DOI:
10.1039/D3RA00168G
(Paper)
RSC Adv., 2023,
13, 7118-7128
Simultaneous voltammetric sensing of Zn2+, Cd2+, and Pb2+ using an electrodeposited Bi–Sb nanocomposite modified carbon paste electrode†
Received
9th January 2023
, Accepted 22nd February 2023
First published on 2nd March 2023
Abstract
A sensor for detecting Zn2+, Cd2+, and Pb2+ ions simultaneously based on the square wave anodic stripping response at a bismuth antimony (Bi–Sb) nanocomposite electrode was developed. The electrode was prepared in situ by electrodepositing bismuth and antimony on the surface of a carbon-paste electrode (CPE) while also reducing the analyte metal ions. The structure and performance of the Bi–Sb/CPE electrode were studied using scanning electron microscopy, X-ray diffraction, electrochemical impedance spectroscopy, and cyclic voltammetry. Operational conditions including the concentration of Sb and Bi, the type of electrolyte, pH, and preconcentration conditions were optimized. The linear ranges were determined to be 5–200 μg L−1 for Zn2+, 1–200 μg L−1 for Cd2+, and 1–150 μg L−1 for Pb2+ with the optimized parameters. The limits of detection were 1.46 μg L−1, 0.27 μg L−1, and 0.29 μg L−1 for Zn2+, Cd2+, and Pb2+, respectively. Furthermore, the Bi–Sb/CPE sensor is capable of selective determination of the target metals in the presence of the common cationic and anionic interfering species (Na+, K+, Ca2+, Mg2+, Fe3+, Mn2+, Co2+, Cl−, SO42− and HCO3−). Finally, the sensor was successfully applied to the simultaneous determination of Zn2+, Cd2+, and Pb2+ in a variety of real-world water samples.
1 Introduction
Heavy metals are highly persistent pollutants1 and their levels in the environment have risen dramatically in recent decades, possibly as a result of increased industrial and mining activities. The presence of heavy metals, like lead, cadmium and zinc, in water supplies poses a major health hazard.2 Lead and cadmium can cause a variety of cancers3 as well as serious brain and kidney damage.4 Excess zinc, despite its numerous essential biological roles, causes anosmia by rendering the liver or kidneys ineffective.5
Numerous analytical methods for determining trace amounts of hazardous heavy metal ions (HMIs) have been developed over the last few decades. These include atomic fluorescence spectrometry,6 atomic emission spectrometry, atomic absorption spectrometry,7 colorimetry,8,9 inductively coupled plasma atomic emission spectrometry, and inductively coupled plasma mass spectrometry.10,11 However, while these techniques are very selective and sensitive, they present a number of drawbacks in the detection of HMIs, including high cost, complex operating procedures, and poor field applicability.12
Electrochemical techniques, such as stripping voltammetry provide an attractive methodology for detecting HMIs, offering advantages for multiplexed detection in terms of accuracy, sensitivity, simplicity, low cost, and suitability for on-site detection.13–15 Anodic stripping voltammetry (ASV) is based on a two-step process. The first step is to pre-concentrate or electrodeposite the heavy metal at the electrode surface by reducing the metal ions. The second step is stripping, which involves re-oxidation of the investigated metals back to produce the ions in solution, according to the following equations:16,17
Mn+ + ne− → M (deposition step) |
M → Mn+ + ne− (stripping step) |
Due to ready alloy formation between mercury and HMIs, mercury electrodes have been employed to detect HMIs with good sensitivity and reproducibility. However, they can cause harm to human health and the environment.18 The first application of bismuth film electrodes (BiFEs) was reported in 2000,19 and since then, the entire topic has already attained a respectable position within the research activities focused on the development of mercury-free electrodes. The uses of BiFEs in stripping voltammetry and stripping chronopotentiometry of metal ions, particularly cadmium, lead, zinc, indium, and thallium, have drawn the interest.20,21 Antimony-based film electrodes (SbFEs) prepared in situ on the glassy carbon or carbon paste substrate electrode revealed low background currents and a favorable negative overvoltage for hydrogen evolution, similarly as encountered in the case of its bismuth and mercury analogues. The SbFEs also demonstrated a distinguishing characteristic linked to an extraordinarily low antimony oxidation signal, which allowed the detection of analytes with their oxidation potentials close to antimony's oxidation potential.22,23 Moreover, the presence of Sb in the electrode material can enhance the catalytic effect in determination of some HMIs such as Cd and Zn due to the formation of CdSb24 and ZnSb25 intermetallic compounds.
In recent years, nanoparticles, particularly metal nanoparticles, have shown various advantages in the field of electrochemical sensing.26,27 Because of their small dimensions, they greatly enhance electrode surface areas. Furthermore, metallic nanoparticles are able to improve the sensitivity of electrodes by providing fast electron transfer and increasing the mass-transport rate.28 Bismuth nanoparticles (BiNPs) have been widely employed to modify the surfaces of a variety of electrodes,29–32 while the application of antimony nanoparticles (SbNPs) is a new approach to modifying working electrodes for the detection of heavy metals.33–35 Accordingly, the development of a bimetallic Bi–Sb-modified electrode would combine the advantages of bismuth and antimony, improving sensitivity and affinity for several metals with low detection limits.
In this work, an electro-sensor was prepared in situ by electrodepositing bismuth and antimony on the surface of a carbon-paste electrode (Bi–Si/CPE). Then, its square wave anodic stripping voltammetry (SW-ASV) response was used to simultaneously determine Zn2+, Cd2+, and Pb2+ in water samples.
2 Experimental
2.1 Materials
Standard stock solutions (1 g L−1 Zn2+, Cd2+, Pb2+, Bi3+, and Sb3+) were supplied by Merck. Acetic acid (CH3COOH), sodium acetate (CH3COONa·3H2O), graphite powder, and Nujol oil were obtained from Sigma Aldrich. Freshly bi-distilled water was used. The real water samples were: tap water; Aquafina® bottled water; underground water from a well located in Shebin Elkom City, El-Menoufia Governorate, Egypt; and treated waste water from a treatment station located in Quesna City, El-Menoufia Governorate, Egypt.
