DOI:
10.1039/D3RA00088E
(Paper)
RSC Adv., 2023,
13, 6993-6999
Copper-catalyzed selective C5-H bromination and difluoromethylation of 8-aminoquinoline amides using ethyl bromodifluoroacetate as the bifunctional reagent†
Received
5th January 2023
, Accepted 13th February 2023
First published on 1st March 2023
Abstract
A simple and effective method for the copper-catalyzed selective C5-H bromination and difluoromethylation of 8-aminoquinoline amides with ethyl bromodifluoroacetate as the bifunctional reagent was developed. The combination of cupric catalyst and alkaline additive results in a C5-bromination reaction, whereas cuprous catalyst combined with silver additive results in the C5-difluoromethylation reaction. This method has a broad substrate scope and allows for easy and convenient access to desired C5-functionalized quinolones with good to excellent yields.
Introduction
8-Aminoquinoline derivatives have attracted widespread interest over the past decades because the privileged scaffold can be found in a wide variety of important compounds, including natural products,1 bioactive molecules,2 pharmaceuticals,3 agrochemicals,4 pesticides,5 optoelectronic materials, and fluorescence probe materials.6 Moreover, the efficient and reversible coordination to transition metal catalysts, the low cost, and ease of attachment and detachment from most carboxylic acid substrates make the 8-aminoquinoline a widely applicable auxiliary group for proximal C–H functionalization (Fig. 1).7–9 However, extensive research on the structural optimization of lead compounds suggests that the introduction of functional groups onto 8-aminoquinoline motifs could enhance their bioactivities, such as antimalarial and antitumor properties.10 Therefore, the synthesis of substituted 8-aminoquinolines is crucial.
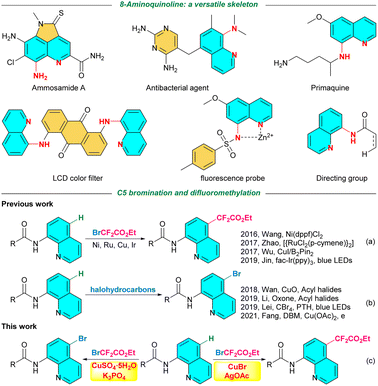 |
| Fig. 1 Synthetic strategies toward C5-brominated and difluoromethylated 8-aminoquinolines and representative 8-aminoquinoline-containing molecules. | |
In recent years, direct C–H activation has emerged as an elegant method for the rapid generation of functionalized 8-aminoquinolines due to its mild conditions, atom economy, and high tolerance to various functional groups. Numerous studies have reviewed the regioselective functionalization of 8-aminoquinolines through C–H activation at the positions of C2–C7.11 Since Stahl et al.12 reported the first instance of cuprous-catalyzed chlorination using lithium chloride in an oxygen atmosphere, remote C5-functionalization has garnered particular interest.13 Recent efforts have been focused on C–C,14 C–N,15 C–P,16 C–O,17 C–S18 and C–X19 bond construction through direct C5-H functionalization via transition-metal-catalyzed reactions as well as by employing metal-free conditions.
The difluoromethylene group, which can be proposed as the bioisostere of hydroxyl, carbonyl, amino, and sulfhydryl groups, has evolved as an important structural motif in drug research and development. Previous research revealed that bromodifluoroacetates are attractive reagents to incorporate difluoromethylene group due to their post functionalization potential. As illustrated in Fig. 1a, Wang, Zhao, Wu, and Jin, performed the ethoxycarbonyldifluoromethylation of 8-aminoquinolines with the aid of Ni, Ru, Cu, and Ir catalysts through direct C5-H functionalization employing ethyl bromodifluoroacetate as the difluoromethylation reagent.20 These intelligent strategies demonstrated that ethyl difluorobromoacetate is an excellent reagent for introducing the CF2CO2Et group on the C5-position of 8-aminoquinolines.
