DOI:
10.1039/D3RA00369H
(Paper)
RSC Adv., 2023,
13, 6433-6441
Multi-responsive paper chemosensors based on mesoporous silica nanospheres for quantitative sensing of heavy metals in water†
Received
17th January 2023
, Accepted 4th February 2023
First published on 23rd February 2023
Abstract
Exposure to low concentrations of heavy metal cations seriously harms living organisms, hence they are considered environmental toxins. Portable simple detection systems are required for field monitoring of multiple metal ions. In this report, paper-based chemosensors (PBCs) were prepared by adsorbing 1-(pyridin-2-yl diazenyl) naphthalen-2-ol (chromophore), which recognizes heavy metals, onto filter papers coated with mesoporous silica nano spheres (MSNs). The high density of the chromophore probe on the surface of PBCs resulted in ultra-sensitive optical detection of heavy metal ions and short response time. The concentration of metal ions was determined using digital image-based colorimetric analysis (DICA) and compared to spectrophotometry under optimal sensing conditions. The PBCs exhibited stability and short recovery times. The detection limits determined using DICA of Cd2+, Co2+, Ni2+ and Fe3+ were 0.22, 0.28, 0.44, and 0.54 μM; respectively. Additionally, the linear ranges for monitoring Cd2+, Co2+, Ni2+ and Fe3+ were 0.44–4.4, 0.16–4.2, 0.8–8.5, and 0.002–5.2 μM; respectively. The developed chemosensors showed high stability, selectivity, and sensitivity for sensing of Cd2+, Co2+, Ni2+ and Fe3+ in water under optimum conditions and hold potential for low cost, onsite sensing of toxic metals in water.
Introduction
Heavy metal ions leak into surface or underground water from industrial activities or geological sources and pose a severe risk to living organisms.1 Cadmium is widely used in different industries including metal alloys, electroplating, stains, fertilizers, and rechargeable batteries.8 Accumulation of cadmium in tissues has been correlated to the enlargement of vital organs, bone weakening, and impairment of the cardiovascular, immunological, and reproductive systems.9 Cobalt is used as a catalyst for chemical industries and manufacturing rechargeable lithium-ion batteries.10 Although cobalt plays an important function in mammalian metabolism as a trace element; exposure to high cobalt levels has been linked to cardiomyopathy and thyroid hyperplasia.11–14 Iron is an essential component of key proteins involved in major physiological functions such as respiration and energy metabolism.2 Iron overload, however, leads to damage of vital organs such as liver, heart, and endocrine glands. Nickel is widely used in making Ni–Cd batteries and electroplating.3 Exposure to high levels of nickel has been linked to cardiovascular, kidney, and lung diseases.4–7 Multiple guidelines for acceptable levels of heavy metals in drinking water were published by different regulatory bodies including the US Environmental Protection Agency, the European Union, and the World Health Organization (Table 1).15–19 Various analytical techniques have been established for detection of heavy metal ions such as ICP-AES, ICP-MS, chemiluminescence, atomic absorption spectrometry,20,21 solid-phase22,23 and fluorescence spectroscopy.24 However, they have several drawbacks such as long turnaround time, complicated procedures, need for sophisticated infrastructure, and high cost. Therefore, the challenges for sensing different heavy metals using chromogenic materials and portable chemosensors were investigated in different assays.25–33 In this report, multi-responsive PBCs were prepared for monitoring of Cd2+, Co2+, Fe3+, and Ni2+ in water. A chromophore probe was immobilized onto mesoporous silica nanosphers (MSNs) for selective detection of various heavy metal ions.34,35 MSNs offer several advantages, including large pore volume, high surface area, and homogeneous mesopore size distribution. To generate quantitative results, color generated by the chemosensors upon detection of heavy metals was quantified by digital image colorimetric analysis (DICA) and compared to UV-vis spectroscopy (Scheme 1).36 The prepared PBCs exhibited high selectivity, stability, and sensitivity for sensing Cd2+, Co2+, Fe3+, and Ni2+ in water under optimum sensing conditions.