2.2 Instruments
Epsilon-EC voltammetric analyzer device from BASi was used to conduct cyclic voltammetry (CV) and SW-ASV analyses. The three-electrode system consisted of a holder electrode with diameter 3.0 mm filled with carbon paste (graphite and Nujol oil), a silver/silver chloride (Ag/AgCl) reference electrode, and an auxiliary electrode made of platinum (Pt) wire. A Model Versa STAT 4 potentiostat (Princeton Applied Research, Oak Ridge, TN, USA) was used to conduct electrochemical impedance spectroscopy (EIS) experiments. EIS was fitted by the equivalent circuit modeling applying the ZSim ver. 3.20 software (EChem Software, Michigan, USA). Scanning electron microscopy (SEM) images and energy-dispersive X-ray (EDX) analysis were achieved using a Thermo Scientific Quattro (Netherlands). The X-ray diffraction (XRD) patterns were carried out by Shimadzu 6000, the measurement conditions used were Cu target radiation, voltage 40.0 kV, and current 30.0 mA at room temperature.
2.3 Modified-carbon-paste electrode preparation
In a small mortar, 5 g graphite powder (1–2 μm) and 1.8 mL Nujol oil (d = 0.84 g mL−1) were thoroughly milled to make a homogeneous carbon paste. Carbon paste was then packed into the electrode body and its surface was gently smoothed until shiny appearance. The bismuth-antimony (Bi–Sb) nanocomposite was electrodeposited on the CPE surface from a solution of acetate buffer (pH 5.6) containing 100 μg L−1 of each of Bi3+ and Sb3+ ions only (ex situ) or together with the target metal ions (Zn2+, Cd2+, and Pb2+) (in situ) as shown in Fig. 1. Electrodeposition was carried out under mild stirring conditions (at 400 rpm) at a deposition potential (dp E) = −1.55 V and deposition time (dp t) = 180 s. The modified Bi–Sb/CPE was washed by double distilled water and then keep to dry at room temperature prior to use. All experimental work was employed in room temperature.
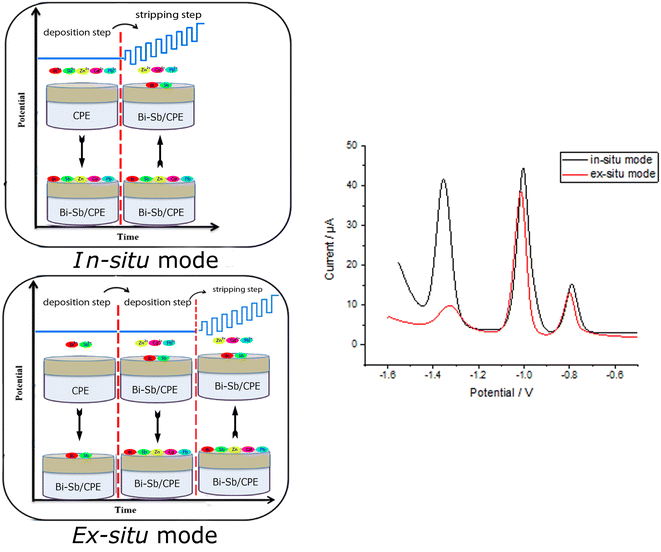 |
| Fig. 1 Schematic representation of in situ and ex situ methods used for preparation of the Bi–Sb/CPE modified electrode and for determination of Zn2+, Cd2+, and Pb2+ metal ions. | |
2.4 Procedure for the determination of Zn2+, Cd2+, and Pb2+
The prepared CPE was placed in a solution of acetate buffer (pH 5.6). To the buffer solution, standard HMI solutions (Zn2+, Cd2+, Pb2+, Bi3+, and Sb3+) were added. SW-ASV was performed in two steps. Firstly, metal deposition at deposition potential (dp E) = −1.55 V and deposition time (dp t) = 180 s, followed by stripping performed using SWV in a potential range from −1.55 to −0.5 V at a frequency of 15 Hz, potential steps of 6 mV, and a pulse amplitude of 30 mV.
3 Results and discussion
3.1 SEM, EDX and XRD characterization of different electrodes
SEM was used to characterize the morphologies of the electrodes. The SEM micrographs show the morphologies of the deposited SbNPs and BiNPs on the surface of the carbon paste at different magnifications to clarify the details of the surface (Fig. 2a–d). Furthermore, EDX analysis was used to identify the elements and determine their relative abundances in the examined samples. The SEM images of the bare carbon-paste electrode reveal a microstructure featuring layers of irregular isolated flakes of graphite powder. The SEM micrographs of Bi–Sb/CPE show that Sb–Bi nano-composites are well embedded within the carbon-paste matrix, and the electrode surface shows increased roughness and porosity, consequently increasing the electroactive surface area.
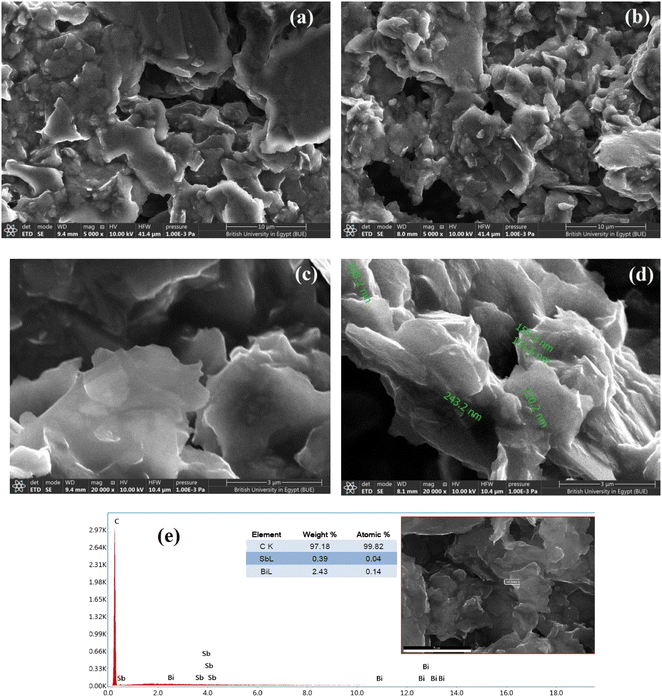 |
| Fig. 2 SEM image of bare CPE (a, c) with magnification degrees = 5000× and 2000×, respectively, modified Bi–Sb/CPE (b, d) with the same two magnification degrees and EDX of the modified electrode surface (e). | |
The surface of Bi–Sb/CPE is a sheet material, as evidenced by EDX analysis, and the proportions of elements therein are shown Fig. 2e. The antimony and bismuth contents in the composite obtained by in situ electrodeposition were also determined by the SEM-EDX technique, and elemental ratios of 0.39% and 2.43% were obtained for antimony and bismuth, respectively.