On the other hand, brominated aromatics are important compounds in organic synthesis. To avoid the direct use of hazardous, toxic, and harmful molecular bromine, a large number of efficient brominating reagents and reaction systems for the bromination of arenes in a safe and environmentally friendly manner have been developed. Bromoamide derivatives and other Br-containing chemicals such as HBr, bromine salts, acyl bromides and bromoalkanes have been frequently employed as brominating reagents for the incorporation of the bromine atom into 8-aminoquinolines through direct C5-H functionalization pathway.19 In addition, the use of halohydrocarbons as brominating reagents has gained traction due to their efficiency, safety, mild reaction conditions, and low pollution. Wan and Li independently reported two methods for the one-pot N-acylation and C5-H halogenation of 8-aminoquinolines using acyl halides as the donors of both the acyl and halide atoms. Lei and Fang developed the C5-bromination of 8-aminoquinoline amides using inert halomethanes as the halogen source, utilizing photocatalytic and electrocatalytic technologies (Fig. 1b).21 However, to the best of our knowledge, the direct C5-H bromination of 8-aminoquinolines using ethyl difluorobromoacetate as the organic halogen source has not been previously reported. Hence, to address the gap in knowledge, this study presents a simple and efficient method for copper-catalyzed selective C5-H bromination and difluoromethylation of 8-aminoquinoline amides with ethyl bromodifluoroacetate as the bifunctional reagent (Fig. 1c).
Results and discussion
N-(Quinolin-8-yl)benzamide (1a, 0.2 mmol) and ethyl bromodifluoroacetate (2, 0.8 mmol) were selected as the model substrates for the optimization of reaction conditions, and the results are shown in Table 1. Initially, the reaction was carried out in DMSO (1.0 mL) at 100 °C for 12 h under air in the presence of a catalytic amount of CuCl2 (20 mol%) using K2CO3 as the additive. The result gave N-(5-bromoquinolin-8-yl)benzamide (3a) rather than ethyl 2-(8-benzamidoquinolin-5-yl)-2,2-difluoroacetate (4a) as the only product obtained with a 32% yield (Table 1, entry 1). The catalysts were then extensively screened, resulting in a significant improvement of the yield of the brominated product 3a to 75% when CuSO4 was employed, and the reaction yields improved further to 84% when CuSO4·5H2O was employed as the catalyst (Table 1, entries 2 and 3). Interestingly, cuprous salts were also capable of promoting the transformation with a significant yield (Table 1, entry 4). Numerous additives, including Li2CO3, Cs2CO3, KHCO3, and K3PO4, were investigated, and the experimental results revealed that K3PO4 was the most efficient alkaline additive (96%) of the group (Table 1, entries 5–8). In addition, DMF, MeCN, and DCE solvents were also examined; the results were insignificant for the bromination reaction (Table 1, entries 9–11). The effects of some crucial factors, such as the loading of copper catalyst, the dosage of the additive, the amount of ethyl bromodifluoroacetate (2), as well as the reaction temperature, were also investigated (Table 1, entries 12–15).