Table 1 Drinking water quality guidelines (μg L−1) for heavy metals
Metal |
WHO |
EU |
US EPA |
Oxidation states |
Health effects |
Cadmium |
3 |
5 |
5 |
II |
Cardiovascular issues, osteoporosis, cancer.15 |
Cobalt |
— |
— |
100 |
II, III |
Cardiovascular and pulmonary issues.16 |
Iron |
— |
200 |
300 |
II, III |
Haemochromatosis, gastrointestinal issues.17,18 |
Nickel |
70 |
20 |
— |
II |
Dermatitis, kidney failure19,20 |
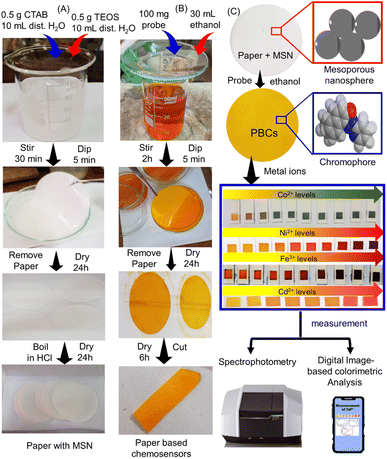 |
| Scheme 1 Fabrication of paper based chemosensors (PBCs) for sensing of Ni2+, Fe3+, Co2+ and Cd2+. (A) Mesoporous silica nanospheres (MSNs) were loaded over filter paper. (B) The papers with MSN dipped in ethanolic solution of 1-(pyridin-2-yl diazenyl) naphthalen-2-ol (organic chromophore) to prepare PBCs. (C) The PBCs generate different colours upon detection of Ni2+, Fe3+, Co2+ and Cd2+ in water under optimum conditions Spectrophotometry and digital image-based colorimetric analysis (DICA) were used for quantification of results. | |
Results and discussion
Morphology of PBCs
Field emission scanning electron microscopy (FESEM) was used to examine the morphology of the filter paper and the filter paper coated with MSNs (Fig. 1A and B). A thin layer of MSN was observed which covered the pores of the cellulose paper. Cellulose fibre cages were blocked after decoration with chromophore (Fig. 1C and D). SEM-EDS analysis and mapping of treated filter paper with MSNs, paper based chemosensors (PBCs), and PBCs + M2+ were investigated. The existing of Si element was confirmed the treatment of filter paper with MSNs (Fig. S1A†). The distribution of chromophore elements and metal ions over treated filter paper with MSNs confirms the successful immobilization and complexation respectively (Fig. S1B and C†). The X-ray diffraction patterns of paper with MSNs and PBCs were analyzed (Fig. 1E). The filter paper containing MSNs, PBCs, and PBCs after detection of metal ions revealed specific diffraction peaks ascribed to (110), (200), (110) and (004) planes. The cellulose structure was identified by the resulting XRD data.37 The diffraction intensities of the paper containing MSNs reduced significantly after chromophore decoration. Results suggest hydrogen bond interactions between the cellulose chains with MSNs and the chromophore.38 FTIR spectroscopy was conducted to investigate the decoration of chromophore into filter paper (Fig. S2†). The existence of band at 797.97, 1053.78, which are referred to siloxane bond (797.97, Si–O–Si bending (1053.78 cm−1). Our result confirms the successful grafting of MSNs. The stretching of the –OH group is responsible for the wide band between 3400 and 3200 cm−1, whereas the C–H stretching of methylene groups is responsible for the band about 2903 cm−1.
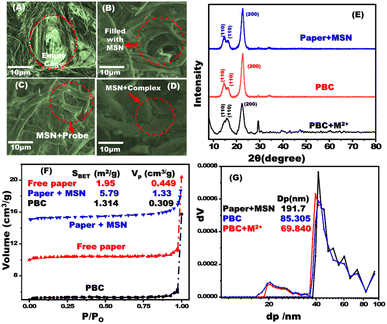 |
| Fig. 1 FE-SEM images of free filter paper (A); paper containing mesoporous silica nanospheres (MSNs) (B); MSN coated filter papers after addition of the chromophore probe (C); and interaction of MSNs/carrier/chromophore with metal ion (D). X-ray diffraction patterns of paper containing MSNs, PBCs (MSNs plus chromophore), and complex of PBC with metal ion (E). N2 isotherms (F) and NLDFT (G) of paper with MSNs, PBCs and chromophore with metal ion. | |
The N2 isotherms and NLDFT experiments were conducted to determine the pore size and surface area of the fabricated PBCs and their complexes (Fig. 1).39–41 Untreated filter paper, paper containing MSNs, optical probe, and complex showed the same isotherm type (III). The surface area of paper containing MSN was 5.79 m2 g−1 and that of untreated paper was 1.95 m2 g−1, indicating that MSNs were successfully adsorbed to the filter paper. The PBC surface area decreased dramatically (1.31 m2 g−1) when they were in combination with metal ions (0.91 m2 g−1), which suggests the efficient loading of chromophore on the treated filter paper. The NLDFT investigations revealed the presence of different pore sizes in the filter paper containing MSN (Fig. 1G). After the immobilization procedure, the pore diameter of the treated filter paper was dramatically decreased.
Determination of optimum pH for sensing metal ions
Changes in pH dramatically modify color intensity and dispersion at ultra-trace metal ion concentrations. Standard solutions of metal ions were prepared using buffer solutions at different pH values and the UV-vis absorbance was measured to identify the optimal pH level for metal ion sensing using PBCs. The determined optimal pH values for sensing Fe3+, Co2+, Ni2+and Cd2+ were 5.5, 7.0, 9.0 and 10; respectively (Fig. 2).
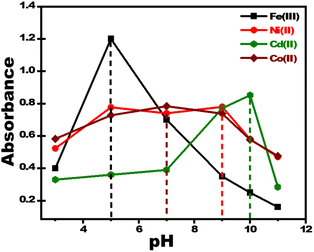 |
| Fig. 2 Effect of pH on response of paper-based chemosensors for detection of 2 ppm of Fe3+, Co2+, Ni2+, and Cd2+. The optimum pH values for sensing Fe3+, Co2+, Ni2+, and Cd2+ were 5.5, 7.0, 9.0 and 10; respectively. | |
Quantitative measurement of metal ions using PBCs and spectrophotometry
UV-vis absorption spectra were obtained for PBCs treated using increasing concentrations of Co2+, Cd2+, Ni2+, and Fe3+. Then the detection ranges (DR) of each metal ion sensing technique were determined (Fig. 3). PBCs kits offered one-step detecting methods for both qualitative and quantitative determination of Co2+, Cd2+, Ni2+, and Fe3+.