Surface of both bare CPE and modified Bi–Sb/CPE were tested by XRD patterns as shown in Fig. 3. Graphite pattern appeared at 26°,36 due to the presence of the oxygenated groups on carbon sheets (Fig. 3a). Fig. 2b exhibited the XRD diffraction patterns of the surface of the modified Bi–Sb/CPE after the electrodeposition of Bi and Sb nanocomposite on the graphite paste. As shown in Fig. 3b, Bi nanoparticles appeared at 23.84°,37 and Sb nanoparticles were recorded at 21.64°, and 36.1°,38 while the graphite patterns seemed weak pattern at 26.97° with a small shift compared to the graphite electrode. This behavior confirmed the deposition of Bi and Sb nanocomposite on the graphite surface.
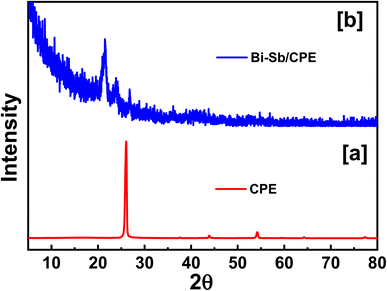 |
| Fig. 3 XRD patterns of electrode surfaces of bare CPE and as-prepared Bi–Sb/CPE. | |
3.2 Electrochemical behavior and effective surface area of the Sb–Bi/CPE electrode
The CV responses of the bare CPE and Bi–Sb/CPE were investigated (Fig. 4) using a 1.0 mM potassium ferrocyanide (K4[Fe(CN)6]) solution containing 0.1 M KCl. Higher oxidation and reduction peak currents are observed for the Bi–Sb/CPE than for the bare CPE, indicating better electrochemical catalytic activity and improved electron transport at the modified electrode surface.
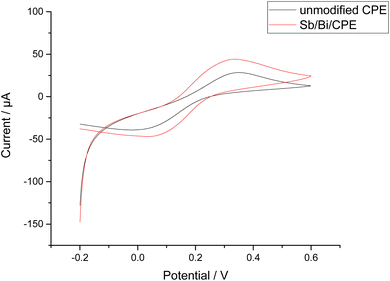 |
| Fig. 4 Cyclic voltammetry (CV) results for 1.0 mM K4[Fe(CN)6] in 0.1 M KCl using a scan rate of 0.1 V s−1 at the bare CPE and Bi–Sb/CPE. | |
We can calculate the active surface areas of the bare CPE and Bi–Sb/CPE using the Randles–Sevcik equation (eqn (1)):
|
Ip = (2.69 × 105)n3/2AD1/2Cv1/2
| (1) |
where
Ip corresponds to the current (A),
n corresponds to the number of electrons transferred during the oxidation reduction reaction (
n = 1),
A corresponds to the active surface area of the electrode (cm
2),
D corresponds to the K
4[Fe(CN)
6] diffusion coefficient (7.6 × 10
−6 cm
2 s
−1),
C corresponds to the K
4[Fe(CN)
6] concentration (mol L
−1), and
v corresponds to the scan rate (V s
−1).
Fig. 5a and b show the effects of scan rate on the peak currents for K
4[Fe(CN)
6] at the bare and modified electrodes. The slope of the relationship between the square root of the scan rate and peak current was utilized to calculate active surface area. The active surface areas of the bare CPE and the Bi–Sb/CPE are 0.052 and 0.168 cm
2, respectively. The improvement of the active surface area supports the results obtained by SW-ASV of the target metal ions on the surfaces of the bare and modified electrodes.
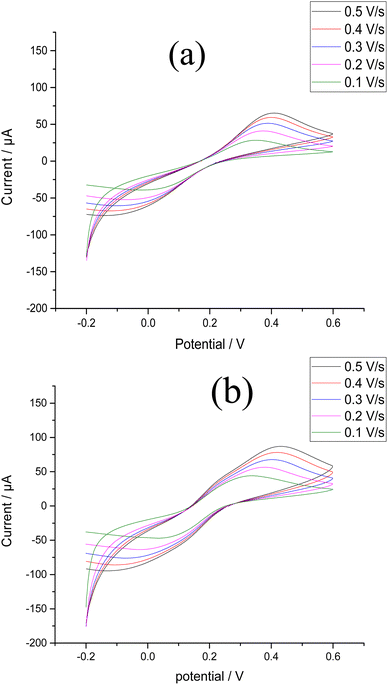 |
| Fig. 5 CV results for 1.0 mM K4[Fe(CN)6] in 0.1 M KCl using scan rates of 0.1–0.5 V s−1 at (a) the bare CPE (b) the Bi–Sb/CPE. | |
3.3 EIS
The EIS technique is commonly used to study the barrier properties of electrode surfaces. The obtained curve in our study using EIS techniques called the Nyquist plot. In the high-frequency region, it is semicircle-shaped and corresponds to the electron-transfer process, while in the low-frequency region; it is linear and corresponds to the diffusion process. The semicircle diameter can be used to estimate the charge-transfer resistance (Rct), which reflects the facility of the electrode reaction. Fig. 6 shows that semicircle with a greater diameter is produced at the bare CPE, and the Rct value for the [Fe(CN)6]3−/[Fe(CN)6]4− oxidation reduction process was determined to be 11.446 kΩ. However, the diameter of the semicircle is considerably decreased for Bi–Sb/CPE, and Rct was determined to be 0.9739 kΩ. This large decrease is because Bi and SbNPs greatly improve the electrical-transport properties, which facilitates the oxidation reduction process of the [Fe(CN)6]3−/[Fe(CN)6]4− system. The results from impedance study are consistent with those obtained from CV measurements, indicating that Bi and SbNPs were successfully electrodeposited onto the bare CPE. The standard constants of heterogeneous rates for the bare CPE and Bi–Sb/CPE were calculated according to the eqn (2):where k° corresponds to the standard heterogeneous electron-transfer rate constant (cm s−1), T corresponds to the thermodynamic temperature (298.15 K), R corresponds to the universal gas constant (8.314 J K−1 mol−1), F corresponds to the Faraday constant (96
485C mol−1), Rct corresponds to the charge transfer resistance (Ω), A corresponds to the surface area of the electrode (cm2), and C corresponds to the [Fe(CN)6]3−/4− solution concentration (1.0 mM). The k° value is an indication of the kinetic facility of a redox pair. When a system has a high k° value, it takes a shorter time to reach equilibrium than one with a low k° value, resulting in a faster equilibrium. The k° constants were calculated for bare CPE and Bi–Sb/CPE to be 4.47 × 10−9 and 1.63 × 10−8 cm s−1, respectively. As a result, the Bi–Sb/CPE has a higher k° and a lower Rct than the unmodified electrode, implying a faster electron-transfer process. Moreover, the EIS for Bi–Sb/CPE was fitted by the equivalent circuit modeling of (R(C(RW))) as shown in Fig. 6 (inset). This model was built using series components; the first one is the bulk solution resistance, Rs, second one the parallel combination of the double layer capacitance, Cdl, charge transfer resistor, Rct, and the Warburg impedance, W. Rct at the electrode surface is equal to the semicircle diameter, which can be used to describe the interface properties of the electrode.
