DOI:
10.1039/D3RA00234A
(Paper)
RSC Adv., 2023,
13, 6779-6792
Efficient photo-Fenton catalysis using magnetic iron nanoparticles decorated boron nitride quantum dots: theoretical and experimental investigations†
Received
12th January 2023
, Accepted 13th February 2023
First published on 27th February 2023
Abstract
To achieve the efficient removal of pharmaceutical wastes, novel photo-Fenton catalysts, iron-decorated boron nitride quantum dots (Fe@BNQDs) were prepared. Fe@BNQDs were characterized using XRD, SEM-EDX, FTIR, and UV-Vis spectrophotometry. The decoration of Fe on the surface of BNQDs enhanced the catalytic efficiency due to the photo-Fenton process. Photo-Fenton catalytic degradation of folic acid was investigated under UV and visible light. The influence of H2O2, catalyst dose, and temperature on the degradation yield of folic acid was investigated using Response Surface Methodology. Moreover, the efficiency of the photocatalysts and kinetics was investigated. Radical trapping experiments revealed that holes were the main dominant species in the photo-Fenton degradation mechanism and BNQDs played active roles because of their hole extraction ability. Additionally, active species such as e− and O2−˙ have a medium effect. The computational simulation was utilized to provide insights into this fundamental process, and for this purpose, electronic and optical properties were calculated.
1. Introduction
The worldwide industrial revolution in the 21st century brought a wide spectrum of problems, mainly the contamination of water with harmful and waste materials, leading to significant adverse effects on the environment and wildlife.1 Water is the common solvent that is available in enough quantity for industrial operations. In most pharmaceutical manufacturing, water is in contact with drugs either as a solvent, product, and/or for cleaning purposes.2 The contaminated water comes into contact with a river stream or underground water or remains on the surface and then evaporates, leaving chemicals including drugs that bind to sand particles, which again due to erosion by rainwater re-contact with the water body.3,4
In recent decades, the removal of harmful chemicals is the research interest in academia and industry. Efforts are being made to develop novel new materials and technologies to remove contaminants, including physical, chemical, and biological wastewater treatments.2
Regarding photocatalytic mineralization, most researchers try to achieve a higher rate and more efficient photocatalysts. Heterogeneous photocatalysis uses semiconductor oxides irradiated with UV, near-UV, or visible light at ambient temperature and pressure and in the presence of oxygen.1,5–8 Nano-sized photocatalysts, specifically the zero-dimensional (0D) nanoparticles, exhibit enhanced photocatalysis efficiency compared to their bulk equivalents. This is caused by the presence of more active sites on the surface, enhanced electron–hole separation, a lower recombination rate, and broad absorption in the visible spectrum, which makes the photocatalyst harvest more energy.9,10
Advanced oxidation processes (AOPs) are a group of methods that can produce oxidants with the use of catalysts, potent oxidants, light, or thermal input. They are promising and strong methods for degrading many organic compounds because they produce strong oxidizing agents.2,3
The Fenton process in AOPs is regarded as one of the most efficient methods for degrading a wide variety of organic compounds at ambient temperature.4,11–14 The Fenton process involves the reaction between H2O2 and ferrous ions in an acidic aqueous solution, leading to the production of hydroxyl radicals (˙OH).13 Although the reagents used in the Fenton process are relatively safe to handle and easy to store, however, there are some disadvantages of the Fenton reaction, such as the limited pH range (acidic pHs) and the difficulty of regeneration of the catalyst. Ferrous ions must be added to the reaction medium continuously to sustain the reaction.
To overcome the limitation of the Fenton process, light irradiation was introduced into the Fenton system to form the so-called photo-Fenton process. During the photo-Fenton process, only small amounts of H2O2 and iron salt are required.4,11–14 However, more ˙OH is produced than by the Fenton process or non-Fenton photo processes,13 which accelerate the degradation rates of a variety of pollutants.
Heterogeneous photocatalysis is a good candidate to harvest light and trigger the Fenton reaction within wider pH ranges.11,15 Numerous heterogeneous photocatalysts, including metallic nanoparticles,16–18 metal oxides,8,19,20 CdS21–23 and carbon-based nanomaterials24,25 have been developed.
Boron nitride quantum dots (BNQDs) are a class of highly fluorescent quantum dots (QDs).26–28 It has a wide band gap of 4.5 eV to 6.0 eV, which is not suitable for visible light applications.29–31 To be utilized for solar-light applications, a narrowing of the band gap is attempted. Angizi et al. studied that functionalized boron nitride has an Eg below 4.5 eV, and this might depend on the functional groups of BNQDs. Hydroxyl-functionalized BNQDs gave Eg 2.3–3.6 eV, methyl functionalized was 3.2–4.2 eV, and amine-functionalized was 3.1–4.0 eV. Thus, the functionalization of BNQDs make them a potential tool in photocatalysis research.32
In the present work, nanostructured Fe@BNQDs were prepared and used to remove folic acid via photo-Fenton degradation. A heterogeneous photo-Fenton-like process was applied for this purpose due to the addition of H2O2 and the presence of Fe nanoparticles on BNQDs. Trapping experiments of the reactive species (ROS) were performed to find the active species responsible for the degradation of folic acid. Fig. 1 shows a scheme for the preparation and application of Fe@BNQDs.
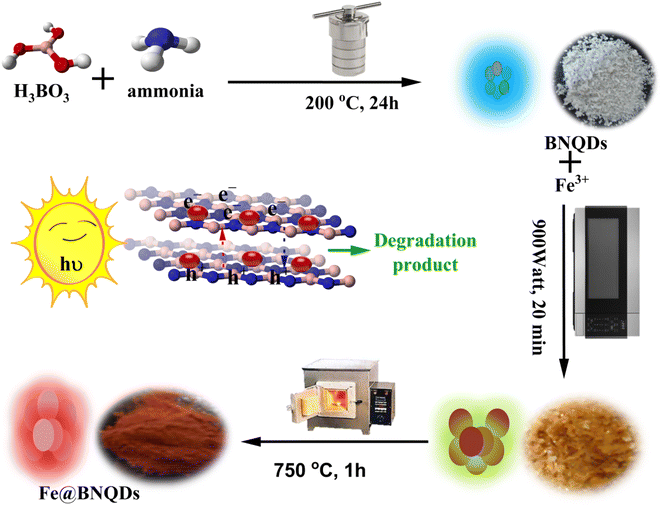 |
| Fig. 1 A schematic diagram demonstrates the preparation and application of Fe@BNQDs. | |
2. Experimental section
2.1. Instrumentation
The surface morphological, chemical composition, crystal quality, and structural properties of the synthesized Fe@BNQDs were characterized and studied utilizing field-emission scanning electron microscopy (FE-SEM) (SEM 4500-Quanta FEI, USA); the chemical composition of the synthesized nanostructures was measured by energy-dispersive X-ray spectroscopy (EDX) performed in FE-SEM, X-ray powder diffraction (XRD) was used to measure crystal structure using X'Pert PRO (PANalytical, Netherlands) using Kα for copper element (Cu Kα = 1.5406 Å at 40 kV, 30 mA) in the 2θ range of (20° to 70°) and the rate of scanning was 1° min−1, respectively. FTIR spectra were recorded using (Thermo Scientific, USA). For monitoring the photodegradation process, absorbance spectra were recorded on a Lambda 25 PerkinElmer UV-Vis spectrophotometer (PerkinElmer, USA).
2.2. Chemicals
All chemicals used were of analytical grade and used as purchased without further treatment. Boric acid, iron(III) nitride, sodium oxalate, potassium bromate, and hydrogen peroxide were obtained from CARL ROTH GmbH (Karlsruhe, Germany). Ammonia at 25% was purchased from Merck (Darmstadt, Germany). Isopropyl alcohol was purchased from Fluka AG in Sigma Aldrich (Steinheim, Germany). Ascorbic acid was purchased from Scharlau Chemicals (Barcelona, Spain) and folic acid was purchased from UNI-CHEM (China) chemicals.
2.3. Synthesis of iron-decorated boron nitride quantum dots (Fe@BNQDs)
First, boron nitride quantum dots (BNQDs) were prepared using the hydrothermal method.33 For every 0.3 g boric acid, 10 mL ammonia 25% was added. The mixture was mixed on a magnetic stirrer for half an hour, then the solution was placed in a Teflon-lined autoclave and heated in an oven for 24 hours at 200 °C. The resulting solution was clear and gave fluorescence with blue-green emission under the UVA light. The solvent was then evaporated, yielding white powder. After this step, for each gram of BNQDs, 2.02 mL of 0.1 M Fe(NO3)3·9H2O was added with continuous stirring, and then the solution was placed in a microwave oven at 900 W for 20 minutes. The resulting particles were put in a muffle furnace at 750 °C for roughly one hour, Fig. 1 shows the steps in the synthesis of Fe@BNQDs. Finally, the powder was purified, washed several times with deionized water, dried, and used in photodegradation applications.