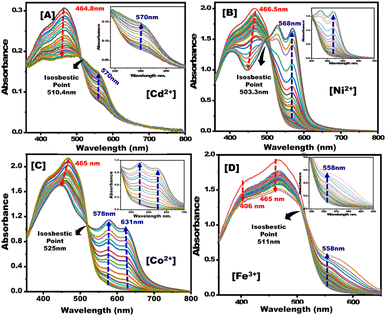 |
| Fig. 3 Absorption spectra of paper-based chemosensors upon titration with (A) Cd2+, (B) Ni2+, (C) Co2+, and (D) Fe3+ under optimum sensing parameters. | |
The calibration curves of PBCs were linear at low concentration ranges of Co2+, Cd2+, Ni2+, and Fe3+ (Fig. 4). The determined limits of detection (LD) suggest that the fabricated PBCs have recognized ultra low concentrations of target ions, as compared to sensors fabricated by conventional strategyies. PBCs based on MSNs enabled, for the first time, efficient metal ion detection down to ∼10−9 mol L−1 (Table 2). The fabricated PBCs exhibited greater recognition of Co2+, Cd2+, Ni2+, and Fe3+ compared to other chemosensors that have been described previously (Table 3).
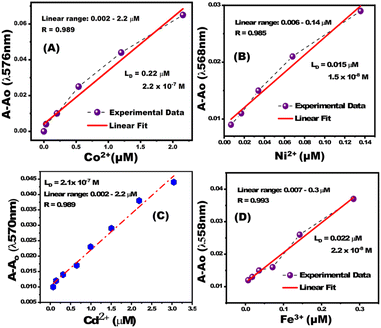 |
| Fig. 4 Calibration curves for paper-based chemosensors for (A) Co2+ at λ570nm, (B) Ni2+ at λ568nm, (C) Cd2+ at λ570nm, and (D) Fe3+ at λ558nm. | |
Table 2 Analytical parameters for detection of metal ions using paper-based chemosensors and spectrophotometric analysisa
Metal ion |
LD (μM) |
DR (μM) |
Rt (s) |
Optimum pH |
Limit of detection (LD), detection range (DR), response time (Rs). |
Co+2 |
0.22 |
0.002–2.2 |
20 |
7 |
Cd+2 |
0.13 |
0.002–8.8 |
10 |
10 |
Ni+2 |
0.015 |
0.006–0.14 |
15 |
9 |
Fe+3 |
0.022 |
0.007–0.3 |
15 |
5.5 |
Table 3 Analytical parameters for detection of metal ions using paper-based chemosensors and digital image-based colorimetric analysis
Detection principle |
Sensor |
Metal Ion |
LD (μM) |
Ref. |
Absorbance |
Cubic mesocage sensors |
Cd2+ |
0.0307 |
42 |
Absorbance |
Cd2+ chemosensors |
Cd2+ |
0.19 |
43 |
Absorbance |
Dithizone TiO2 sensor |
Cd2+ |
0.0156 |
44 |
Absorbance |
Aluminosilica optical sensor |
Cd2+ |
0.0024 |
45 |
Co2+ |
0.0028 |
Absorbance |
Green AuNP probe |
Cd2+ |
0.03 |
46 |
Absorbance |
Functionalized AuNP |
Cd2+ |
0.0629 |
47 |
Absorbance |
Azo-HNTA probe |
Co2+ |
0.77 |
48 |
Absorbance |
CpAD probe |
Co2+ |
0.0066 |
49 |
Absorbance |
Chemosensor for cobalt |
Co2+ |
1.8 |
50 |
Absorbance |
Chemosensor (HL) based on coumarin |
Co2+ |
0.31 |
51 |
Fluorescence |
Eu(III)-organic framework |
Fe3+ |
23 |
52 |
Fluorescence |
Uranyl organic framework |
Fe3+ |
0.0992 |
53 |
Fluorescence |
Eu3+post-functionalized UiO-66 |
Fe3+ |
12.8 |
54 |
Colorimetric |
Multicomponent sensor |
Fe3+ |
1.26 |
55 |
Fluorescence |
Aryl hydrazones of β-diketones |
Ni2+ |
7 |
56 |
Absorbance |
Alizarin complexone |
Ni2+ |
40 |
57 |
Fluorescence |
Hydrazine carbothioamide |
Ni2+ |
0.079 |
58 |
Absorbance |
Quinoline derivative |
Ni2+ |
0.22 |
59 |
Absorbance |
Chalcone based ratiometric chemosensor |
Ni2+ |
5.14 |
60 |
Absorbance |
Paper based chemosensors (PBCs) using spectrophotometry |
Cd2+ |
0.13 |
This work |
Co2+ |
0.22 |
Ni2+ |
0.015 |
Fe3+ |
0.022 |
Absorbance |
Paper based chemosensors (PBCs) using digital image-based colorimetric analysis (DICA) |
Cd2+ |
0.22 |
This work |
Co2+ |
0.28 |
Ni2+ |
0.44 |
Fe3+ |
0.54 |
Quantitative determination of metal ions using PBCs and digital image-based colorimetric analysis (DICA)
The change in RGB intensity values (IR, IG, and IB) of PBCs were examined using the images of PBC colors (obtained using cell phone camera) which developed upon sensing different concentrations of Co2+, Cd2+, Ni2+, and Fe3+ in water. The obtained images of PBCs kits were analysed using Adobe Photoshop CS6. Scheme 1C shows the digital images of PBCs with different concentrations of Co2+, Cd2+, Ni2+, and Fe3+. The mean integer value for each RGB component decreased when an intense color was created as the metal ion concentration increased (Table 4). As shown in Fig. 5, the relationships between color absorbance and the concentrations of Co2+, Cd2+, Ni2+, and Fe3+ agreed with those obtained by spectrophotometry (Fig. 3). The Co2+, Cd2+, Ni2+, and Fe3+ concentrations and the absorbance of the mean integer value for each RGB component were shown to be linearly correlated, demonstrating that these ions may be detected with great sensitivity at extremely low concentrations (Fig. 6). The LD and LQ of metal ions using DICA are in agreement with those obtained by spectrophotometry. Therefore, DICA can be utilized as a low cost, portable, and semi-quantitative analytical method for sensing Co2+, Cd2+, Ni2+, and Fe3+ recognized by the developed PBCs.