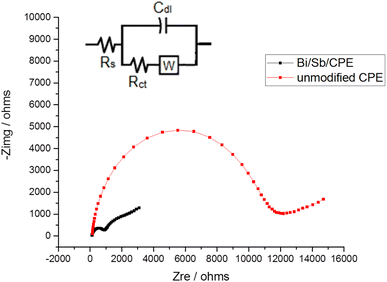 |
| Fig. 6 Nyquist plots for bare CPE and Bi–Sb/CPE in the presence of 1.0 mM K4[Fe(CN)6] and 0.1 M KCl at frequencies of 10 kHz to 0.1 Hz. Inset is the equivalent circuit. | |
3.4 SW-ASV of Zn2+, Cd2+, and Pb2+
The simultaneous determination of Zn2+, Cd2+, and Pb2+ ions was performed at both the bare CPE and modified Bi–Sb/CPE. The SW-ASV results for 100 μg L−1 each of Zn2+, Cd2+, and Pb2+ in acetate buffer solution (ABS) pH 5.6 are shown in Fig. 7. The stripping peak current responses of Zn2+, Cd2+, and Pb2+ ions at the bare CPE are relatively small and appear at 1.316, 1.008, and 0.796 V, respectively. At the modified Bi–Sb/CPE, the peak separation is increased and the three peaks appear at −1.352, −1.008, and −0.792 V for Zn2+, Cd2+, and Pb2+, respectively. The peak current values in case of the modified Bi–Sb/CPE were 9.98, 2.19, and 2.02 times much enhanced over that of the bare CPE for Zn2+, Cd2+, and Pb2+, respectively. The increased peak current values could be ascribed to the Bi–Sb nanocomposite, which increases the electroactive surface area and electrical conductivity.
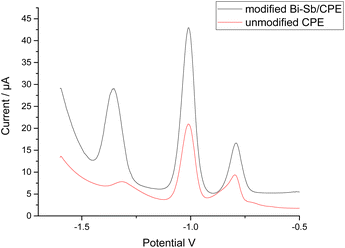 |
| Fig. 7 Square wave anodic stripping voltammetry (SW-ASV) results for 100 μg L−1 Zn2+, Cd2+, and Pb2+ at the bare CPE and Bi–Sb/CPE in ABS pH 5.6. (dp E) was −1.55 V; (dp t) was 180 s; frequency was 15 Hz; potential step was 6 mV; and pulse amplitude was 30 mV. | |
3.5 Optimization of experimental conditions
Several key factors were considered in order to achieve the best experimental conditions for SW-ASV measurement of Zn2+, Cd2+, and Pb2+. These parameters were the method of electrodeposition of Bi–Sb nanocomposite, electrolyte type, pH, deposition potential, and deposition time. The optimum conditions were determined based on the values of the Zn2+, Cd2+, and Pb2+ stripping peak currents at the same concentration (100 μg L−1) for each metal ion.
3.5.1 Method of electrodeposition of Bi–Sb nanocomposite. Zn2+, Cd2+, and Pb2+ were determined using Bi–Sb nanocomposite electrodeposited on the surface of a CPE in both in situ and ex situ modes (Fig. 8a). For the three target metal ions, the in situ electrodeposition is clearly preferable. At the Bi–Sb/CPE prepared in situ, the peak current values are 6.21, 1.15, and 1.13 times much enhanced than those obtained by the ex situ prepared electrode for Zn2+, Cd2+, and Pb2+, respectively. Hence, for the preparation of our modified electrode, the in situ mode was chosen for the following studies.
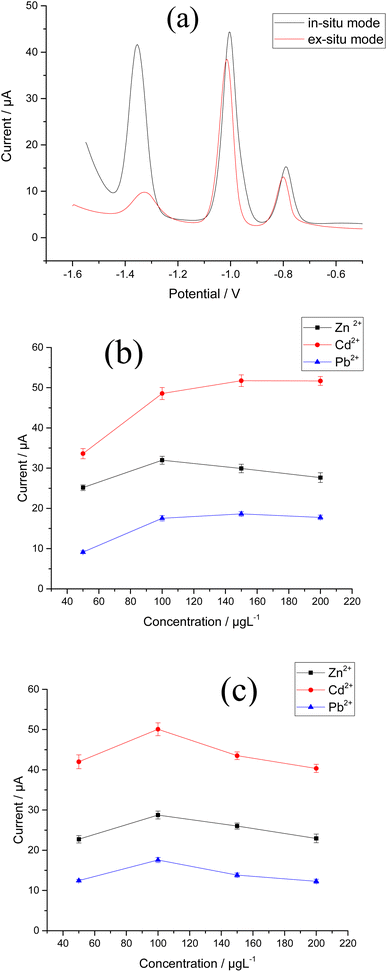 |
| Fig. 8 Effect of method of electrodeposition of Bi–Sb nanocomposite (a) in situ and ex situ modes (b) Bi3+ ion concentration (c) Sb3+ ion concentration on the stripping peak currents of 100 μg L−1 Zn2+, Cd2+ and Pb2+ in ABS pH 5.6, dp E = −1.55 V and dp t = 180 s at the Bi–Sb/CPE. | |
3.5.2 Effect of concentration of Bi3+ and Sb3+ ions. In two separate experiments, the concentrations of either Sb3+ or Bi3+ were varied in the range 50–200 μg L−1 in the presence of 100 μg L−1 of the other metal ion. It can be seen from Fig. 8b and c that the stripping peak currents of the three determined metals are maximal when using concentration of 100 μg L−1 for either Bi3+ or Sb3+.
3.5.3 Effect of electrolyte type and pH. The stripping voltammograms for 100 μg L−1 Zn2+, Cd2+, and Pb2+ were recorded at the modified Sb–Bi CPE using 0.1 M HClO4 (pH 1), 0.01 M HCl (pH 2), acetate buffer (pH 5.6), and phosphate buffer (pH 7) as supporting electrolytes. Fig. 9a shows the current responses for Zn2+, Cd2+, and Pb2+ in the four different electrolytes. Peaks corresponding to the three target metal ions are observed for all studied electrolytes, where remarkable improvements in peak current are observed for pH 5.6. Thus, the influence of pH on the stripping peak current was investigated in ABS with a pH range between 3.6 and 5.6, and the results are illustrated in Fig. 9b. The greatest Zn2+, Cd2+, and Pb2+ stripping peak currents are observed at pH 5.6.