2.4. Computational study
The ab initio calculations were performed on a plane-wave basis using DFT's pseudopotential methods. All computations were performed using the CASTEP and Dmol3 codes in Material Studio version 2017. We applied non-local-functional generalized gradient approximation (GGA) meta-GGA34 and hybrid functional including (Becke-3 Parameter-Lee–Yang–Parr) B3LYP35 and (Heyd–Scuseria–Ernzerhof) exchange–correlation functional HSE06.
Each exchange–correlation (XC) functional was computed using a kinetic energy cut-off: 500 eV at norm-conserving pseudopotentials. The Brillouin zone integration was performed using a 4 × 4 × 4 k-point Monkhorst–Pack grid and the self-consistent field (SCF) tolerance was 2 × 10−6 (eV per atom). The calculated lattice parameter and band gap values in (eV) are shown in Tables S2 and S3,† respectively. Fig. 6A and B show the band structure and the total density of state for the Fe@BNQDs photocatalysts. Hybrid functional B3LYP offered better results and was consistent with the observed data, giving the Eg of about 1.839 eV and 2.295 eV as direct and indirect band gaps, respectively. In addition, this method was used to analyze the band structures and densities of states.
For the band edge study in the vacuum, we did not use the frozen core approximation, the full simulation was carried out with the Fe@BNQD (100) slab model with a lattice parameter of: a = b= 3.8 Å and c = 17.98 Å in order to avoid the effect of interaction between layers, including 8 atoms. The calculation was carried out using the Dmol3 code for the work function calculation, The (GGA) was applied to treat correlation effects and electron exchange in the (PBE) formulation, and the basis set was determined as the double-numerical plus polarization (DNP) for this job. To improve the results, a 0.005 Ha Fermi-smearing with a 4.6 real space cutoff was used. For the geometry optimization, and work function, respectively, convergence tolerance of 10–5 Ha, 0.002 Ha, and 0.005 was used. The k-points were set at 4 × 4 × 1. The optical properties of Fe@BNQDs can be illustrated from the complex dielectric function, ε(ω) = ε1(ω) + iε2(ω), which is related to the interaction between photons and electrons. The momentum matrix elements between the occupied levels in the VBM and the vacant levels in the CBM were utilized to calculate the imaginary part 2(ω). The Kramer–Kronig equation can then be applied to derive the real part 1(ω) of the dielectric function from 2(ω). All others, including the electron energy-loss spectrum, optical conductivity, extinction coefficient, and reflectivity, can be determined from 1(ω) and 2(ω).
2.5. Photo-Fenton activity
The photocatalytic activity of Fe@BNQDs was evaluated through the degradation of folic acid under 5 W of the blue LED (Light Bulb LED GU10 5W 400Lm Grow, GREENICE Co., Madrid, Spain). An ultraviolet water sterilization lamp 220–240 V 50/6 Hz (UVCD215 TS 6 W) was used as a source of UV irradiation. Prior to illumination, according to Table 1, Fe@BNQDs along with H2O2 were dispersed into 25 mL of the solution containing the test compound, and then magnetically stirred in the dark for 60 min to establish an adsorption–desorption equilibrium. At predetermined intervals, 3 mL of the suspension was taken and centrifuged at 8000 rpm for 6 min to remove Fe@BNQDs. Then, the absorbance spectra of the folic acid in the supernatants were analyzed using a UV-Vis spectrophotometer at 282 nm. The initial concentration was kept constant at 15 ppm.
Table 1 Levels of the parameters studied in BBD statistical experiments
Independent variable |
Unit |
Levels |
−1 (low) |
0 (middle) |
+1 (high) |
Fe@BNQDs |
g/100 mL H2O |
0 |
0.05 |
0.1 |
H2O2 |
M |
0 |
0.2 |
0.4 |
Temperature |
°C |
20 |
30 |
40 |
To determine the ROS, isopropyl alcohol (IPA) was used as the ˙OH (hydroxyl radical) scavenger, ascorbic acid as ˙O2− (superoxide radical) trapping species, sodium oxalate and potassium bromate were added as scavengers for h+ and e−, respectively.28,36–39
3. Result and discussion
3.1. Characterizations of Fe@BNQDs
The FTIR spectra were used to investigate the formation of BNQDs and then the formation of Fe@BNQDs. Fig. 2A shows the FTIR spectra of BNQDs and Fe@BNQDs, respectively. The peaks at 457.86 cm−1, 506.33 cm−1, and 545.75 cm−1 are assigned for BNQDs.40 The peaks at 642.35 cm−1 and 693.19 cm−1, are assigned to B–O bond vibrations.41,42 Another peak was observed at 781.11 cm−1 due to the amorphous nature of BNQDs and the B–N–B bending mode.40,43–46 The peak at 924.75 cm−1 is for the B–N–O vibration mode.40,47 The peaks at 1022.77 cm−1, 1093.4 cm−1, 1194.87 cm−1, and 1234.03 are similar to the related results of BNQDs elsewhere.40,44–46,48 Moreover, two peaks at 2261.93 cm−1 and 2358.83 cm−1 resulted from the bending vibration of B–H. The presence of oxygen functional groups is clear from the FTIR spectra of both BNQDs and Fe@BNQDs. The broad peak at 1450 cm−1 is due to the bending mode of B–OH41 and the stretching mode of B–N.42 In addition, a peak at 2498.22 cm−1 and a broad peak at 3207.55 cm−1 have resulted from the stretching vibration mode of B–OH and B–NH2.44,46,49
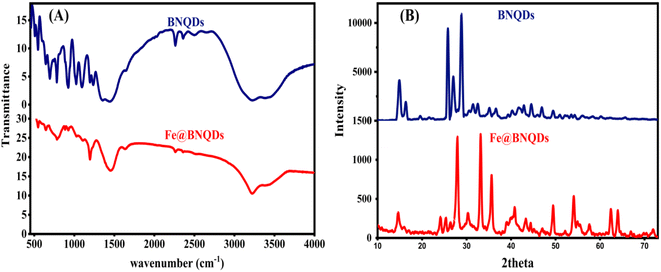 |
| Fig. 2 XRD and FTIR pattern of the fabricated Fe@BNQDs: (A) FTIR of BNQDs and Fe@BNQDs, (B) XRD of BNQDs and Fe@BNQDs. | |
Compared with the FTIR spectrum of Fe@BNQDs, there were some additional signals after BNQDs were decorated with Fe atoms; the bands at 547.09 cm−1 have resulted from the Fe–N stretching mode,50 and 642.8 cm−1 is ascribed to the Fe–O stretching vibration mode,51–53 the bands at 1633.46 cm−1 and 3220.28 cm−1 correspond to Fe–OH bending and stretching vibration, respectively.52–54
The crystal structure of the product was analyzed using powder XRD spectra. Fig. 2B shows the XRD pattern of BNQDs; the diffraction peaks of 2θ (theta) equal to 14.77°, 16.12°, 25.12°, 26.27°, 30.52°, 31.52°, 34.02°, 35.42°, 38.02°, 38.92°, 40.37°, 40.72°, 41.37°, 42.97° can be indexed to the corresponding crystal planes 111, 020, 202, 122, 222, 113, 213, 322, 313, 422, 242 that match from the JCPDS card (no. 40-65-373).55 Another peak at 28.02° is related to the face 002.56
Fig. 2B displays the XRD pattern of Fe@BNQDs, the peaks are located at 2θ (theta) equal to 14.62° (boron nitride, BN, and Fe3B),57 24.15° (Fe2B),58 26.4° (BN), 27.93° (BN Fe2B),58 33.1° (Fe3B),57 35.62° (Fe2B),58 40.83° (BN and FeN0.06),59 43.35° (BN, Fe2N),60 44.4° (BN) 49.48° (Fe2B),58 54.1° (Fe3B),57 54.93° (BN), 55.48° (BN), 57.62° (Fe3B),57 62.45° (Fe2N),60 64° (Fe2N),60 71.93° (BN, Fe2N),60 these data match with those obtained from the JCPDS cards (no. 96-101-1241,61 no. 96-201-6174,62 no. 96-101-0603,63 and 96-101-0332 (ref. 64)). The other two peaks, 25.35° (BN) and 30.33° (BN and Fe2N)60 are found in other ref. 65 and 66.
An XRD pattern was used to measure the crystallinity index (CI),28,67,68 which was found to be 68.8 for BNQDs before decoration with Fe and 76.6 for Fe@BNQDs. It is worth mentioning that CI was measured from the ratio of the integrated area of all crystalline peaks to the total integrated area under the XRD peaks. The FTIR spectra were characterized to investigate the formation of BNQDs and then the formation of Fe@BNQDs.
