarchical composites with enhanced visible light photocatalytic performance, J. Alloys Compd., 2019, 788, 1154–1161 CrossRef CAS
. - J. Tian, L. Wei, Z. Ren, J. Lu and J. Ma, The facile fabrication of Z-scheme Bi2WO6-P25 heterojunction with enhanced photodegradation of antibiotics under visible light, J. Environ. Chem. Eng., 2021, 9(5), 106167 CrossRef CAS
. - J. Wang, L. Tang, G. Zeng, Y. Deng, Y. Liu, L. Wang, Y. Zhou, Z. Guo, J. Wang and C. Zhang, Atomic scale g-C3N4/Bi2WO6 2D/2D heterojunction with enhanced photocatalytic degradation of ibuprofen under visible light irradiation, Appl. Catal., B, 2017, 209, 285–294 CrossRef CAS
. - K. Kalantari, M. Kalbasi, M. Sohrabi and S. J. Royaee, Enhancing the photocatalytic oxidation of dibenzothiophene using visible light responsive Fe and N co-doped TiO2 nanoparticles, Ceram. Int., 2017, 43(1), 973–981 CrossRef CAS
. - S. Xiong, S. Bao, W. Wang, J. Hao, Y. Mao, P. Liu, Y. Huang, Z. Duan, Y. Lv and D. Ouyang, Surface oxygen vacancy and graphene quantum dots co-modified Bi2WO6 toward highly efficient photocatalytic reduction of CO2, Appl. Catal., B, 2022, 305, 121026 CrossRef CAS
. - J. Cheng, S. Shi, T. Tang, S. Tian, W. Yang and D. Zeng, Controllable topological transformation from BiOCl hierarchical microspheres to Bi2WO6 superstructures in the Bi–W–Cl–O system, J. Alloys Compd., 2015, 643, 159–166 CrossRef CAS
. - M. Maçzka, L. Macalik, K. Hermanowicz, L. Kępiński and P. Tomaszewski, Phonon properties of nanosized bismuth layered ferroelectric material-Bi2WO6, J. Raman Spectrosc., 2010, 41(9), 1059–1066 CrossRef
. - Y. Zhou, Z. Tian, Z. Zhao, Q. Liu, J. Kou, X. Chen, J. Gao, S. Yan and Z. Zou, High-yield synthesis of ultrathin and uniform Bi2WO6 square nanoplates benefitting from photocatalytic reduction of CO2 into renewable hydrocarbon fuel under visible light, ACS Appl. Mater. Interfaces, 2011, 3(9), 3594–3601 CrossRef CAS
. - S. Liu, S. Wang, C. Lei, R. Li, S. Feng and Q. Jin, Study of the efficiency of g-C3N4-loaded P25 for photocatalytic degradation of malachite green in aqueous and Pickering emulsion systems, J. Mater. Sci.: Mater. Electron., 2022, 33, 5846–5858 CrossRef CAS
. - G. Zhang, Z. Hu, M. Sun, Y. Liu, L. Liu, H. Liu, C.-P. Huang, J. Qu and J. Li, Formation of Bi2WO6 Bipyramids with Vacancy Pairs for Enhanced Solar-Driven Photoactivity, Adv. Funct. Mater., 2015, 25(24), 3726–3734 CrossRef CAS
. - J. Low, C. Jiang, B. Cheng, S. Wageh, A. A. Al-Ghamdi and J. Yu, A Review of Direct Z-Scheme Photocatalysts, Small Methods, 2017, 1(5), 1700080 CrossRef
. - Y. Zhou, Y. Zhang, M. Lin, J. Long, Z. Zhang, H. Lin, J. C.-S. Wu and X. Wang, Monolayered Bi2WO6 nanosheets mimicking heterojunction interface with open surfaces for photocatalysis, Nat. Commun., 2015, 6, 8340 CrossRef PubMed
. - H. Huang, R. Cao, S. Yu, K. Xu, W. Hao, Y. Wang, F. Dong, T. Zhang and Y. Zhang, Single-unit-cell layer established Bi2WO6 3D hierarchical architectures: Efficient adsorption, photocatalysis and dye-sensitized photoelectrochemical performance, Appl. Catal., B, 2017, 219, 526–537 CrossRef CAS
. - Y. Huang, K. Wang, T. Guo, J. Li, X. Wu and G. Zhang, Construction of 2D/2D Bi2Se3/g-C3N4 nanocomposite with High interfacial charge separation and photo-heat conversion efficiency for selective photocatalytic CO2 reduction, Appl. Catal., B, 2020, 277, 119232 CrossRef CAS
. - S. Bai, J. Jiang, Q. Zhang and Y. Xiong, Steering charge kinetics in photocatalysis: intersection of materials syntheses, characterization techniques and theoretical simulations, Chem. Soc. Rev., 2015, 44(10), 2893–2939 RSC