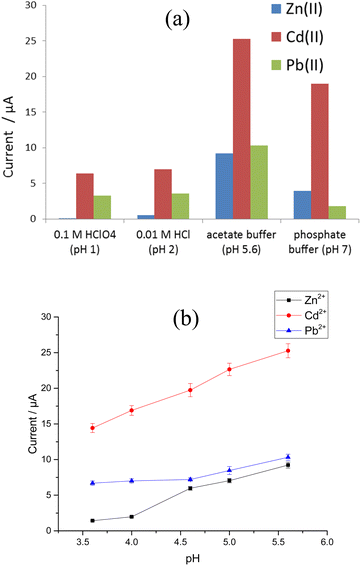 |
| Fig. 9 Effect of (a) type of electrolyte and (b) pH on the stripping peak currents for 100 μg L−1 Zn2+, Cd2+, and Pb2+ at the Bi–Sb/CPE. | |
3.5.4 Parameters affecting SW-ASV response. Instrumental factors such as frequency, potential step, and amplitude that affect SW-ASV response were studied (Fig. S1a–c†). The frequency was investigated between 5 and 35 Hz, and 15 Hz shows the best performance. Various step-potentials between 2 and 10 mV were tested, indicating that 6 mV is optimal. The square wave amplitude was investigated between 5 and 40 mV. The optimal value was found to be 30 mV. Therefore, the optimal instrumental conditions of frequency = 15 Hz, step-potential = 6 mV, and amplitude = 30 mV were used in the rest of the study.
3.5.5 Effect of accumulation conditions. The influence of deposition potential on Zn2+, Cd2+, and Pb2+ SW-ASV responses was investigated in the potential range −1.5 to −1.7 V. When the deposition potential shifts from −1.5 to −1.55 V, the stripping peak currents for the three metals increase (Fig. 10a). However, when the accumulation potential became more negative, the stripping peak currents decrease. The decrease in the current of the peaks can be attributed to competition with H2 formation. As a result, in order to achieve the highest sensitivity, −1.55 V was selected as the best deposition potential.
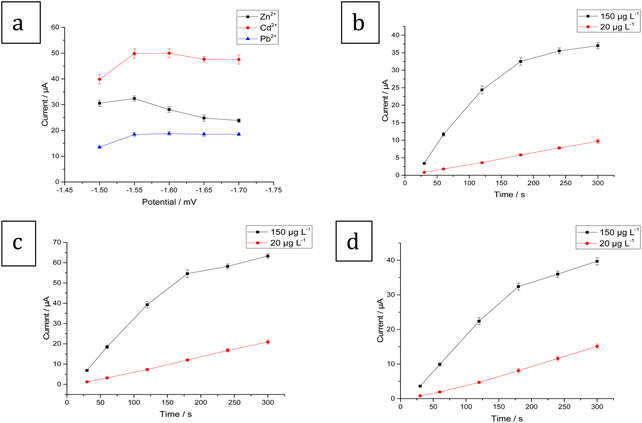 |
| Fig. 10 (a) Effect of accumulation potential on the stripping peak currents for 100 μg L−1 Zn2+, Cd2+, and Pb2+. Effect of accumulation time (30, 60, 120, 180, 240, and 300 s) on the stripping peak currents for 150 and 20 μg L−1 (b) Zn2+, (c) Cd2+, and (d) Pb2+ in ABS pH 5.6 and dp E = −1.55 V at the Bi–Sb/CPE. | |
SW-ASVs of 20 and 150 μg L−1 of Zn2+, Cd2+, and Pb2+ were obtained under the optimal conditions after preconcentration by electrodeposition at −1.55 V for increasing preconcentration times (30 to 300 s). The peak currents for 20 μg L−1 Zn2+, Cd2+, and Pb2+ increase in a linear manner with preconcentration time over the studied period, as illustrated in Fig. 10b–d. The peak currents for 150 μg L−1 Zn2+, Cd2+, and Pb2+ show a linear rise up to 180 s. At longer preconcentration periods, the Bi–Sb/CPE surface is saturated by the deposited metals and therefore the currents of the peaks plateau. Thus, 180 s was chosen for further experiments to achieve wider concentration ranges of determination.
3.6 Method validation
3.6.1 Linear range and detection limit. Under the optimal experimental conditions, Zn2+, Cd2+, and Pb2+ were determined individually and simultaneously with the Bi–Sb/CPE using SW-ASV. The SW-ASV responses of Zn2+ at various concentrations are shown in Fig. 11a. In the range between 5 and 200 μg L−1, well-defined peaks proportional to the concentration of Zn2+ are observed. The sensitivity value of 0.4189 μA μg L−1 (R2 = 0.997) was obtained. The limit of detection (LOD) was calculated to be 1.46 μg L−1. The SW-ASV responses of the Sb–Bi/CPE toward Cd2+ over the concentration range between 1 and 200 μg L−1 are illustrated in Fig. 11b. The Cd2+ concentration and peak current exhibit a good linear relationship. The sensitivity value is 0.4523 μA L μg−1 (R2 = 0.995) with a LOD value of 0.27 μg L−1. Fig. 11c shows that the current for the Pb2+ peak rises in a linear manner with concentration in the range 1–150 μg L−1. The sensitivity value is 0.3403 μA μg L−1 (R2 = 0.994) with a LOD of 0.29 μg L−1. Therefore, Bi–Sb/CPE shows great promise as a platform for determining HMIs.