Table 4 Recognition of metal ions using PBCs and digital image-based colorimetric analysis
Metal ion |
LD (mol L−1) |
DR (mol L−1) |
Rt (s) |
Specific pH |
Co(II) |
2.8 × 10−7 (16.5 ppb) |
(0.16–4.2) × 10−6 |
20 |
7 |
Cd(II) |
3.1 × 10−7 (34.85 ppb) |
(0.44–4.4) × 10−6 |
10 |
10 |
Ni(II) |
4.4 × 10−7 (25.824 ppb) |
(0.8–8.5) × 10−6 |
20 |
9 |
Fe(II) |
5.4 × 10−7 (30.15 ppb) |
(0.002–5.2) × 10−6 |
20 |
5 |
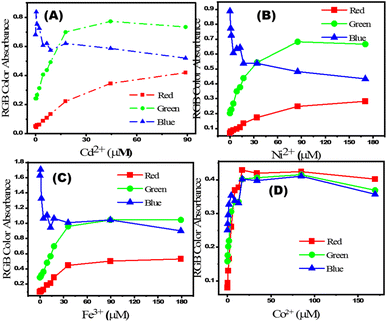 |
| Fig. 5 Relationship between (A) [Cd2+], (B) [Ni2+], (C) [Fe3+] and (D) [Co2+] and their calculated absorbances from RGB of images captured using a mobile camera. | |
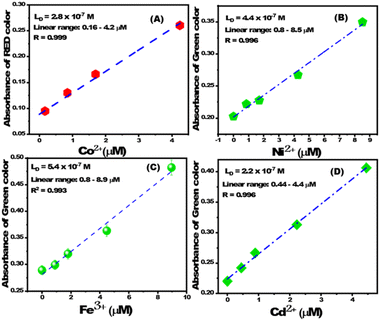 |
| Fig. 6 The linear correlation between absorbance of (A) red color and [Co2+], (B) green color and [Ni2+], (C) green color and [Fe3+], and (D) green color and [Cd2+]. | |
Selectivity analyses
The selectivity of PBCs towards Cd2+, Co2+, Ni2+, and Fe3+ was investigated under optimal sensing conditions. Known concentrations of possible interferring cations were added to 0.5 ppm of Co2+, Cd2+, Ni2+, and Fe3+ and solutions tested using the PBCs at the specific ion sensing pH values of 5.5, 7, 9, and 10 for Fe3+, Co2+, Ni2 and Cd2+; respectively. For each target ion, and in the presence of interfering ions, there were no significant variations in the PBC absorption spectra or visual color patterns as detection of metal ions was carried out at their specific pH values (Fig. 7). Table S1† illustrates the tolerance concentrations of several interfering ions.
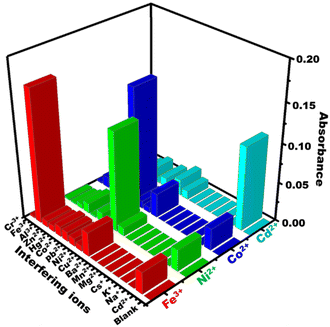 |
| Fig. 7 Effect of common interfering cations on absorbance spectra of paper-based chemosensors under optimum sensing conditions (Co2+ at λ570nm, Ni2+ at λ568nm, Cd2+ at λ570nm, and Fe3+ at λ558nm). | |
Proposed sensing mechanism of PBCs
Under optimum sensing conditions, commercial filter papers treated with MSNs then covered with the optical probe were employed to detect Co2+, Cd2+, Ni2+, and Fe3+ (Scheme 2). When oxygen and azo-nitrogen of organic probe on PBCs are available for complexation with Co2+, Cd2+, Ni2+, or Fe3+, stable complex with two coordination spheres are formed. As indicated in Scheme 2, the stoichiometric ratio of Co2+, Cd2+, Ni2+, and Fe3+ to organic probe at a specific pH is predicted to be 1
:
2. The results show that raising Co2+, Cd2+, Ni2+, and Fe3+ concentrations improved absorption spectra (Fig. 3). Furthermore, complex formation and charge transfer were linked to the occurrence of an isosbestic point. The developed PBCs enable naked-eye detection of ultra-trace metal ion concentrations without the need for complicated traditional techniques.
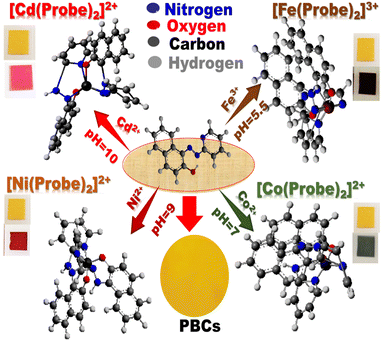 |
| Scheme 2 . Schematic diagram of the possible interactions of the paper-based chemosensors (PBCs) with Cd2+, Co2+, Fe3+ and Ni2+ under optimal sensing conditions. Upon the formation of different complexes between the optical probe and the heteroatom, the color of PBCs changes: (a) from yellow to pink in presence of Cd2+ at pH = 10; from yellow to dark green in presence of Co2+ at pH = 7, (c) from yellow to red in presence of Ni2+ at pH = 9, and (d) from yellow to dark brown in presence of Fe3+ at pH = 5.5. | |
Using PBCs for detection of metal ions in water
PBCs were used to test several drinking water samples to identify Co2+, Cd2+, Ni2+, and Fe3+. Water samples were collected from a variety of places and spiked with known concentrations of Co2+, Cd2+, Ni2+, and Fe3. Under optimum sensing conditions, PBCs were dipped into the water samples and different colors developed immediately in presence of different metal ions (Table S2†). Water samples were tested three times for Co2+, Cd2+, Ni2+, and Fe3+, then the results were quantified using DICA. Results obtained from DICA analysis were concordant to those obtained by spectrophotometry (Table S2†). Elution studies were carried out using various concentrations of EDTA to determine the best eluent for adsorbent renewal and reusability (Fig. S3†). The designed optical chemosensors for sensing Fe(III), Co(II), Ni(II), and Cd(II) ions can be used multiple times.