The detection of oxygen, iron, nitrogen, and boron is shown in Fig. 3B, which corresponds to the EDX spectra of the Fe@BNQDs sample. It is worth mentioning that the presence of oxygen is due to the hydroxyl functionalized on the surface, which makes nanoparticles soluble in water.69,70 However, the high content of oxygen that appeared in the EDX map (Fig. 3B) is due to the hydrophilic nature of BNQDs, which is due to the functionalized hydroxyl groups on its edge.26,27,32,71 Fig. 3A shows the morphology of Fe@BNQDs as imaged by SEM. It can be clearly seen that sphere-like nanostructures were prepared with sizes between 60 and 65 nm. In addition, the Scherrer equation and the Williamson-Hall (W–H) equation were applied to measure the average particle size, and it was found that the average particle size of Fe@BNQDs was about 26 nm according to the Scherrer equation and 31 nm according to the W–H equation.
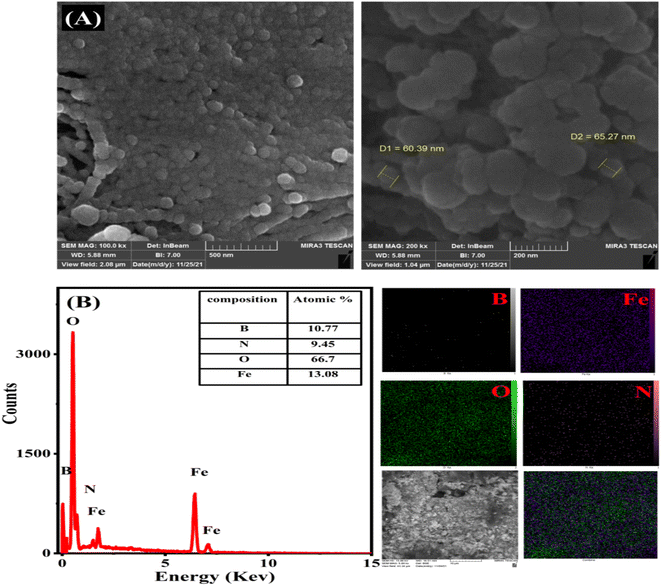 |
| Fig. 3 (A) SEM images of Fe@BNQDs and (B) EDX spectrum and total map of the elemental ratio of Fe@BNQDs. | |
3.2. Photocatalytic degradation
Fe@BNQDs were prepared and subjected to the heterogeneous photo-Fenton process. To study the effects of H2O2, Fe@BNQDs, and temperature, the design of the experiment (DOE) and statistical analysis were performed. All the operational variables and their levels applied for designing the experiment are summarized in Tables 1 and 2.
Table 2 Central composite design matrix Box–Behnken design BBD with fitted and observed valuesa
Run order |
Fe@BNQDs (g/100 mL) |
H2O2 (M) |
Temp. (°C) |
% of degradation, observed |
% of degradation, fitted |
pH was kept constant at 7, and 1 hour photodegradation all Fe@BNQDs samples were adsorption–desorption equilibrated for one hour in the dark, and the light source was blue LED light 5 × 1 W. |
1 |
0 |
0 |
30 |
0 |
−1.684 |
2 |
0.1 |
0 |
30 |
1.77 |
1.143 |
3 |
0 |
0.4 |
30 |
0 |
0.344 |
4 |
0.1 |
0.4 |
30 |
6.38 |
7.781 |
5 |
0 |
0.2 |
30 |
0 |
1.278 |
6 |
0.1 |
0.2 |
20 |
9.58 |
9.235 |
7 |
0 |
0.2 |
40 |
0 |
0.062 |
8 |
0.1 |
0.2 |
40 |
3.93 |
2.369 |
9 |
0.05 |
0 |
20 |
0.7 |
1.248 |
10 |
0.05 |
0.4 |
20 |
12.2 |
10.719 |
11 |
0.05 |
0 |
40 |
0.58 |
2.344 |
12 |
0.05 |
0.4 |
40 |
1.805 |
1.540 |
13 |
0.05 |
0.2 |
30 |
6.12 |
5.162 |
14 |
0.05 |
0.2 |
30 |
4.72 |
5.162 |
15 |
0.05 |
0.2 |
30 |
5.2126 |
5.162 |
16 |
0.1 |
0.2 |
30 |
4.6 |
5.732 |
Response surface methodology (RSM) is a statistical analysis method that utilizes the experimentally obtained quantitative data and, from the design of the experiment and based on practical data, develops the regression equation, which is beneficial for the optimization and prediction of the effect of operating parameters and conditions in the photo-Fenton degradation process.72,73
The study was applied by utilizing the widely used Box–Behnken design (BBD). The main advantage of the BBD study is that it reduces the total number of experiments required to determine the photocatalysis degradation yield.4,73–75 The BBD was performed for three independent variables, including H2O2 concentration, Fe@BNQDs dose, and temperature. The observed dependent variable was the degradation percentage of folic acid. Three levels were determined for each independent variable, as shown in Table 1. A total of 16 runs were assigned and then analyzed.
The results of the ANOVA for photodegradation efficiency and the RSM model fitting of folic acid removal using Fe@BNQDs are presented in Table S1.† The ANOVA analyses were based on the F-test method and p-values. For data manipulation, the proposed parameter was deemed significant at a 95% confidence interval.74,75 The lack of fit (LOF) is another parameter used to determine the validity of the model, which exhibits and determines the random error in the achieved data. LOF is supposed to be not significant in a reliable model; here, LOF has a value of 8.21 as an F-test and 0.111 as a p-value, which is not significant, so the model is reliable.
From the ANOVA results shown in Table S1,† it can be concluded that the overall regression is significant with a p-value of 0.027. Regarding the regression equation with linear, square, and interaction terms for all selected parameters, the results show that Fe@BNQDs dose and H2O2 are affecting the percentage of degradation linearly with p-values of 0.026 and 0.014, respectively, but there is no significant effect when the parameter values are squared. In addition, the interaction between the peroxide concentration and temperature gives a significant result with a p-value of 0.024. It can lead to the conclusion that the terms are affecting the degradation yield linearly.
The regression coefficients were determined, and the results were analyzed using Minitab software version 17. The quality of the fit for the model was expressed from the p-value and correlation coefficient (R2) of 91.41%. The calculated coefficient from the quadratic regression equation is shown in Table S1.† The magnitude and sign of the coefficients represent the influence of each parameter over the response. According to the coefficient presented from the quadratic regression model, the term with the highest coefficient is for the Fe@BNQDs dose with a value of 192.879, which means it is a dominant factor in the overall responses. The positive value of the coefficient means a synergetic effect; however, the negative value of the coefficient means an antagonistic effect. Specifically, the interaction term of temperature and H2O2 concentration by a factor of −1.284 shows the most antagonistic effect on the degradation of Fe@BNQDs, which have a significant p-value of 0.024.
The observed degradation percentage of folic acid varied between zero degradation in the absence of a photocatalyst and 12.2% in the presence of a photocatalyst after one-hour irradiation by a LED-5 W visible lamp, and the model prediction was matched with the experimental results satisfactorily. Control experiments were performed using Fe ions alone along with peroxide and without BNQDs and the result was zero degradation, BNQDs as photocatalysts were also tested and they were not active under visible light; in addition, another experiment for Fe@BNQDs was carried out in the dark, and the result showed no degradation. Thus, we can conclude from these experiments that Fe@BNQDs are responsible for and are involved in photo-Fenton degradation.
3.2.1 Kinetic study and efficiency of photocatalysis. To study the kinetics of photodegradation, first, the best experiment was selected, which is run number 10 in Table 2. The experiment was conducted under the same conditions and radiation source. The rate of folic acid degradation was estimated by examining the degradation at 282 nm. To determine the order of degradation, the log–log graphical method, and the integral and deferential rate laws were applied. The goodness of the kinetic order can also be evaluated by the correlation coefficient (R2) when the value of R2 is close to unity.3,4,8,76 It appears that the degradation at peak 282 nm is the first order with an R2 value of 0.998 compared to the second order, which is 0.97, as shown in Fig. 4A and B, the same procedure was repeated when irradiating with UV light, as shown in Fig. 4C and D, respectively. The result shows that the degradation percentage after one hour of irradiation was tripled in the case of 35% degradation with UV light. As regards the rate constant, degradation that occurred under irradiation with UV light was 12.77 folds faster than that with visible light, with rate constants equal to 6.4 × 10−4 min−1 and 0.0082 min−1 for visible light and ultraviolet, respectively.