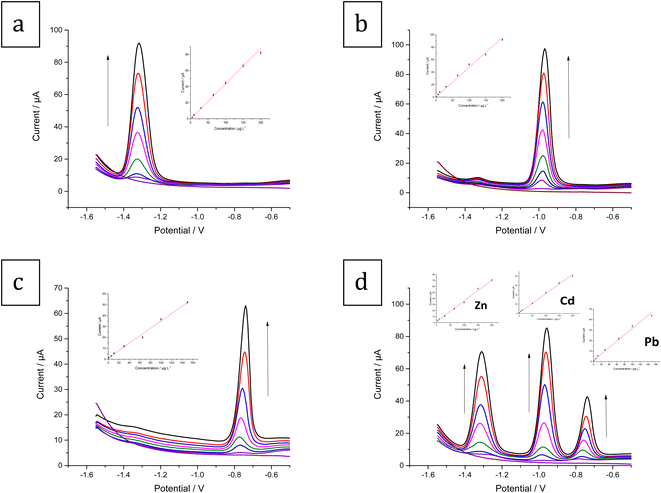 |
| Fig. 11 SW-ASV results and calibration for (a) Zn2+ (0 (blank), 5, 10, 30, 65, 100, 150, 200 μg L−1) (b) Cd2+ (0 (blank), 1, 6, 11, 30, 65, 100, 150, 200 μg L−1) (c) Pb2+ (0 (blank), 1, 6, 11, 30, 65, 100, 150 μg L−1) (d) the three metal ions simultaneously: Zn2+ (0 (blank), 5, 10, 30, 65, 100, 150, 200 μg L−1), Cd2+ (0 (blank), 1, 6, 11, 50, 100, 150, 200 μg L−1), and Pb2+ (0 (blank), 1, 6, 11, 30, 65, 100, 150 μg L−1) in ABS pH 5.6, dp E = −1.55 V and dp t = 180 s at the Bi–Sb/CPE. | |
Fig. 11d shows the SW-ASV results for the measurement of Zn2+, Cd2+, and Pb2+ simultaneously at increasing concentrations using Bi–Sb/CPE under the optimal experimental conditions. The developed electrode was successful in determining the three target metal ions simultaneously. The voltammetric peaks are sufficiently separated, and thus simultaneous and selective detection with the Bi–Sb/CPE sensor is possible.
The calibration curve equations for the individual and simultaneous detection of the target metal ions are provided in Table 1. Comparison of the slopes of the calibration curves for each metal ion in the absence and presence of the other two target metal ions shows that Bi–Sb/CPE can be utilized for the voltammetric detection of Zn2+, Cd2+, and Pb2+ individually or simultaneously without any interference between the three analytes. Table 2 compares the data obtained using the proposed Bi–Sb/CPE and other different modified electrodes reported previously in the literature. As shown in Table 2, our method provides wide detection ranges and the lowest LOD values for the investigated metal ions.
Table 1 Analytical parameters for Zn2+, Cd2+, and Pb2+ detection at the Bi–Sb/CPE
|
Metal ion |
Regression equation: [i (μA)/C (μg L−1)] |
R2 |
LOD (μg L−1) |
LOQ (μg L−1) |
Individual determination |
Zn2+ |
i (μA) = 0.4189 ± 0.0142C (μg L−1) + 1.0278 ± 0.2044 |
0.997 |
1.46 |
4.88 |
Cd2+ |
i (μA) = 0.4523 ± 0.0117C (μg L−1) + 2.8304 ± 0.0411 |
0.995 |
0.27 |
0.91 |
Pb2+ |
i (μA) = 0.3403 ± 0.0071C (μg L−1) + 1.0404 ± 0.0333 |
0.994 |
0.29 |
0.98 |
Simultaneous determination |
Zn2+ |
i (μA) = 0.3505 ± 0.0112C (μg L−1) + 1.0457 ± 0.1724 |
0.995 |
1.48 |
4.92 |
Cd2+ |
i (μA) = 0.405 ± 0.0145C (μg L−1) + 2.1456 ± 0.0381 |
0.997 |
0.28 |
0.94 |
Pb2+ |
i (μA) = 0.2933 ± 0.0129C (μg L−1) + 1.9331 ± 0.0282 |
0.992 |
0.29 |
0.96 |
Table 2 Comparison between the prepared electrode and other related modified electrodes reported in the literature for the detection of Zn2+, Cd2+, and Pb2+a
Electrode material |
Method |
tdep (s) |
Linear range (μg L−1) |
LOD (μg L−1) |
Ref. |
Zn2+ |
Cd2+ |
Pb2+ |
Zn2+ |
Cd2+ |
Pb2+ |
Sb-BDD: antimony nanoparticle modified boron doped diamond. D/G: diamond/graphite. AgNPs: Ag nanoparticles. G/PANI: graphene/polyaniline. SPCNTE: screen-printed carbon nanotubes electrodes. SIA-ASV: sequential injection analysis-anodic stripping voltammetry. poly(p-ABSA): poly(p-aminobenzene sulfonic acid). |
GCE-GO-BiNPs |
SWASV |
120 |
— |
11.24–158.48 |
20.72–292.15 |
— |
2.92 |
6.22 |
29 |
GC/rGO-SbNPs |
SWASV |
120 |
— |
11.24–505.8 |
20.72–932.4 |
— |
5.09 |
3.42 |
33 |
Cr-CPE |
SWASV |
100 |
80–800 |
10–800 |
10–800 |
25 |
3 |
3 |
39 |
D/G nano-platelets film electrode |
DPASV |
270 |
10–250 |
10–250 |
25–250 |
9.12 |
2.45 |
3.05 |
40 |
AgNP/Bi/Nafion |
SWASV |
360 |
5–400 |
0.5–400 |
0.1–500 |
5.0 |
0.5 |
0.1 |
41 |
Nafion/G/PANI nano-composite |
SWASV |
240 |
1–300 |
1–300 |
1–300 |
1.0 |
0.1 |
0.1 |
42 |
Bi-SPCNTE |
SIA-ASV |
180 |
12–100 |
2–100 |
2–100 |
11 |
0.8 |
0.2 |
43 |
Bi/poly(p-ABSA) |
DPASV |
240 |
1–110 |
1–110 |
1–130 |
0.62 |
0.63 |
0.80 |
44 |
Bi–Sb/CPE |
SWASV |
180 |
5–200 |
1–200 |
1–150 |
1.46 |
0.27 |
0.29 |
This work |
3.6.2 Precision and accuracy. The precision (RSD) and accuracy (% recovery) for the proposed SW-ASV method for determining Zn2+, Cd2+, and Pb2+ were evaluated by analyzing several standard solutions of the three metal ions. The RSD calculation was based on five replicate measurements using five freshly reproduced surfaces of the sensor, and the % recovery was obtained by applying the calibration curve method. Table of results (Table S2†) show that RSD values didn't exceed 4.13 and % recovery lied between 98.2 and 101.2%. Moreover, the peak current magnitude of 100 μg L−1 of each of Zn2+, Cd2+, and Pb2+ on the same surface of the Bi–Sb/CPE sensor was tested daily over a week. Mean recoveries of 96.11 ± 3.37, 97.53 ± 2.78 and 97.19 ± 2.55 (n = 7) for Zn2+, Cd2+, and Pb2+, respectively, were achieved. These measurements confirmed suitable repeatability, reproducibility, re-usability, and long-term stability of the modified Bi–Sb/CPE sensor for electroanalytical sensing of the target metal ions.