Table 1 Optimization of the copper-catalyzed C5-selective functionalization reactiona

|
Entry |
Cu catalyst (mol%) |
Ligand (mol%) |
Additive (mmol) |
Solvent |
3a yieldb (%) |
4a yieldb (%) |
Reaction conditions: 1a (0.2 mmol), 2 (0.8 mmol), Cu catalyst, ligand, additive, solvent (1.0 mL). 1H NMR yield with dibromomethane as the internal standard, isolated yield in parentheses. 0.6 mmol 2 was used. Stirred at 80 °C. TMEDA = N,N,N′,N′-tetramethylethylenediamine; AdCO2H = 1-adamantanecarboxylic acid; Ac-Gly-OH = N-acetylglycine. |
1 |
CuCl2 (20) |
— |
K2CO3 (0.2) |
DMSO |
32 |
— |
2 |
CuSO4 (20) |
— |
K2CO3 (0.2) |
DMSO |
75 |
— |
3 |
CuSO4·5H2O (20) |
— |
K2CO3 (0.2) |
DMSO |
84 |
— |
4 |
CuCl (20) |
— |
K2CO3 (0.2) |
DMSO |
78 |
— |
5 |
CuSO4·5H2O (20) |
— |
Li2CO3 (0.2) |
DMSO |
82 |
— |
6 |
CuSO4·5H2O (20) |
— |
Cs2CO3 (0.2) |
DMSO |
88 |
— |
7 |
CuSO4·5H2O (20) |
— |
KHCO3 (0.2) |
DMSO |
92 |
— |
8 |
CuSO4·5H2O (20) |
— |
K3PO4 (0.2) |
DMSO |
96 (93) |
— |
9 |
CuSO4·5H2O (20) |
— |
K3PO4 (0.2) |
DMF |
5 |
— |
10 |
CuSO4·5H2O (20) |
— |
K3PO4 (0.2) |
MeCN |
— |
— |
11 |
CuSO4·5H2O (20) |
— |
K3PO4 (0.2) |
DCE |
— |
— |
12 |
CuSO4·5H2O (10) |
— |
K3PO4 (0.2) |
DMSO |
68 |
— |
13 |
CuSO4·5H2O (20) |
— |
K3PO4 (0.1) |
DMSO |
— |
— |
14c |
CuSO4·5H2O (20) |
— |
K3PO4 (0.2) |
DMSO |
81 |
— |
15d |
CuSO4·5H2O (20) |
— |
K3PO4 (0.2) |
DMSO |
— |
— |
16 |
CuSO4·5H2O (50) |
— |
AgOAc (0.4) |
DMSO |
— |
— |
17 |
Cu(OAc)2 (50) |
— |
AgOAc (0.4) |
DMSO |
— |
37 |
18 |
CuBr (50) |
— |
AgOAc (0.4) |
DMSO |
— |
62 |
19 |
CuI (50) |
— |
AgOAc (0.4) |
DMSO |
— |
49 |
20 |
CuBr (50) |
— |
Ag2O (0.4) |
DMSO |
— |
— |
21 |
CuBr (50) |
— |
Ag2CO3 (0.4) |
DMSO |
— |
— |
22 |
CuBr (50) |
— |
AgOAc (0.4) |
DMF |
— |
38 |
23 |
CuBr (50) |
— |
AgOAc (0.4) |
MeCN |
— |
28 |
24 |
CuBr (50) |
— |
AgOAc (0.4) |
THF |
— |
— |
25 |
CuBr (50) |
— |
AgOAc (0.4) |
Toluene |
— |
— |
26 |
CuBr (50) |
TMEDA (100) |
AgOAc (0.4) |
DMSO |
— |
43 |
27 |
CuBr (50) |
AdCO2H (100) |
AgOAc (0.4) |
DMSO |
— |
50 |
28 |
CuBr (50) |
Ac-Gly-OH (100) |
AgOAc (0.4) |
DMSO |
— |
66 |
29 |
CuBr (20) |
Ac-Gly-OH (40) |
AgOAc (0.4) |
DMSO |
— |
67 (62) |
30 |
CuBr (10) |
Ac-Gly-OH (20) |
AgOAc (0.4) |
DMSO |
— |
40 |
31 |
CuBr (20) |
Ac-Gly-OH (40) |
AgOAc (0.2) |
DMSO |
— |
35 |
32c |
CuBr (20) |
Ac-Gly-OH (40) |
AgOAc (0.4) |
DMSO |
— |
52 |
33d |
CuBr (20) |
Ac-Gly-OH (40) |
AgOAc (0.4) |
DMSO |
— |
63 |