To the best of our knowledge, this study is the first to develop multi-responsive optical chemosensors based on mesoporous silica that incorporates simplicity but still retains sensitivity, selectivity, and fast-response detection of target ions in drinking and environmental water samples. Coating the filter papers with mesoporous silica nanospheres as carriers of the probe improved the sensing functionality for detection of ultra-trace concentrations of metal ions in water samples. The optical chemosensors have uniform structural morphology enabled by utilizing mesoporous silica nanospheres as carriers for sensing the ultra-trace concentration of metal ions in water resources. The detection limit of sensing ultra-trace metal ions is 100 times lower than other reported method. The developed optical chemosensors can be regenerated, using 0.1 M EDTA solution, and reused multiple times. Superior selectivity of optical chemosensors for their target cations under optimal sensing conditions (pH, etc) even in the presence of multiple interfering cations. Colorimetric techniques can be used to measure color intensities of the chemosensors and generate quantitative results. The fabricated optical chemosensors exhibited high stability (in water and on shelf).
Clearly, this study supports several of the United Nations Sustainability Development Goals including good health and well-being, clean water and sanitation, and industry and innovation. The designed paper based chemosensors (kits) are of low cost compared to similar commercial ones which would enable large scale accessibility by populations in low- and middle-income countries. It is also of note that the produced chemosensors can be regenerated and used multiple times. Additionally, similar chemosensors can be optimized for detection of toxic metals in cosmetics. Finally, future studies can investigate the use of the developed strategy for removal of heavy metals from water.
Experimental
Chemicals
All experiments were conducted using Milli-Q water. CoCl2, NiCl2, FeCl3, CdCl2, diethyl ether, CH3COONa, CH3COOH, 1-(pyridin-2-yl diazenyl) naphthalen-2-ol (C15H11N3O), tetraethyl orthosilicate (TEOS), acetone, and cetyltrimethyl ammonium bromide (CTAB), were obtained from Sigma-Aldrich (St. Louis, MO). NaOH, and Na2HPO4 were acquired from El-Nasr Pharmaceuticals (Cairo, Egypt).
Fabrication of PBCs
MSNs were prepared at pH = 7 and applied on filter papers as described previously with minor modifications.28 CTAB (0.5 g) was mixed with 100 mL of distilled water for 30 min. Ethanol (10 mL) and TEOS (2.5 mL) were then added and the solution mixed for another 0.5 h (Scheme 1A). Then, 1.5 mL of NaOH was added and the solution stirred for 2 h. The filter papers were immersed in the prepared MSN solution multiple times (5 min each). The papers containing MSN were dried at 50 °C for 6 h then dipped in ethanolic solution (30 mL) of 1-(pyridin-2-yl diazenyl) naphthalen-2-ol (100 mg) for multiple times (5 min each) as shown in (Scheme 1B). To produce the PBCs which were then cut into appropriate size (1 cm2) and placed into 3D printed holders (Scheme 1C).
Analysis of toxic metal ions
Metal ion solutions (200 ppm) were prepared in Milli-Q water. The PBC was placed in a quartz cuvette then a known concentration of a specific metal ion was added and sonicated for 5 s. UV-vis spectroscopic spectra were measured within seconds (without shaking). Colorimetric detection of different concentrations of Cd2+, Co2+, Fe3+, and Ni2+ using PBCs were performed at different pH. UV-vis spectra were obtained and after equilibration, a prominent color change was observed and the PBC absorbance spectra obtained. The color change of PBCs was also quantified using DICA.
Conclusions
PBCs were fabricated by adsorbing mesoporous silica nanosphere carrier, prepared using a low-cost method, on filter papers then decorated with the optical chromophore 1-(pyridin-2-yl diazenyl) naphthalen-2-ol. Optimal sensing conditions for each PBC were determined. The MSN-based PBCs enabled visual detection of Co2+, Cd2+, Ni2+, and Fe3+ present in water with high sensitivity and selectivity. The PBCs allowed detection of multiple ions and featured long-term stability and could be utilized several times using a simple regeneration process. Quantitative results were obtained by analysis of images (obtained using cell phone camera) of colored PBCs using DICA which were similar to those obtained with spectrophotometry. This possibly is the first report that employs PBCs based on MSNs for detecting multiple heavy metal ions in water using DICA or UV-vis spectroscopy. Additional PBCs could be developed using similar strategies and optimized for detection of additional metals in water and other matrices.
Author contributions
Conceptualization, I. M. E, H. M. E. A.; methodology and data collection, A. R. and I. M. E.; writing, reviewing and editing, all authors; project administration and funding, H. M. E. A. All authors have written and reviewed the final version of the manuscript.
Conflicts of interest
HMEA is an inventor on a granted patent on developing optical chemosensors for detection of toxic metals.
Acknowledgements
This work has been funded by an award from the French Chamber of Commerce in Cairo to H. M. E. A.