3.6.3 Interference effect. The effects of possible interfering cations and anions present in water samples were studied before applying the modified electrode to real environmental samples analysis. Interferences from major cations (Na+, K+, Ca2+, and Mg2+), some trace metal ions (Mn2+, Fe3+, and Co2+) and major anions (Cl−, SO42− and HCO3−) that are typically present in real water samples on the current response of 100 μg L−1 of each of Zn2+, Cd2+, and Pb2+ were assessed (Table 3). The interference tolerance level for each interfering species is defined as the greatest concentration of interfering species that results in a ±5% variation in peak current. The obtained results demonstrate that the Bi–Sb/CPE can be considered a good sensor for detecting Zn2+, Cd2+, and Pb2+ in real water samples.
Table 3 Effect of various interfering species on the peak currents of 100 μg L−1 of each of Zn2+, Cd2+, and Pb2+
Interfering ion |
Tolerance levela (mg L−1) |
Peak current deviation ±5%. |
Na+ |
50 |
K+ |
60 |
Ca2+ |
40 |
Mg2+ |
40 |
Fe3+ |
3 |
Mn2+ |
1 |
Co2+ |
1 |
Cl− |
80 |
SO42− |
50 |
HCO3− |
120 |
3.7 Application to real water samples
The electrochemical performance of the Bi–Sb/CPE sensor was evaluated with a variety of real water samples, including tap water, bottled water, underground water, and treated waste water. Since Zn2+, Cd2+, and Pb2+ were not found in the first three water samples, our study was continued by spiking the samples with three different concentrations (15, 75, and 150 μg L−1) of each metal ion. The three metal ions were all found in the treated waste water and were measured using the calibration curve method without spiking. Results are tabulated in Table 4 and it shows that RSD values didn't exceed 4.54 and % recovery lied between 95.2 and 103.7%. The results demonstrate that the developed Bi–Sb/CPE sensor exhibits good accuracy for Zn2+, Cd2+, and Pb2+ detection in environmental water samples.
Table 4 Detection of Zn2+, Cd2+, and Pb2+ in different samples of real water using the proposed method
Type of water |
Metal ions |
Spiked (μg L−1) |
Found (μg L−1) |
% recovery |
RSD |
Tap water |
Zn |
15 |
14.60 |
97.3 |
3.55 |
75 |
71.85 |
95.8 |
4.23 |
150 |
147.15 |
98.1 |
2.34 |
Cd |
15 |
14.43 |
96.2 |
1.26 |
75 |
73.58 |
98.1 |
2.47 |
150 |
145.20 |
96.8 |
2.75 |
Pb |
15 |
14.46 |
96.4 |
4.34 |
75 |
71.78 |
95.7 |
4.73 |
150 |
147.75 |
98.5 |
3.34 |
Bottled water |
Zn |
15 |
14.58 |
97.2 |
2.44 |
75 |
76.73 |
102.3 |
4.27 |
150 |
147.15 |
98.1 |
2.93 |
Cd |
15 |
15.32 |
102.1 |
3.38 |
75 |
76.05 |
101.4 |
1.32 |
150 |
155.25 |
103.5 |
2.43 |
Pb |
15 |
15.56 |
103.7 |
4.29 |
75 |
76.35 |
101.8 |
3.42 |
150 |
154.80 |
103.2 |
3.38 |
Underground water |
Zn |
15 |
14.36 |
95.7 |
3.47 |
75 |
72.60 |
96.8 |
1.66 |
150 |
146.40 |
97.6 |
2.64 |
Cd |
15 |
14.13 |
94.2 |
4.32 |
75 |
73.73 |
98.3 |
2.97 |
150 |
146.25 |
97.5 |
3.54 |
Pb |
15 |
14.45 |
96.3 |
2.38 |
75 |
71.40 |
95.2 |
4.54 |
150 |
147.30 |
98.2 |
2.87 |
Treated waste water |
Zn |
— |
6.11 |
— |
2.44 |
Cd |
— |
4.18 |
— |
3.69 |
Pb |
— |
5.25 |
— |
2.95 |
4 Conclusions
A simple and efficient CPE modified with a Bi–Sb nanocomposite was successfully fabricated for stripping analysis of Zn2+, Cd2+, and Pb2+ metal ions. The suggested sensor demonstrates good analytical performance under optimal conditions, with LOD values of 1.46, 0.27, and 0.29 μg L−1 for simultaneous determination of Zn2+, Cd2+, and Pb2+, respectively. The described method can be effectively utilized to determine the three metal ions in real water samples without significant interference. Future projection and scope will be trying to apply the developed Bi–Sb/CPE sensor for trace determination of some biologically-active organic compounds such as drugs and pesticides.
Author contributions
E. A. Shalaby: investigation, data curation, methodology. A. M. Beltagi: conceptualization, supervision, methodology, writing – review & editing. A. A. Hathoot: supervision, methodology. M. Abdel Azzem: conceptualization, supervision, methodology, writing – review & editing.
Conflicts of interest
There are no conflicts to declare.
Acknowledgements
Authors thank the Alexander von Humboldt Foundation as it presented some electrochemical instruments to the authors as a research gift.
References
- Y. Wang, Y. Peng, Y. Chen, K. Du, Y. Li and X. He, Anal. Methods, 2016, 8, 1935–1941 RSC.
- J. A. Buledi, S. Amin, S. I. Haider, M. I. Bhanger and A. R. Solangi, Environ. Sci. Pollut. Res., 2020, 1–9 Search PubMed.
- J. Barton, M. B. G. García, D. H. Santos, P. Fanjul-Bolado, A. Ribotti, M. McCaul, D. Diamond and P. Magni, Microchim. Acta, 2016, 183, 503–517 CrossRef CAS.
- J. Dong, Q. Fang, H. He, Y. Zhang, J. Xu and Y. Sun, Microchim. Acta, 2015, 182, 653–659 CrossRef CAS.
- N. Wang, E. Kanhere, A. G. P. Kottapalli, J. Miao and M. S. Triantafyllou, Microchim. Acta, 2017, 184, 3007–3015 CrossRef CAS.
- J. L. Gómez-Ariza, F. Lorenzo and T. García-Barrera, Anal. Bioanal. Chem., 2005, 382, 485–492 CrossRef PubMed.
- P. Pohl, TrAC, Trends Anal. Chem., 2009, 28, 117–128 CrossRef CAS.
- F. Chai, C. Wang, T. Wang, Z. Ma and Z. Su, Nanotechnology, 2009, 21, 025501 CrossRef PubMed.