Previous literature suggested that silver salts could be used as single electron oxidants to initiate radical transformations in α-carbonyl alkyl bromide reactions.22 Further investigation of the copper catalyzed C5-difluoromethylation reaction of 8-aminoquinoline amides was carried out employingAgOAc as the additive. Based on the optimal conditions that were established for the C5-bromination, the reaction was carried out in the presence of CuSO4·5H2O (50 mol%) in DMSO (1.0 mL) with AgOAc (0.4 mmol) at 100 °C under an N2 atmosphere for 12 h. This yielded no desired product (4a) (Table 1, entry 16). Subsequent screening revealed that CuBr outperformed Cu(OAc)2 and CuI in terms of activity, and the desired product 4a was obtained at a 62% yield (Table 1, entries 17–19). Other silver additives like Ag2O and Ag2CO3 could not promote the difluoromethylation reaction (Table 1, entries 20 and 21). Several other solvents, such as DMF, MeCN, THF, and toluene, were examined, but none of them was as effective as DMSO (Table 1, entries 22–25). Then, a series of ligands were also screened; the addition of ligands did not significantly increase the yield of the desired product; however, the loading of the copper catalyst could be decreased to 20 mol% (Table 1, entries 26–30). Contradictory experiments revealed that the yield decreased with decreased amounts of AgOAc, ethyl bromodifluoroacetate (2) and the reaction temperature (Table 1, entries 31–33).
With the optimized reaction conditions in hand, these protocols were then applied to a series of 8-aminoquinoline amides, and the results are shown in Fig. 2 and 3. Based on these results, it can be concluded that the present reactions possessed the advantages of broad substrate scopes, mild reaction conditions, moderate to excellent product yields, and remarkable functional group tolerance. Initially, the substrate scope for the C5-bromination reaction was explored (Fig. 2). 8-Aminoquinoline amides with a variety of substituted groups on different positions of benzene rings in the benzoic acid section exhibited high reactivity (3b–3k). Aromatic amides with electron-donating substituted groups (Me, OMe, OAc) and electron-withdrawing substituted groups (CF3, F, Cl, Br, I, CO2Me, CN) were well tolerated in this reaction, resulting in an 84% to almost quantitative yield. Furthermore, 8-aminoquinoline amide containing multiple methoxyl groups behaved well in the reaction and gave the product 3l in a 96% yield. Notably, the compatibility with halogen atoms, particularly I, Br, and Cl, enables the brominated products to undergo downstream transformations (3f–3h). Moreover, to extend the substrate scope, various aliphatic amides, including phenylacetamide, isopropylamide, and pivalamide, were evaluated and provided the desired products in good yields (3m–3o). In addition, amides with tension rings and polycyclic structures also survived in this reaction, affording the target molecules good to excellent yields (3p,3q). Satisfactorily, the methoxy-substituted quinoline at the C6 position was compatible and provided the desired product 3r in a 92% yield.