Notes and references
- M. A. Zoroddu, J. Aaseth, G. Crisponi, S. Medici, M. Peana and V. M. Nurchi, The essential metals for humans: a brief overview, J. Inorg. Biochem., 2019, 195, 120–129 CrossRef CAS PubMed
. - N. Abbaspour, R. Hurrell and R. Kelishadi, Review on iron and its importance for human health, J. Res. Med. Sci., 2014, 19(2), 164 Search PubMed
. - F. A. Abebe, C. S. Eribal, G. Ramakrishna and E. Sinn, A ‘turn-on’fluorescent sensor for the selective detection of cobalt and nickel ions in aqueous media, Tetrahedron Lett., 2011, 52(43), 5554–5558 CrossRef CAS
. - R. Kozyraki and O. Cases, Vitamin B12 absorption: Mammalian physiology and acquired and inherited disorders, Biochimie, 2013, 95(5), 1002–1007 CrossRef CAS PubMed
. - G. WHO, Guidelines for drinking-water quality, World Health Organization, vol. 216, 2011, pp. 303–304 Search PubMed
. - S. W. Ragsdale, Nickel and its surprising impact in nature. Metal ions in life sciences, Vol. 2. Edited by Astrid Sigel, Helmut Sigel, and Roland K. O. Sigel, Angew. Chem., Int. Ed., 2008, 47, 824–826 CrossRef CAS
. - K. Kasprzak, Nickel carcinogenesis, Mutat. Res., Fundam. Mol. Mech. Mutagen., 2003, 533(1–2), 67–97 CrossRef CAS PubMed
. - L. Trnkova, V. Adam and R. Kizek, The effect of cadmium ions and cadmium nanoparticles on chicken embryos and evaluation of organ accumulation, Int. J. Electrochem. Sci., 2015, 10, 3623–3634 Search PubMed
. - H. N. Kim, W. X. Ren, J. S. Kim and J. Yoon, Fluorescent and colorimetric sensors for detection of lead, cadmium, and mercury ions, Chem. Soc. Rev., 2012, 41(8), 3210–3244 RSC
. - H. Y. Au-Yeung, E. J. New and C. J. Chang, A selective reaction-based fluorescent probe for detecting cobalt in living cells, Chem. Commun., 2012, 48(43), 5268 RSC
. - A. I. Seldén, C. Norberg, C. Karlson-Stiber and E. Hellström-Lindberg, Cobalt release from glazed earthenware: Observations in a case of lead poisoning, Environ. Toxicol. Pharmacol., 2007, 23(1), 129–131 CrossRef PubMed
. - H. Tavallali, G. Deilamy-Rad, A. Parhami and S. Z. Mousavi, A novel development of dithizone as a dual-analyte colorimetric chemosensor: detection and determination of cyanide and cobalt (II) ions in dimethyl sulfoxide/water media with biological applications, J. Photochem. Photobiol., B, 2013, 125, 121–130 CrossRef CAS PubMed
. - K. Ngamdee, T. Tuntulani and W. Ngeontae, l-Cysteine modified luminescence nanomaterials as fluorescence sensor for Co2+: Effects of core nanomaterials in detection selectivity, Sens. Actuators, B, 2015, 216, 150–158 CrossRef CAS
. - D. Maity, V. Kumar and T. Govindaraju, Reactive Probes for Ratiometric Detection of Co2+ and Cu+ Based on Excited-State Intramolecular Proton Transfer Mechanism, Org. Lett., 2012, 14(23), 6008–6011 CrossRef CAS PubMed
. - M. R. Rahimzadeh, M. R. Rahimzadeh, S. Kazemi and A. A. Moghadamnia, Cadmium toxicity and treatment: An update, Caspian J. Intern. Med., 2017, 8, 135 Search PubMed
. - S. Agrawal, K. L. Berggren, E. Marks and J. H. Fox, Impact of high iron intake on cognition and neurodegeneration in humans and in animal models: A systematic review, Nutr. Rev., 2017, 75, 456–470 CrossRef PubMed
. - N. S. Rao, Iron content in groundwaters of Visakhapatnam environs, Andhra Pradesh, India, Environ. Monit. Assess., 2008, 136, 437–447 CAS
. - H. Kitaura, N. Nakao, N. Yoshida and T. Yamada, Induced sensitization to nickel in guinea pigs immunized with mycobacteria by injection of purified protein derivative with nickel, New Microbiol., 2003, 26, 101–108 CAS
. - A. Cavani, Breaking tolerance to nickel, Toxicology, 2005, 209, 119–121 CrossRef CAS PubMed
. - M. M. Junior, L. O. Silva, D. J. Leão and S. L. Ferreira, Analytical strategies for determination of cadmium in Brazilian vinegar samples using ET AAS, Food Chem., 2014, 160, 209–213 CrossRef CAS PubMed
. - W. C. Chaiyasith, A. Thongsaw, G.