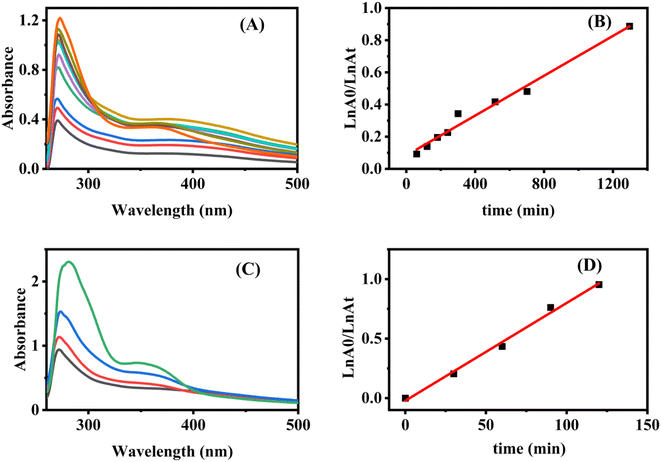 |
| Fig. 4 The change in UV-Vis spectra as a function of time of 15 ppm folic acid by (A) visible light, (C) ultraviolet light. The integral kinetic plot of (B) 1st order visible light and (D) 1st order ultraviolet light at 25 °C. | |
With respect to the degradation efficiency, the sample was irradiated for 24 hours each time. From Fig. S1,† it can be concluded that the efficiency was 77.1% after 24 hours of irradiation of folic acid with Fe@BNQDs using 5 W of blue LED light. The efficiency dropped to 58.5% for the next 24 hours, and then to 36.36% for the next 24 hours. The photocatalyst was then dried and activated at 150 °C for two hours and then used in photodegradation for the next 24 hours. The efficiency was found to increase to 58.61%.
3.2.2 Effect of initial pH on photocatalysis. The photocatalytic degradation of folic acid over Fe@BNQDs at five different pHs was studied. Fig. 5C illustrates the effect of different initial pHs on the photocatalytic efficiency. The results showed that the pH of the solution had a great influence on the photodegradation of folic acid over Fe@BNQDs. In general, as the pH of the solution increases, the degradation efficiency decreases, and the best degradation yield was at an acidic medium pH = 3, where 24.5% was removed, and at a very basic solution pH = 11, the photocatalyst was inactive. As a general rule, the electrostatic effect influences the adsorption and desorption between the surface of the photocatalyst and target molecules; therefore, an oppositely charged photocatalyst and pollutants cause attraction. Accordingly, the point of zero charge, pHPZC (isoelectric point pH) plays an important role in determining the critical value and magnitude of the charge held between the photocatalyst and pollutants during the photocatalytic processes,11,77,78 the oxide-containing photocatalysts are amphoteric in nature, and they turn to an acidic or basic surface as in the following eqn (1) and (2): |
Photocatalyst–OH + H+ → photocatalyst–OH2+, pH < pHPZC
| (1) |
|
Photocatalyst–OH + OH− → photocatalyst–O− + H2O, pH > pHPZC
| (2) |
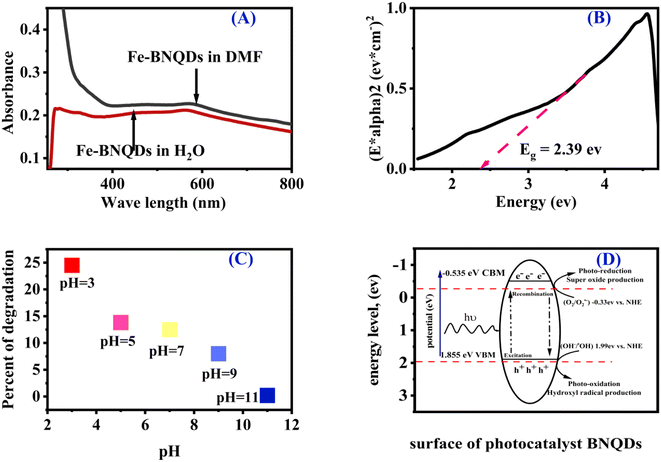 |
| Fig. 5 (A) UV-vis. Absorption spectra. (B) Tauc plot (alpha hν)2 versus energy band gap (hν) of Fe@BNQDs. (C) Effect of initial pH on the photocatalytic degradation of folic acid over Fe@BNQDs and (D) Schematic diagram illustrates the principal mechanism of Fe@BNQDs. | |
Based on this information, it could be deduced that the initial pH determines the attraction or repulsion between the surface of the photocatalyst and pollutants. At pH values lower than pHPZC, the photocatalyst surface is positively charged, so, it is able to attract negatively charged molecules. At pH equal to pHPZC, there is no charge on its surface, while at pH greater than pHPZC, the surface of the photocatalyst is negatively charged.28,77,78 Regarding the folic acid degradation over Fe@BNQDs, the acidic medium pH 3 was optimum, it can be concluded that the pH 3 is close to the pHPZC of Fe@BNQDs; as a result, the surface is positively charged, which is able to attract the negatively charged folic acid molecule. As has been seen in trapping experiments, which confirms that the h+ plays a major role in the photodegradation process of folic acid over Fe@BNQDs.
3.3. Photo-Fenton mechanism and scavenger trapping experiments
The progress of the photocatalytic reaction and mineralization of chemical waste was attained by the transfer and transition of active species h+, e−, ˙O2−, and ˙OH. Which made it possible to confirm the major active radical species that take part in the photodegradation process and the photocatalyst reaction mechanism. As shown in Fig. S3,† it can be seen that the degradation of folic acid was hardly affected by isopropyl alcohol (IPA), while the degradation percentage declined significantly with the addition of Na2C2O4 and decreased in the presence of ascorbic acid and KBrO3. Accordingly, the results indicated that the degradation percentage lowered in the presence of h+, e−, and ˙O2− trapping species, indicating that h+, e−, and O2−˙ were responsible for folic acid degradation over Fe@BNQDs PC. The addition of IPA to the photocatalytic reaction was not significant, which demonstrates that Fe@BNQDs are inert for the production of OH−/˙OH due to the position of the band edges.
Regarding the band structure of the prepared photocatalyst, first UV-visible absorption spectra were recorded both in water and the DMF solvent, as shown in Fig. 5A, then a Tauc plot was plotted, and the results were in good agreement with those from the theoretical DFT calculation.79,80 The Eg of Fe@BNQDs was evaluated from the Tauc plot and it was estimated to be 2.39 eV. From the optical absorption spectra in Fig. 5A, we found that the Fe@BNQDs showed optical absorption that extended more into the visible region, which indicates more energy harvesting.
To elucidate the energy level (band edges) of the prepared nanostructured photocatalysts, photo redox potential was calculated, as shown in Fig. 5D. EVB was estimated to be 1.855 eV and ECB −0.535 eV. The potentials of Fe@BNQDs were measured using the Mulliken electronegativity rules:28,37,81–86
As presented in Fig. 5D, the energy of the valence band maximum EVB of Fe@BNQDs was estimated to be 1.855 eV, which is more negative than that of (OH−/˙OH) (1.99 eV versus NHE); as such, the hole (h+) at VBM is unable to oxidize OH− to produce the hydroxyl radical (˙OH); simultaneously, the CBM potential was measured to be −0.535 eV versus NHE, which is less positive than that of O2/O2˙− −0.33 eV versus NHE,22,38 as such, the electron at the CBM band edge can react with O2 to form O2˙−; these results are in good agreement with the scavenger test, which strongly indicates that h+ and superoxide O2˙− radical are both the active species involved in the photodegradation of folic acid over Fe@BNQDs.
3.4. Computational simulation
To gain a better understanding of the prepared nanomaterials, theoretical calculations were performed. The perfect band gap is a direct gap semiconductor of 1.839 eV at the X point and an indirect band gap of 2.295 eV at the M point at the Valance Band Minimum (VBM) to the G–R at the Conduction Band Maximum (CBM), as shown in Fig. 7A. The band gap of the Fe@BNQDs was observed to decrease after decorating with Fe atoms, from 3.79 eV to 2.39 eV for Fe@BNQDs. It is obvious from Table S3,† which reports Eg the most XC functionals that exhibit better agreement with the experimental results are B3LYP, therefore, the B3LYP functional was used to interpret, discuss, and analyze the band structure and density of states as it gives correct and sufficient data.
The partial density of state (PDOS) of the electronic levels of Fe@BNQDs is plotted in Fig. 6B. It can be noted that the Fermi level is close to VBM. As regard to Fe atoms, the d-orbital showed a high DOS that covers the Fermi level at the valence band, which appears as two broad peaks within the range of (−2.98 to 0.55 eV), which explains that most of the DOS of Fe atoms are located at the 3d-orbital. The bottom of the conduction band consists of mixed 4p and 3d-orbitals with medium intensity at 2.99 eV, Fig. 6B. With respect to boron atoms, they have one broad peak near VBM at −1.83 eV and another peak at −3.94 eV, both belonging to 2p orbitals. Regarding CBM, there are three peaks that belong to 2p-orbitals within the range of (2.34 to 6.48). As for nitrogen atoms, one peak overlaps with the Fermi level at VBM, and one peak is observed at −1.74 eV; at CBM, a peak is observed at 2.82 eV, which belongs to the 2p-orbital with a high DOS and the 2s-orbital with a lower DOS. It can be seen from Fig. 6B that the s-orbitals for Fe, B, and N atoms have a minimum contribution to DOS at VBM and CBM.