. - W. Yin, L. Bai, Y. Zhu, S. Zhong, L. Zhao, Z. Li and S. Bai, Embedding Metal in the Interface of a p–n Heterojunction with a Stack Design for Superior Z-Scheme Photocatalytic Hydrogen Evolution, ACS Appl. Mater. Interfaces, 2016, 8(35), 23133–23142 CrossRef CAS PubMed
. - L. Xiao, R. Lin, J. Wang, C. Cui, J. Wang and Z. Li, A novel hollow-hierarchical structured Bi2WO6 with enhanced photocatalytic activity for CO2 photoreduction, J. Colloid Interface Sci., 2018, 523, 151–158 CrossRef CAS PubMed
. - J. Sheng, Y. He, J. Li, C. Yuan, H. Huang, S. Wang, Y. Sun, Z. Wang and F. Dong, Identification of Halogen-Associated Active Sites on Bismuth-Based Perovskite Quantum Dots for Efficient and Selective CO2-to-CO Photoreduction, ACS Nano, 2020, 14(10), 13103–13114 CrossRef CAS PubMed
. - S. Wang and X. Wang, Photocatalytic CO2 reduction by CdS promoted with a zeolitic imidazolate framework, Appl. Catal., B, 2015, 162, 494–500 CrossRef CAS
. - J. Peng, J. Xu, Z. Wang, Z. Ding and S. Wang, Developing an efficient NiCo2S4 cocatalyst for improving the visible light H2 evolution performance of CdS nanoparticles, Phys. Chem. Chem. Phys., 2017, 19(38), 25919–25926 RSC
. - W. P. C. Lee, X. Y. Kong, L.-L. Tan, M. M. Gui, S. Sumathi and S.-P. Chai, Molybdenum disulfide quantum dots decorated bismuth sulfide as a superior noble-metal-free photocatalyst for hydrogen evolution through harnessing a broad solar spectrum, Appl. Catal., B, 2018, 232, 117–123 CrossRef CAS
. - S. Wang, B. Y. Guan and X. W. D. Lou, Construction of ZnIn2S4-In2O3 Hierarchical Tubular Heterostructures for Efficient CO2 Photoreduction, J. Am. Chem. Soc., 2018, 140(15), 5037–5040 CrossRef CAS PubMed
. - L. Yuan, B. Weng, J. C. Colmenares, Y. Sun and Y.-J. Xu, Multichannel Charge Transfer and Mechanistic Insight in Metal Decorated 2D-2D Bi2WO6-TiO2 Cascade with Enhanced Photocatalytic Performance, Small, 2017, 13(48), 1702253 CrossRef PubMed
. - B. Shao, Z. Liu, G. Zeng, Z. Wu, Y. Liu, M. Cheng, M. Chen, Y. Liu, W. Zhang and H. Feng, Nitrogen-Doped Hollow Mesoporous Carbon Spheres Modified g-C3N4/Bi2O3 Direct Dual Semiconductor Photocatalytic System with Enhanced Antibiotics Degradation under Visible Light, ACS Sustainable Chem. Eng., 2018, 6(12), 16424–16436 CrossRef CAS
. - M. Zhou, S. Wang, P. Yang, C. Huang and X. Wang, Boron Carbon Nitride Semiconductors Decorated with CdS Nanoparticles for Photocatalytic Reduction of CO2, ACS Catal., 2018, 8(6), 4928–4936 CrossRef CAS
. - Z. Wei, J. Liu, W. Fang, M. Xu, Z. Qin, Z. Jiang and W. Shangguan, Photocatalytic hydrogen evolution with simultaneous antibiotic wastewater degradation via the visible-light-responsive bismuth spheres-g-C3N4 nanohybrid: waste to energy insight, Chem. Eng. J., 2019, 358, 944–954 CrossRef CAS
. - J. Fu, K. Jiang, X. Qiu, J. Yu and M. Liu, Product selectivity of photocatalytic CO2 reduction reactions, Mater. Today, 2020, 32, 222–243 CrossRef CAS
. - X. Jin, J. Cao, H. Wang, C. Lv, H. Xie, F. Su, X. Li, R. Sun, S. Shi, M. Dang and L. Ye, Realizing improved CO2 photoreduction in Z-scheme Bi4O5Br2/AgBr heterostructure, Appl. Surf. Sci., 2022, 598, 153758 CrossRef CAS
. - J. Xu, Y. Chen, M. Chen, J. Wang and L. Wang, In situ growth strategy synthesis of single-atom nickel/sulfur co-doped g-C3N4 for efficient photocatalytic tetracycline degradation and CO2 reduction, Chem. Eng. J., 2022, 442, 136208 CrossRef CAS
. - X. Zhao, M. Xu, X. Song, W. Zhou, X. Liu, H. Wang and P. Huo, Integration of 3D macroscopic reduced graphene oxide aerogel with DUT-67 for selective CO2 photoreduction to CO in Gas-Solid reaction, Chem. Eng. J., 2022, 446, 137034 CrossRef CAS
.
|
This journal is © The Royal Society of Chemistry 2023 |