M. Ross and R. Sananmuang, Solidified floating organic drop microextraction for the determination of cadmium in water samples by electrothermal atomic absorption spectrometry, NU. Int. J. Sci., 2016, 13(1), 1–7 Search PubMed
. - H. R. Fouladian and M. Behbahani, Solid phase extraction of Pb (II) and Cd (II) in food, soil, and water samples based on 1-(2-pyridylazo)-2-naphthol-functionalized organic–inorganic mesoporous material with the aid of experimental design methodology, Food Anal. Methods, 2015, 8(4), 982–993 CrossRef
. - Y. Liu, X. Chang, S. Wang, Y. Guo, B. Din and S. Meng, Solid-phase extraction and preconcentration of cadmium (II) in aqueous solution with Cd(II)-imprinted resin (poly-Cd (II)-DAAB-VP) packed columns, Anal. Chim. Acta, 2004, 519(2), 173–179 CrossRef CAS
. - A. Filipiak-Szok, M. Kurzawa and E. Szłyk, Determination of toxic metals by ICP-MS in Asiatic and European medicinal plants and dietary supplements, J. Trace Elem. Med. Biol., 2015, 30, 54–58 CrossRef CAS PubMed
. - C. Zhuo, Z. Zhang, J. Qi, J. You, J. Ma and L. Chen, Colorimetric detection of heavy metal ions with various chromogenic materials: Strategies and applications, J. Hazard. Mater., 2022, 129889 Search PubMed
. - C. Yu, R. Wang, B. Brady and X. Wang, Fully inkjet-printed paper-based Pb2+ optodes for water analysis without interference from the chloramine disinfectant, Anal. Bioanal. Chem., 2022, 414(26), 7585–7595 CrossRef PubMed
. - F. Xiuli, H. Lin, J. Qi, F. Li, Y. Chen, B. Li and L. Chen, A tetrahedral DNA nanostructure functionalized paper-based platform for ultrasensitive colorimetric mercury detection, Sens. Actuators, B, 2022, 362, 131830 CrossRef
. - S. Hoda, J. Tashkhourian and B. Hemmateenejad, Identification and determination of multiple heavy metal ions using a miniaturized paper-based optical device, Sens. Actuators, B, 2022, 359, 131551 CrossRef
. - J. Lim, K. Tai-Yong and W. Min-Ah, Trends in sensor development toward next-generation point-of-care testing for mercury, Biosens. Bioelectron., 2021, 183, 113228 CrossRef CAS PubMed
. - H. A. Silva-Neto, T. M. Cardoso, C. J. McMahon, L. F. Sgobbi, C. S. Henry and W. K. Coltro, Plug-and-play assembly of paper-based colorimetric and electrochemical devices for multiplexed detection of metals, Analyst, 2021, 146(11), 3463–3473 RSC
. - X. Sun, L. Bowei, Q. Anjin, T. Chongguo, H. Jinglong, S. Yajun, L. Bingcheng and C. Lingxin, Improved assessment of accuracy and performance using a rotational paper-based device for multiplexed detection of heavy metals, Talanta, 2018, 178, 426–431 CrossRef CAS PubMed
. - J. Fu, W. Xiaoyan, L. Jinhua, D. Yangjun and C. Lingxin, Synthesis of multi-ion imprinted polymers based on dithizone chelation for simultaneous removal of Hg2+, Cd2+, Ni2+ and Cu2+ from aqueous solutions, RSC Adv., 2016, 6(50), 44087–44095 RSC
. - B. Li, L. Fu, W. Zhang, W. Feng and L. Chen, Portable paper-based device for quantitative colorimetric assays relying on light reflectance principle, Electrophoresis, 2014, 35(8), 1152–1159 CrossRef CAS PubMed
. - I. M. El-Sewify, A. Radwan, A. Shahat, M. F. El-Shahat and M. M. Khalil, Superior adsorption and removal of aquaculture and bio-staining dye from industrial wastewater using microporous nanocubic Zn-MOFs, Microporous Mesoporous Mater., 2022, 329, 111506 CrossRef CAS
. - M. M. Khalil, A. Shahat, A. Radwan and M. F. El-Shahat, Colorimetric determination of Cu(II) ions in biological samples using metal-organic framework as scaffold, Sens. Actuators, B, 2016, 233, 272–280 CrossRef CAS
. - A. Radwan, I. M. El-Sewify, A. Shahat, M. F. El-Shahat and M. M. Khalil, Decorated nanosphere mesoporous silica chemosensors for rapid screening and removal of toxic cadmium ions in well water samples, Microchem. J., 2020, 156, 104806 CrossRef CAS
. - A. Radwan, I. M. El-Sewify and H. M. E. S. Azzazy, Monitoring of Cobalt and Cadmium in Daily Cosmetics Using Powder and Paper Optical Chemosensors, ACS Omega, 2022, 7(18), 15739–15750 CrossRef CAS PubMed
. - J. Gong, J. Li, J. Xu, Z. Xiang and L. Mo, Research on cellulose nanocrystals produced from cellulose sources with various polymorphs, RSC Adv., 2017, 7(53), 33486–33493 RSC
. - I. Kilpeläinen, H. Xie, A. King, M. Granstrom, S. Heikkinen and D. S. Argyropoulos, Dissolution of Wood in Ionic Liquids, J. Agric. Food Chem., 2007, 55(22), 9142–9148 CrossRef PubMed
. - A. M. Azzam, M. A. Shenashen, M. M. Selim, H. Yamaguchi, I. M. El-Sewify, S. Kawada, A. A. Alhamid and S. A. El-Safty, Nanospherical inorganic α-Fe core-organic shell necklaces for the removal of arsenic(V) and chromium(VI) from aqueous solution, J. Phys. Chem. Solids, 2017, 109, 78–88 CrossRef CAS