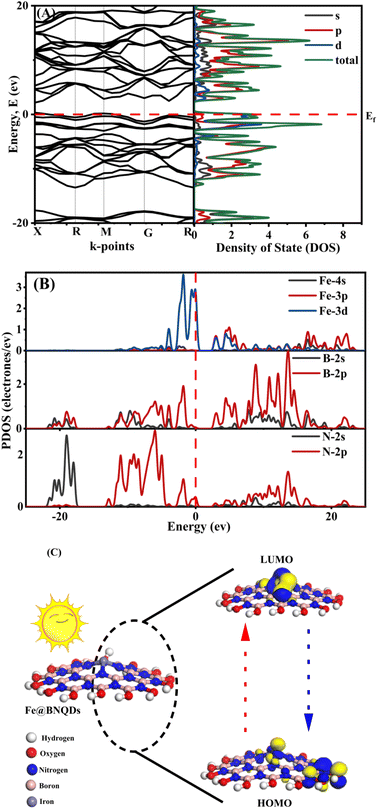 |
| Fig. 6 (A) Band structure and total density of state for pure Fe@BNQDs photo-catalyst, (B) The PDOS of the Fe@BNQDs utilizing DFT/B3LYP/norm-conserving. (C) Molecular orbital transferee during excitation from HOMO to LUMO by the DFT/GGA/PBE Dmol3 code. | |
As shown in Table S2,† the optimized lattice parameter and bond length for Fe@BNQDs and hexagonal boron nitride (h-BN) were computed utilizing PBE exchange functionals.28,87,88 It is reported that the total volume of the cell increased after the insertion of the Fe atom and that the overall structure changed. That has an effect on the B–N bond length and the shape of the cell.
To investigate the electronic density available on elements in the ground and excited states of Fe@BNQDs, first, a single sheet of Fe@BNQD 1.5 nm was optimized. The highest occupied molecular orbital (HOMO) and the lowest unoccupied molecular orbital (LUMO) were calculated using the DFT/GGA/PBE Dmol3 code. As illustrated in Fig. 6C, the electron density at the hydroxyl group of BNQDs in HOMO (ground state) is concentrated at the Fe atom in LUMO (excited state), implying that the OH− functional groups at the edges of the BNQDs inject electrons to the Fe atom and are thus most are involved in the photocatalysis process.
In this study, we characterized the structural stability of the Fe@BNQDs photocatalyst at high temperatures using thermodynamic parameters. The standard statistical thermodynamic functions: Gibbs free energy, heat capacity, entropy, and enthalpy for Fe@BNQDs were provided from the vibrational harmonic frequencies in the temperature range of 25–1000 K, as shown in Fig. S2.† In general, it can be noticed that these thermodynamic functions are increasing with temperature except for Gibbs free energy;89–91 Gibbs free energy is negative at 825 K, so that, the formation of Fe@BNQDs is only achievable at elevated temperatures. At this point, the value of entropy is 466 cal mol−1 K−1 and the enthalpy is 379 kcal mol−1 K−1.
It is well known that one of the crucial characteristics for semiconductors to function as photocatalysts is the position of the valence and conduction band edges. It establishes the photochemical reaction's thermodynamic limit, which is carried out by photogenerated electrons and holes. In general, the conduction band and valence band margins of the photocatalyst should be between the redox potentials of the reactants. The band edges in a vacuum were calculated, as shown in Fig. 7, the band edge calculations illustrated that the Fe@BNQDs is an efficient visible-light-driven photocatalyst for the degradation of organic wastes and it may be suitable for water splitting and CO2 reduction as well.
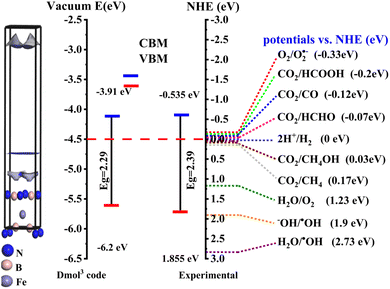 |
| Fig. 7 Bandgaps and band-edge positions with respect to the vacuum level and NHE for the Fe@BNQDs. EVB (red bar) and ECB (blue bar) of the Fe@BNQDs photocatalyst in vacuum and experimental; along with the potentials of CO2 reduction, water splitting, and photodegradation processes at normal hydrogen electrode (NHE) scale at pH = 0. | |
In optoelectronic fields, such as photocatalysis, the optical characteristics of semiconductor materials are of utmost significance since they can explain the behavior of the photocatalyst during photochemical interactions. The spectra of Fe@BNQDs as a function of photon energy ranging from 0.01 up to 60 eV are shown in Fig. 8. The extension of electrical transport to large (optical) frequencies is the optical conductivity; this quantitative evaluation is contact-free and mostly responsive to charged responses. Fig. 8A shows the optical conductivity spectra; the maximum optical conductivity regarding the UV visible region is shown at 3.2 eV.
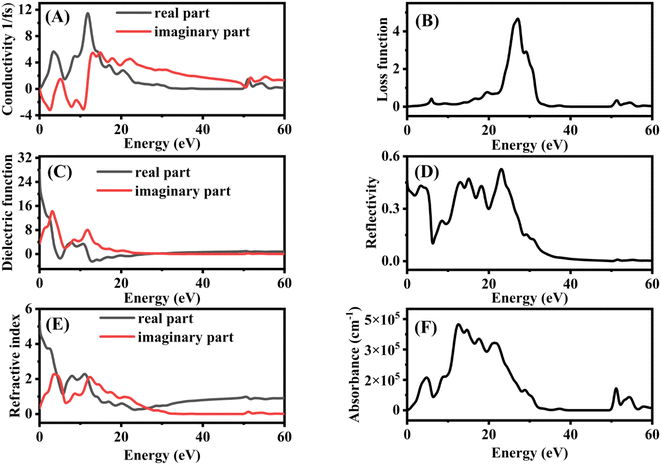 |
| Fig. 8 Optical properties spectra of Fe@BNQDs (A) conductivity, (B) loss function, (C) dielectric function, (D) reflectivity, (E) refractive index, and (F) absorption. | |
Another important optical characteristic is the electron energy loss function. The energy loss experienced by rapid electrons moving through a substance is described by the loss function. The surface plasmon resonance is linked to the loss function's peaks, Fig. 8B shows the loss function spectra; there are no peaks observed in the UV visible area, therefore, we can say that this photocatalyst has an absent plasmonic effect. The dielectric function plays a significant role in explaining how radiation behaves inside a photocatalyst. The imaginary portion of the dielectric function is related to the band structure and absorption characteristics of the photocatalysts. The dielectric function demonstrates what a passing light beam or other electric fields would do to a photocatalyst. The complex dielectric function's imaginary part ε2(ω) denotes the quantity of absorption in a material, while its real part ε1(ω) denotes the polarization of the photocatalyst caused by the propagating light. Fig. 8C shows the dielectric function spectra, the two major peaks are observed at 3.2 and 11.8 eV. The frequent inter-band transitions between VBM and CB are represented by the imaginary dielectric function, which is a significant quantity. Fe@BNQDs have a first peak that is caused by the optical transition according to the band gap value, which is 2.4 eV, followed by two further peaks. The reflectivity spectra, shown in Fig. 8D, exhibit the optical reaction of the systems to the incident light. In terms of reflectivity, a lower energy bandgap results in a higher ε1(0) value and hence a higher reflection coefficient, and vice versa, the reflectivity of Fe@BNQDs is more significant in the infrared to the UV region.