- W. Chansuvarn, T. Tuntulani and A. Imyim, TrAC, Trends Anal. Chem., 2015, 65, 83–96 CrossRef CAS.
- B. Song, G. Zeng, J. Gong, J. Liang, P. Xu, Z. Liu, Y. Zhang, C. Zhang, M. Cheng and Y. Liu, Environ. Int., 2017, 105, 43–55 CrossRef CAS PubMed.
- L. Balcaen, E. Bolea-Fernandez, M. Resano and F. Vanhaecke, Anal. Chim. Acta, 2015, 894, 7–19 CrossRef CAS PubMed.
- Z. Zeng, J. Li, H. M. Hugel, G. Xu and P. J. Marriott, TrAC, Trends Anal. Chem., 2014, 53, 150–166 CrossRef CAS.
- L. Cui, J. Wu and H. Ju, Biosens. Bioelectron., 2015, 63, 276–286 CrossRef CAS PubMed.
- M. F. Altahan, A. G. Ali, A. A. Hathoot and M. Abdel-Azzem, J. Electrochem. Soc., 2020, 167, 127510 CrossRef CAS.
- K. M. Hassan, S. E. Gaber, M. F. Altahan and M. A. Azzem, Electroanalysis, 2018, 30, 1155–1162 CrossRef CAS.
- B. Bansod, T. Kumar, R. Thakur, S. Rana and I. Singh, Biosens. Bioelectron., 2017, 94, 443–455 CrossRef CAS PubMed.
- J. Wang, Analytical Electrochemistry, John Wiley & Sons, Inc., Hoboken, New Jersey, USA, 3rd edn, 2006 Search PubMed.
- K. Wu, S. Hu, J. Fei and W. Bai, Anal. Chim. Acta, 2003, 489, 215–221 CrossRef CAS.
- J. Wang, J. Lu, S. B. Hocevar, P. A. Farias and B. Ogorevc, Anal. Chem., 2000, 72, 3218–3222 CrossRef CAS PubMed.
- I. Švancara and K. Vytřas, Chem. Listy, 2006, 100 Search PubMed.
- I. Švancara, C. Prior, S. B. Hočevar and J. Wang, Electroanalysis, 2010, 22, 1405–1420 CrossRef.
- S. B. Hocevar, I. Švancara, B. Ogorevc and K. Vytřas, Anal. Chem., 2007, 79, 8639–8643 CrossRef CAS PubMed.
- E. Tesarova, L. Baldrianova, S. B. Hocevar, I. Svancara, K. Vytras and B. Ogorevc, Electrochim. Acta, 2009, 54, 1506–1510 CrossRef CAS.
- A. M. Ashrafi and K. Vytřas, Int. J. Electrochem. Sci., 2013, 8, 2095–2103 CAS.
- B. DeWitt and H. Seltz, J. Am. Chem. Soc., 1939, 61, 3170–3173 CrossRef CAS.
- K. M. Hassan, G. M. Elhaddad and M. AbdelAzzem, Microchim. Acta, 2019, 186, 1–10 CrossRef CAS PubMed.
- K. M. Hassan, S. E. Gaber, M. F. Altahan and M. A. Azzem, Sens. Bio-Sens. Res., 2020, 29, 100369 CrossRef.
- S. Sawan, R. Maalouf, A. Errachid and N. Jaffrezic-Renault, TrAC, Trends Anal. Chem., 2020, 116014 CrossRef CAS.
- E. H. Bindewald, A. F. Schibelbain, M. A. Papi, E. G. Neiva, A. J. Zarbin, M. F. Bergamini and L. H. Marcolino-Junior, Mater. Sci. Eng., C, 2017, 79, 262–269 CrossRef CAS PubMed.
- L. Shi, Y. Li, X. Rong, Y. Wang and S. Ding, Anal. Chim. Acta, 2017, 968, 21–29 CrossRef CAS PubMed.
- P. Niu, C. Fernández-Sánchez, M. Gich, C. Ayora and A. Roig, Electrochim. Acta, 2015, 165, 155–161 CrossRef CAS.
- G. J. Lee, C. K. Kim, M. K. Lee and C. K. Rhee, Electroanalysis, 2010, 22, 530–535 CrossRef CAS.
- M. K. Silva and I. Cesarino, Int. J. Environ. Anal. Chem., 2020, 1–15 Search PubMed.
- B. Silwana, C. van der Horst, E. Iwuoha and V. Somerset, Electroanalysis, 2016, 28, 1597–1607 CrossRef CAS.
- K. E. Toghill, L. Xiao, G. G. Wildgoose and R. G. Compton, Electroanalysis, 2009, 21, 1113–1118 CrossRef CAS.
- K. Li, Q. Liu, H. Cheng, M. Hu and S. Zhang, Spectrochim. Acta, Part A, 2021, 249, 119286 CrossRef CAS PubMed.
- X. Chen, S. Chen, W. Huang, J. Zheng and Z. Li, Electrochim. Acta, 2009, 54, 7370–7373 CrossRef CAS.
- Y. Yang, W. Huang, J. Zheng and Z. Li, J. Solid State Electrochem., 2012, 16, 803–809 CrossRef CAS.
- Z. Koudelkova, T. Syrovy, P. Ambrozova, Z. Moravec, L. Kubac, D. Hynek, L. Richtera and V. Adam, Sensors, 2017, 17, 1832 CrossRef PubMed.
- Z. Zhai, N. Huang, H. Zhuang, L. Liu, B. Yang, C. Wang, Z. Gai, F. Guo, Z. Li and X. Jiang, Appl. Surf. Sci., 2018, 457, 1192–1201 CrossRef CAS.
- J. Mettakoonpitak, J. Mehaffy, J. Volckens and C. S. Henry, Electroanalysis, 2017, 29, 880–889 CrossRef CAS.
- N. Ruecha, N. Rodthongkum, D. M. Cate, J. Volckens, O. Chailapakul and C. S. Henry, Anal. Chim. Acta, 2015, 874, 40–48 CrossRef CAS PubMed.
- U. Injang, P. Noyrod, W. Siangproh, W. Dungchai, S. Motomizu and O. Chailapakul, Anal. Chim. Acta, 2010, 668, 54–60 CrossRef CAS PubMed.
- Y. Wu, N. B. Li and H. Q. Luo, Sens. Actuators, B, 2008, 133, 677–681 CrossRef CAS.
|
This journal is © The Royal Society of Chemistry 2023 |
Click here to see how this site uses Cookies. View our privacy policy here.