. - A. E Soliman, M. A. Shenashen, I. M. El-Sewify, G. M. Taha, M. A. El-Taher, H. Yamaguchi, A. S. Alamoudi, M. M Selim and S. A. El-Safty, Mesoporous Organic-Inorganic Core-Shell Necklace Cages for Potentially Capturing Cd2+ Ions from Water Sources, ChemistrySelect, 2017, 2(21), 6135–6142 CrossRef
. - S. A. El-Safty, M. A. Shenashen and M. Khairy, Optical detection/collection of toxic Cd(II) ions using cubic Ia3d aluminosilica mesocage sensors, Talanta, 2012, 98, 69–78 CrossRef CAS PubMed
. - V. Tekuri and D. R. Trivedi, A new colorimetric chemosensors for Cu2+ and Cd2+ ions detection: Application in environmental water samples and analytical method validation, Anal. Chim. Acta, 2017, 972, 81–93 CrossRef CAS PubMed
. - A. Shahat, E. A. Ali and M. F. El Shahat, Colorimetric determination of some toxic metal ions in post-mortem biological samples, Sens. Actuators, B, 2015, 221, 1027–1034 CrossRef CAS
. - M. A. Shenashen, S. A. El-Safty and E. A. Elshehy, Monolithic scaffolds for highly selective ion sensing/removal of Co(ii), Cu(ii), and Cd(ii) ions in water, Analyst, 2014, 139(24), 6393–6405 RSC
. - R. Manjumeena, D. Duraibabu, T. Rajamuthuramalingam, R. Venkatesan and P. T. Kalaichelvan, Highly responsive glutathione functionalized green AuNP probe for precise colorimetric detection of Cd2+ contamination in the environment, RSC Adv., 2015, 5(85), 69124–69133 RSC
. - V. N. Mehta, H. Basu, R. K. Singhal and S. K. Kailasa, Simple and sensitive colorimetric sensing of Cd2+ ion using chitosan dithiocarbamate functionalized gold nanoparticles as a probe, Sens. Actuators, B, 2015, 220, 850–858 CrossRef CAS
. - K. S. Abou-Melha, G. A. A. Al-Hazmi, T. M. Habeebullah, I. Althagafi, A. Othman, N. M. El-Metwaly, F. Shaaban and A. Shahat, Functionalized silica nanotubes with azo-chromophore for enhanced Pd2+ and Co2+ ions monitoring in E-wastes, J. Mol. Liq., 2021, 329, 115585 CrossRef CAS
. - M. R. Awual, M. M. Hasan, A. Islam, A. M. Asiri and M. M. Rahman, Optimization of an innovative composited material for effective monitoring and removal of cobalt(II) from wastewater, J. Mol. Liq., 2020, 298, 112035 CrossRef CAS
. - Y. J. Na, Y. W. Choi, G. R. You and C. Kim, A novel selective colorimetric chemosensor for cobalt ions in a near perfect aqueous solution, Sens. Actuators, B, 2016, 223, 234–240 CrossRef CAS
. - Z. Liu, W. Wang, H. Xu, L. Sheng, S. Chen, D. Huang and F. A. Sun, Naked eye” and ratiometric chemosensor for cobalt(II) based on coumarin platform in aqueous solution, Inorg. Chem. Commun., 2015, 62, 19–23 CrossRef CAS
. - Q. Chang, K. Du, L. Q. Chen, N. N. Xu, F. C. Wang, W. Zhang and X. D. Ding, A fluorescent channel-type Eu(III)-organic framework for selective detection of Fe3+ ion and protective effect against Parkinson disease by increasing mitochondrial complex activity, J. Mol. Struct., 2020, 1203, 127439 CrossRef CAS
. - W. Liu, J. Xie, L. Zhang, M. A. Silver and S. Wang, A hydrolytically stable uranyl organic framework for highly sensitive and selective detection of Fe3+ in aqueous media, Dalton Trans., 2018, 47(3), 649–653 RSC
. - L. Li, S. Shen, W. Ai, S. Song, Y. Bai and H. Liu, Facilely synthesized Eu3+ post-functionalized UiO-66-type metal-organic framework for rapid and highly selective detection of Fe3+ in aqueous solution, Sens. Actuators, B, 2018, 267, 542–548 CrossRef CAS
. - H. Wang, X. Wang, R. M. Kong, L. Xia and F. Qu, Metal-organic framework as a multi-component sensor for detection of Fe3+, ascorbic acid and acid phosphatase, Chin. Chem. Lett., 2021, 32(1), 198–202 CrossRef CAS
. - A. Subhasri, S. Balachandran, K. Mohanraj, P. S. Kumar, K. J. Jothi and C. Anbuselvan, Synthesis, Computational and cytotoxicity studies of aryl hydrazones of β-diketones: Selective Ni2+ metal Responsive fluorescent chemosensors, Chemosphere, 2022, 297, 134150 CrossRef CAS PubMed
. - K. I. S. Aikawa and Y. Fukushima, Colorimetric chemosensor for Ni2+ based on alizarin complexone and a cationic polyelectrolyte in aqueous solution, J. Appl. Polym. Sci., 2019, 136(19), 47496 CrossRef
. - A. Subhasri, S. Balachandran, K. Mohanraj, P. S. Kumar, K. J. Jothi and C. Anbuselvan, Synthesis, Computational and cytotoxicity studies of aryl hydrazones of β-diketones: Selective Ni2+ metal Responsive fluorescent chemosensors, Chemosphere, 2022, 297, 134150 CrossRef CAS PubMed
. - X. Liu, Q. Lin, T. B. Wei and Y. M. Zhang, A highly selective colorimetric chemosensor for detection of nickel ions in aqueous solution, New J. Chem., 2014, 38(4), 1418–1423 RSC
. - J. Prabhu, K. Velmurugan, A. Raman, N. Duraipandy, M. S. Kiran, S. Easwaramoorthi and R. Nandhakumar, A simple chalcone based ratiometric chemosensor for sensitive and selective detection of Nickel ion and its imaging in live cells, Sens. Actuators, B, 2017, 238, 306–317 CrossRef CAS
.
|
This journal is © The Royal Society of Chemistry 2023 |