The refractive index explains how interactions with photocatalysts alter light waves (amount of light reflected or bent). The imaginary part, which is called the extension coefficient of the refractive index, indicates the reduction of an electromagnetic wave moving through a photocatalyst, whereas, the real part measures the phase velocity of the electromagnetic wave in a medium. The real and imaginary components of the refractive index are connected by the complex dielectric function; Fig. 8E shows refractive index spectra. Fe@BNQDs exhibit a high value of the extension coefficient at the infrared region and a decrease in the visible and UV regions, while the real part has a low value in the infrared, then increases in the visible region and decreases again at the UV region. Reflectivity refers to the ratio of the amount of light that strikes a substance to the amount of light that is reflected from it. By synchronizing the electric and magnetic fields at the surface, it is possible to obtain the reflection coefficient for the basic case of normal incidence onto a plane surface. The absorption coefficient offers useful information when the UV-Vis energy is applied to achieve optimum efficiency, and absorption coefficient spectra are shown in Fig. 8F; in general, Fe@BNQDs exhibit good adsorption in the UV visible region, which is of interest in photocatalysis.88,92–97
4. Conclusions
A novel magnetically-separable and visible-light-driven photo-Fenton catalyst (Fe@BNQDs) was synthesized and characterized. Fe@BNQDs indicated efficient degradation of folic acid. The RSM based on BBD was employed to demonstrate the degradation efficiency of folic acid under the influence of three variables, including Fe@BNQDs dose, H2O2 concentration, and temperature. According to the ANOVA and regression coefficients, the results showed that the effect of the operating variable is a linear positive p-value of 0.013, and the effect of H2O2 and temperature interaction was a negative p-value of 0.024. The photocatalytic efficiency tests revealed that the Fe@BNQDs have good stability; the experiment lasted for 96 hours under irradiation. Trapping experiments were performed to investigate the active species and possible mechanisms involved in photodegradation over Fe@BNQDs. Analysis of the results shows that the Fe@BNQDs are inert toward the production of the ˙OH radical, and the active species are h+, e−, and O2˙. A theoretical study was performed on the basis of the DFT framework, regarding the electronic structure. The B3LYP/DFT hybrid function gives a better result and is closer to the experimental value.
Conflicts of interest
The authors declare there are no conflicts to declare.
References
- R. C. Pawar and C. S. Lee, Heterogeneous Nanocomposite-Photocatalysis for Water Purification, 2015, DOI:10.1016/C2014-0-02650-0.
- A. J. Abdul-Zahra, M. S. Mashkour, A. M. Juda and H. A. Al-Sultani, Photocatalytic degradation of paracetamol and procaine, Int. J. ChemTech Res., 2016, 9, 412–425 CAS.
- S. A. Idrees, S. A. Naman and A. Shorachi, Kinetic and thermodynamic study of Trifluralin photo-degradation by ultra violet light, IOP Conf. Ser. Mater. Sci. Eng., 2018, 454, 12045 CrossRef.
- M. M. Molla-Babaker and S. A. Idreesb, Degradation of Congo Red Dye Using Homogeneous Photo Fenton Catalyst Coupled with Oxygen Kinetics and Statistical Analysis, Asian J. Appl. Chem. Res., 2020, 6, 1–9 CrossRef.
- V. Loddo, M. Bellardita, G. Camera-Roda, F. Parrino and L. Palmisano, Heterogeneous Photocatalysis: A Promising Advanced Oxidation Process, Current Trends and Future Developments on (Bio-) Membranes: Photocatalytic Membranes and Photocatalytic Membrane Reactors, Elsevier Inc., 2018, DOI:10.1016/B978-0-12-813549-5.00001-3.
- C. B. Mendive and D. W. Bahnemann, Mechanisms in Heterogeneous Photocatalysis, Encycl. Mater. Sci. Technol., 2011, 1–5, DOI:10.1016/b978-0-08-043152-9.02273-9.
- A. R. Khataee and M. Fathinia Recent Advances in Photocatalytic Processes by Nanomaterials, New and Future Developments in Catalysis: Catalysis by Nanoparticles, Elsevier B.V., 2013, DOI:10.1016/B978-0-444-53874-1.00011-1.
- S. A. Idrees, R. N. Salih, K. Bashir and A. A. Hamasaeed, Kinetic Study of Congo-Red Photo-Catalytic Degradation in Aqueous Media Using Zinc Oxide as Photo Catalyst Under Led Light, Sci. J. Univ. Zakho, 2021, 9, 20–24 CrossRef.
- M. R. Ashwin Kishore and P. Ravindran, Tailoring the Electronic Band Gap and Band Edge Positions in the C2N Monolayer by P and As Substitution for Photocatalytic Water Splitting, J. Phys. Chem. C, 2017, 121, 22216–22224 CrossRef CAS.
- K. M. Omer, N. N. Mohammad and S. O. Baban, Up-Conversion Fluorescence of Phosphorous and Nitrogen Co-Doped Carbon Quantum Dots (CDs) Coupled with Weak LED Light Source for Full-Spectrum Driven Photocatalytic Degradation via ZnO-CDs Nanocomposites, Catal. Lett., 2018, 148, 2746–2755 CrossRef CAS.
- U. J. Ahile, R. A. Wuana, A. U. Itodo, R. Sha'Ato and R. F. Dantas, Stability of iron chelates during photo-Fenton process: The role of pH, hydroxyl radical attack and temperature, J. Water Process Eng., 2020, 36, 101320 CrossRef.
- F. Velichkova, Heterogeneous Fenton and Photo-Fenton Oxidation for Paracetamol Removal Using Iron Containing ZSM-5 Zeolite as Catalyst, AIChE J., 2012, 59, 215–228 Search PubMed.
- F. Audino, et al., A kinetic study for the Fenton and photo-Fenton paracetamol degradation in an annular photoreactor, Environ. Sci. Pollut. Res., 2019, 26, 4312–4323 CrossRef CAS PubMed.
- S. Hashemian, Fenton-like oxidation of malachite green solutions: Kinetic and thermodynamic study, J. Chem., 2013, 2013, 809318 Search PubMed.
- J. H. Sun, S. P. Sun, G. L. Wang and L. P. Qiao, Degradation of azo dye Amido black 10B in aqueous solution by Fenton oxidation process, Dyes Pigm., 2007, 74, 647–652 CrossRef CAS.
- S. X. Liu, Z. P. Qu, X. W. Han and C. L. Sun, A mechanism for enhanced photocatalytic activity of silver-loaded titanium dioxide, Catal. Today, 2004, 93–95, 877–884 CrossRef CAS.
- H. Wang, S. Baek, J. Lee and S. Lim, High photocatalytic activity of silver-loaded ZnO-SnO2 coupled catalysts, Chem. Eng. J. J., 2009, 146, 355–361 CrossRef CAS.
- S. S. Wong, M. J. Hülsey, H. An and N. Yan, Quantum yield enhancement in the photocatalytic HCOOH decomposition to H2 under periodic illumination, Catal. Sci. Technol., 2022, 12, 5217–5228 RSC.
- C. B. Mendive, T. Bredow, J. Schneider, M. Blesa and D. Bahnemann, Oxalic acid at the TiO2/water interface under UV(A) illumination: Surface reaction mechanisms, J. Catal., 2015, 322, 60–72 CrossRef CAS.
- M. Curti, D. W. Bahnemann and C. B. Mendive, Mechanisms in Heterogeneous Photocatalysis: Titania under UV and Visible Light Illumination, Ref. Modul. Mater. Sci. Mater. Eng., 2016, 1–6, DOI:10.1016/b978-0-12-803581-8.03800-5.
- N. Bühler, K. Meier and J. F. Reber, Photochemical hydrogen production with cadmium sulfide suspensions, J. Phys. Chem., 1984, 88, 3261–3268 CrossRef.
- X. Ruan, et al., A visible-light-driven Z-scheme CdS/Bi12GeO20 heterostructure with enhanced photocatalytic degradation of various organics and the reduction of aqueous Cr(VI), J. Colloid Interface Sci., 2019, 543, 317–327 CrossRef CAS PubMed.
- M. R. Ashwin Kishore, K. Larsson and P. Ravindran, Two-dimensional CdX/C2N (X = S, Se) heterostructures as potential photocatalysts for water splitting: A DFT study, ACS Omega, 2020, 5, 23762–23768 CrossRef CAS PubMed.
- B. Zhu, P. Xia, Y. Li, W. Ho and J. Yu, Fabrication and photocatalytic activity enhanced mechanism of direct Z-scheme g-C3N4/Ag2WO4 photocatalyst, Appl. Surf. Sci., 2017, 391, 175–183 CrossRef CAS.
- T. Chen, et al., Carbon nitride modified hexagonal boron nitride interface as highly efficient blue LED light-driven photocatalyst, Appl. Catal., B, 2018, 238, 410–421 CrossRef CAS.
- Y. Zhan, et al., Targets regulated formation of boron nitride quantum dots – Gold nanoparticles nanocomposites for ultrasensitive detection of acetylcholinesterase activity and its inhibitors, Sens. Actuators, B, 2019, 279, 61–68 CrossRef CAS.
- J. H. Jung, et al., Defect engineering route to boron nitride quantum dots and edge-hydroxylated functionalization for bio-imaging, RSC Adv., 2016, 6, 73939–73946 RSC.
- S. A. Idrees, L. A. Jamil and K. M. Omer, Fabrication Of Novel Metal-Free Phosphorous Doped Boron Nitride As UV. Active Photo-Catalyst, Iran. J. Catal., 2021, 11, 405–416 CAS.
- Z. He, et al., Formation of heterostructures via direct growth CN on h-BN porous nanosheets for metal-free photocatalysis, Nano Energy, 2017, 42, 58–68 CrossRef CAS.
- J. Yu, et al., Vertically aligned boron nitride nanosheets: Chemical vapor synthesis, ultraviolet light emission, and superhydrophobicity, ACS Nano, 2010, 4, 414–422 CrossRef CAS PubMed.
- D. Huang, M. Cheng, L. Hu and W. Xiong, Semiconductor/boron nitride composites: synthesis, properties, and photocatalysis applications, Appl. Catal., B, 2018, 238, 6–8 CrossRef.
- S. Angizi, F. Shayeganfar, M. H. Azar and A. Simchi, Surface/edge functionalized boron nitride quantum dots: Spectroscopic fingerprint of bandgap modification by chemical functionalization, Ceram. Int., 2020, 46, 978–985 CrossRef CAS.
- B. Liu, et al., One-Step Synthesis of Boron Nitride Quantum Dots: Simple Chemistry Meets Delicate Nanotechnology, Chem.–Eur. J., 2016, 22, 18899–18907 CrossRef CAS PubMed.
- W. Kohn and L. J. Sham, Self-Consistent Equations Including Exchange and Correlation Effects, Phys. Rev., 1965, 140, A1133–A1137 CrossRef.
- C. Lee, W. Yang and R. G. Parr, Development of the Colle-Salvetti correlation-energy formula into a functional of the electron density, Phys. Rev. B: Condens. Matter Mater. Phys., 1988, 37, 785–789 CrossRef CAS PubMed.
- P. Ju, et al., A novel calcined Bi2WO6/BiVO4 heterojunction photocatalyst with highly enhanced photocatalytic activity, Chem. Eng. J., 2014, 236, 430–437 CrossRef CAS.
- M. Pirhashemi and A. Habibi-Yangjeh, Ultrasonic-assisted preparation of plasmonic ZnO/Ag/Ag2WO4 nanocomposites with high visible-light photocatalytic performance for degradation of organic pollutants, J. Colloid Interface Sci., 2016, 491, 216–229 CrossRef PubMed.
- J. Du, et al., The Research on the Construction and the Photocatalytic Performance of BiOI/NH2-MIL-125(Ti) Composite, Catalysts, 2021, 125, 1–15 Search PubMed.
- F. Cao, et al., An: In situ Bi-decorated BiOBr photocatalyst for synchronously treating multiple antibiotics in water, Nanoscale Adv., 2019, 1, 1124–1129 RSC.
- R. Geick, C. H. Perry and G. Rupprecht, Normal Modes in Hexagonal Boron, Phys. Rev., 1966, 146, 543–547 CrossRef CAS.
- B. Singh, G. Kaur, P. Singh, K. Singh and B. Kumar, Nanostructured Boron Nitride With High Water Dispersibility For Boron Neutron Capture Therapy, Nat. Sci. Rep., 2016, 6, 35535 CAS.
- T. Shen, Highly efficient preparation of hexagonal boron nitride by direct
microwave heating for dye removal, J. Mater. Sci., 2019, 54, 8852–8859 CrossRef CAS.
- D. G. Ortiz, et al., Exfoliation of Hexagonal Boron Nitride (h-BN) in Liquide Phase by Ion Intercalation To cite this version: HAL Id: hal-01926507 Exfoliation of Hexagonal Boron Nitride (h-BN) in Liquide Phase by Ion Intercalation, MDPI Nanomater., 2018, 8, 1–12 Search PubMed.
- L. Zhai, et al., Cyanate ester resin based composites with high toughness and thermal conductivity, RSC Adv., 2019, 9, 5722–5730 RSC.
- D. J. Kester, K. S. Ailey, R. F. Davis, N. Carolina and K. L. More, Phase evolution in boron nitride thin films, Mater. Res. Soc., 1993, 8, 1213–1216 CrossRef CAS.
- B. C. Tang, Y. Bando, Y. Huang, C. Zhi and D. Golberg, Synthetic Routes and Formation Mechanisms of Spherical Boron Nitride Nanoparticles, Adv. Funct. Mater., 2008, 18, 3653–3661 CrossRef.
- P. M. Sudeep, et al., Functionalized boron nitride porous solids, RSC Adv., 2015, 5, 93964–93968 RSC.
- X. Luo, Y. Zhang, C. Zandén, M. Murugesan, Y. Cao, L. Ye and J. Liu, Novel thermal interface materials: boron nitride nanofiber and indium composites for electronics heat dissipation applications, J. Mater. Sci.: Mater. Electron., 2014, 25, 2333–2338 CrossRef CAS.
- L. Chen, et al., Thermal Conductivity Performance of Polypropylene Composites Filled with Polydopamine-Functionalized Hexagonal Boron Nitride, PLoS One, 2017, 1–16, DOI:10.1371/journal.pone.0170523.
- C. Várhelyi, et al., Preparation and characterization of novel [Fe(methylisopropylglyoximato)2(amine)2] mixed chelates, J. Radioanal. Nucl. Chem., 2015, 304, 745–750 CrossRef.
- L. Nalbandian, et al., Magnetic Nanoparticles in Medical Diagnostic Applications: Synthesis, Characterization and Proteins Conjugation, Curr. Nanosci., 2015, 12, 455–468 CrossRef.
- N. R. Jannah and D. Onggo, Synthesis of Fe3O4 nanoparticles for colour removal of printing ink solution, J. Phys. Conf. Ser., 2019, 1245, 1–7 CrossRef.
- A. Azizi, Green Synthesis of Fe3O4 Nanoparticles and Its Application in Preparation of Fe3O4/Cellulose Magnetic Nanocomposite: A Suitable Proposal for Drug Delivery Systems, J. Inorg. Organomet. Polym. Mater., 2020, 30, 3552–3561 CrossRef CAS.
- E. Bertolucci, et al., Chemical and magnetic properties characterization of magnetic nanoparticles, Conf. Rec. - IEEE Instrum. Meas. Technol. Conf., 2015, 1492–1496 Search PubMed.
- G. C. Fortman, A. M. Z. Slawin and S. P. Nolan, Highly active iridium(III)-NHC system for the catalytic B-N bond activation and subsequent solvolysis of ammonia-borane, Organometallics, 2011, 30, 5487–5492 CrossRef CAS.
- C. Huang, et al., Stable colloidal boron nitride nanosheet dispersion and its potential application in catalysis, J. Mater. Chem. A, 2013, 1, 12192–12197 RSC.
- V. A. Barinov, V. A. Tsurin, V. I. Voronin, S. I. Novikov and V. T. Surikov, Mössbauer investigations of the metastable Fe23B 6 phase, Phys. Met. Metallogr., 2006, 101, 456–466 CrossRef.
- C. I. VillaVelázquez-Mendoza, et al., Effect of substrate roughness, time and temperature on the processing of iron boride coatings: Experimental and statistical approaches, Int. J. Surf. Sci. Eng., 2014, 8, 71–91 CrossRef.
- K. H. Jack, The occurrence and the crystal structure of α′′-iron nitride; a new type of interstitial alloy formed during the tempering of nitrogen-martensite, Proc. R. Soc. London, Ser. A, 1951, 208, 216–224 CAS.
- W. T. Zheng, et al., Crystal structures and magnetic properties of Fe–N thin films deposited by dc magnetron sputtering, Powder Diffr., 2004, 19, 352–355 CrossRef CAS.
- L. Pauling and S. B. Hendricks, The crystal structures of hematite and corundum, J. Am. Chem. Soc., 1925, 47, 781–790 CrossRef CAS.
- O. O. Kurakevych and V. L. Solozhenko, Rhombohedral boron subnitride, B13N2, by X-ray powder diffraction, Acta Crystallogr., Sect. C: Cryst. Struct. Commun., 2007, 63, 80–82 CrossRef PubMed.
- A. Brager, An X-ray examination of the structure of boron nitride, Acta Physicochim., 1937, 7, 699–706 CAS.
- O. Hassel, Die kristalustrakture des boronnitride, BN, Nor. Geol., 1927, 9, 266–270 Search PubMed.
- H. Türkez, et al., Synthesis, characterization and cytotoxicity of boron nitride nanoparticles: emphasis on toxicogenomics, Cytotechnology, 2019, 71, 351–361 CrossRef PubMed.
- V. Guerra, et al., 2D boron nitride nanosheets (BNNS) prepared by high-pressure homogenisation: Structure and morphology, Nanoscale, 2018, 10, 19469–19477 RSC.
- S. A. Idrees, L. A. Jamil and K. M. Omer, Silver-Loaded Carbon and Phosphorous Co-Doped Boron Nitride Quantum Dots (Ag@CP-BNQDs) for Efficient Organic Waste Removal: Theoretical and Experimental Investigations, ACS Omega, 2022, 7, 37620–37628 CrossRef CAS PubMed.
- Y. Sa, et al., Are different crystallinity-index-calculating methods of hydroxyapatite efficient and consistent?, New J. Chem., 2017, 41, 5723–5731 RSC.
- Q. Weng, X. Wang, X. Wang, Y. Bando and D. Golberg, Functionalized hexagonal boron nitride nanomaterials: Emerging properties and applications, Chem. Soc. Rev., 2016, 45, 3989–4012 RSC.
- X. Shi, et al., Few-Layer Hydroxyl-Functionalized Boron Nitride Nanosheets for Nanoscale Thermal Management, ACS Appl. Nano Mater., 2020, 3, 2310–2321 CrossRef CAS.
- D. Kim, et al., Sonication-assisted alcoholysis of boron nitride nanotubes for their sidewalls chemical peeling, Chem. Commun., 2015, 51, 7104–7107 RSC.
- S. Debnath, N. Ballav, H. Nyoni, A. Maity and K. Pillay, Optimization and mechanism elucidation of the catalytic photo-degradation of the dyes Eosin Yellow (EY) and Naphthol blue black (NBB) by a polyaniline-coated titanium dioxide nanocomposite, Appl. Catal., B, 2015, 163, 330–342 CrossRef CAS.
- S. A. Idrees and M. K. Ibrahim, Optimization of Congo-Red Photo-Catalytic Degradation by Central Composite Design, in 2018 International Conference on Advanced Science and Engineering (ICOASE), 2018, pp. 389–393, DOI:10.1109/ICOASE.2018.8548919.
- M. Berkani, Y. Kadmi, M. K. Bouchareb, M. Bouhelassa and A. Bouzaza, Combinatıon of a Box-Behnken design technique with response surface methodology for optimization of the photocatalytic mineralization of C.I. Basic Red 46 dye from aqueous solution, Arab. J. Chem., 2020, 13, 8338–8346 CrossRef CAS.
- H. Chaker, N. Ameur, K. Saidi-Bendahou, M. Djennas and S. Fourmentin, Modeling and Box-Behnken design optimization of photocatalytic parameters for efficient removal of dye by lanthanum-doped mesoporous TiO2, J. Environ. Chem. Eng., 2021, 9, 104584 CrossRef CAS.
- K. Xie, H. Zhang, S. Sun and Y. Gao, Functions of boric acid in fabricating TiO2 for photocatalytic degradation of organic contaminants and hydrogen evolution, Mol. Catal., 2019, 479, 110614 CrossRef CAS.
- S. Ghattavi and A. Nezamzadeh-Ejhieh, A brief study on the boosted photocatalytic activity of AgI/WO3/ZnO in the degradation of methylene blue under visible light irradiation, Desalin. Water Treat., 2019, 166, 92–104 CrossRef CAS.
- L. Nadjia, E. Abdelkader and B. Ahmed, Photodegradation study of Congo Red in Aqueous Solution using ZnO/UV-A: Effect of pH And Band Gap of other Semiconductor Groups, J. Chem. Eng. Process Technol., 2011, 02, 1–7 Search PubMed.
- R. Mohammed, S. Ahmed, A. Abdulrahman and S. Hamad, Synthesis and Characterizations of ZnO Thin Films Grown by Physical Vapor Deposition Technique, J. Appl. Sci. Technol. Trends, 2020, 1, 135–139 CrossRef.
- A. F. Abdulrahman, S. M. Ahmed and M. A. Almessiere, Effect of the growth time on the optical properties of ZnO nanorods grown by low temperature method, Dig. J. Nanomater. Biostructures, 2017, 12, 1001–1009 Search PubMed.
- A. Habibi-yangjeh and M. Shekofteh-gohari, Novel magnetic Fe3O4/ZnO/NiWO4 nanocomposites : Enhanced visible-light photocatalytic performance through p–n heterojunctions, Sep. Purif. Technol., 2017, 184, 334–346 CrossRef CAS.
- M. Mousavi, A. Habibi-yangjeh and M. Abitorabi, Fabrication of novel magnetically separable nanocomposites using graphitic carbon nitride, silver phosphate and silver chloride and their applications in photocatalytic removal of different pollutants using visibl, J. Colloid Interface Sci., 2016, 480, 218–231 CrossRef CAS PubMed.
- H. Dong, An advanced Ag-based photocatalyst Ag2Ta4O11 with outstanding activity, Phys. Chem. Chem. Phys., 2014, 3–6 CAS.
- S. Brook, The absolute energy positions of conduction and valence bands of selected semiconducting minerals, Am. Mineral., 2000, 85, 543–556 CrossRef.
- L. Dia, H. Yanga, T. Xian and X. Chen, Enhanced Photocatalytic Degradation Activity of BiFeO3 Microspheres by Decoration with g-C3N4 Nanoparticles, Mater. Res., 2018, 21, 5 Search PubMed.
- S. Shenoy and K. Tarafder, Enhanced photocatalytic efficiency of layered CdS/CdSe heterostructures: Insights from first principles electronic structure calculations, J. Phys. Condens. Matter, 2020, 32, 8–14 CrossRef PubMed.
- J. P. Perdew, K. Burke and M. Ernzerhof, Generalized gradient approximation made simple, Phys. Rev. Lett., 1996, 77, 3865–3868 CrossRef CAS PubMed.
- M. K. Abdel-Sattar and M. Taha, Electronic structures and optoelectronic properties of ATiOPO4 (A = H, Li, Na, K, Rb, Cs, Fr, NH4, Ag) compounds and their applications in water splitting, CO2 reduction, and photo-degradation, Mater. Res. Express, 2020, 7, 045901 CrossRef CAS.
- M. I. Hussien, et al., Experimental and Theoretical (ab initio and DFT) Analysis of UV-Vis Spectra, Thermodynamic Functions and Non-linear Optical Properties of 2-Chloro-3,4-Dimethoxybenzaldehyde, J. Adv. Phys., 2015, 8, 1201–1229 Search PubMed.
- T. Rajamani, S. Muthu and M. Karabacak, Electronic absorption, vibrational spectra, nonlinear optical properties, NBO analysis and thermodynamic properties of N-(4-nitro-2-phenoxyphenyl) methanesulfonamide molecule by ab initio HF and density functional methods, Spectrochim. Acta, Part A, 2013, 108, 186–196 CrossRef CAS PubMed.
- M. Karabacak, L. Sinha, O. Prasad, Z. Cinar and M. Cinar, The spectroscopic (FT-Raman, FT-IR, UV and NMR), molecular electrostatic potential, polarizability and hyperpolarizability, NBO and HOMO-LUMO analysis of monomeric and dimeric structures of 4-chloro-3,5-dinitrobenzoic acid, Spectrochim. Acta, Part A, 2012, 93, 33–46 CrossRef CAS PubMed.
- H. H. AbdElAziz, M. Taha, W. M. A. El Rouby, M. H. Khedr and L. Saad, Evaluating the performance of Cs2PtI6−xBrx for photovoltaic and photocatalytic applications using first-principles study and SCAPS-1D simulation, Heliyon, 2022, 8, e10808 CrossRef CAS PubMed.
- H. Samir, M. Taha, S. I. El-Dek and A. H. Zaki, Electronic Structures and Electrical Properties of Cr2+-, Cu2+-, Ni2+-, and Zn2+-Doped Sodium Titanate Nanotubes, ACS Omega, 2022, 7, 27587–27601 CrossRef CAS PubMed.
- H. H. Kora, M. Taha, A. Abdelwahab, A. A. Farghali and S. I. El-Dek, Effect of pressure on the geometric, electronic structure, elastic, and optical properties of the normal spinel MgFe2O4: A first-principles study, Mater. Res. Express, 2020, 7, 1–11 Search PubMed.
- H. H. Kora, M. Taha, A. A. Farghali and S. I. El-Dek, First-Principles Study of the Geometric and Electronic Structures and Optical Properties of Vacancy Magnesium Ferrite, Metall. Mater. Trans. A, 2020, 51, 5432–5443 CrossRef CAS.
- M. N. H. Liton, et al., Electronic, mechanical, optical and photocatalytic properties of perovskite RbSr2Nb3O10 compound, J. Alloys Compd., 2021, 867, 159077 CrossRef CAS.
- A. Chakraborty, M. N. H. Liton, M. S. I. Sarker, M. M. Rahman and M. K. R. Khan, A comprehensive DFT evaluation of catalytic and optoelectronic properties of BaTiO3 polymorphs, Phys. B, 2023, 648, 414418 CrossRef CAS.
|
This journal is © The Royal Society of Chemistry 2023 |
Click here to see how this site uses Cookies. View our privacy policy here.