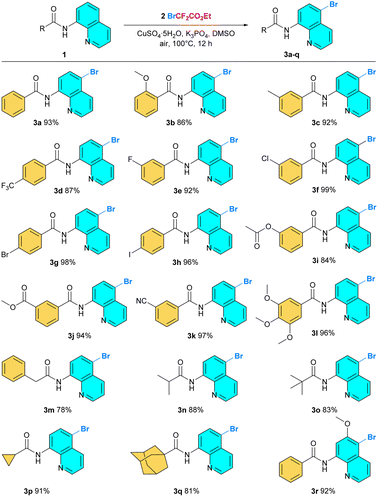 |
| Fig. 2 Substrate scope for the C5-bromination of 8-aminoquinoline amides. | |
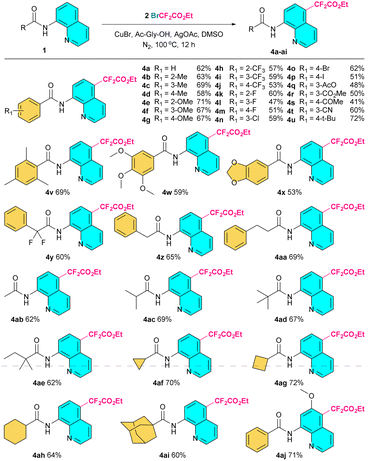 |
| Fig. 3 Substrate scope for the C5-difluoromethylation of 8-aminoquinoline amides. | |
Next, the applicability of the C5-difluoromethylation reaction for different 8-aminoquinoline amides was extensively investigated. As listed in Fig. 3, the results clearly show that aromatic amides with a variety of functional groups on the benzene ring as well as different kinds of aliphatic amides were able to accomplish the difluoromethylation reaction with ethyl bromodifluoroacetate (2) smoothly, affording the desired coupling products 4a–4ai in moderate to good yields. The ethoxycarbonyldifluoromethyl group could be introduced onto α,α-difluorophenylacetamide through this reaction to afford the bis-difluoromethylated product 4y in 60% yield. In addition, halogen atoms could be preserved integrally in this transformation, although a silver acetate additive was involved (4k–4p). Moreover, the substrate with a methoxyl group on the quinoline scaffold proceeded well to afford the difluoromethylated product in a 71% yield (4aj).
Finally, to gain a preliminary understanding of these reactions, some extended investigations were performed (Scheme 1). Firstly, we failed to apply this method to N-methyl-N-(quinolin-8-yl)benzamide (5), quinolin-8-yl benzoate (6), N-(naphthalen-1-yl)benzamide (7), 8-aminoquinoline (8), and its alkylated derivatives (9, 10). These revealed that the N,N-bidentate chelating coordination of the 8-aminoquinoline amide skeleton is necessary. Subsequently, several control experiments were carried out to investigate the mechanisms. It was observed that the bromination reaction continued in the presence of free radical scavengers such as 2,2,6,6-tetramethyl-1-piperidinyloxy (TEMPO) and 2,6-di-tertbutyl-4-methyphenol (BHT), while the difluoromethylation reaction was completely inhibited. In addition, the corresponding product 3a was obtained in 90% yield when the bromination reaction was conducted under nitrogen atmosphere. However, when the difluoromethylation reaction was carried out in an air atmosphere, no product was obtained, and the initial material was recovered. Meanwhile, the TEMPO-CF2CO2Et adduct was detected by GC-MS (see ESI†). These results indicated that the difluoromethylation might proceed via a free radical process and the bromination might be accomplished through an ionic pathway.
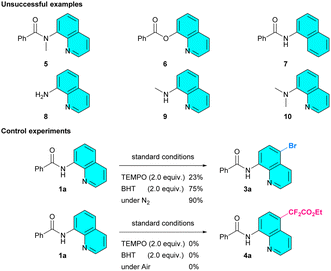 |
| Scheme 1 Extended investigations. | |
Although the precise mechanisms remain unclear to date, on the basis of our experimental results and literature,23 plausible mechanisms are proposed. As shown in Scheme 2(a), the nucleophilic attack of DMSO on ethyl bromodifluoroacetate (2) to give intermediate A, which then reacts with the bromine anion to produce intermediate B. Subsequently, dimethylsulfonium bromide or dimethyl thioether/molecular bromine complex C is generated by the attack of the bromine anion on intermediate B.23a,b Finally, bromination is accomplished through the aromatic electrophilic substitution of intermediate C with 1a with the help of copper salt. In Scheme 2(b), a mechanistic hypothesis for the difluoromethylation of 8-aminoquinoline amide is also proposed. Firstly, CuOAc is provided by the anion exchange of CuBr with AgOAc. The Cu(I)-quinoline complex A′ is then formed via bidentate nitrogen atom chelation. The intermolecular SET from A′ to ethyl bromodifluoroacetate (2) followed by the deprotonation in the presence of AgOAc produces ·CF2CO2Et radical and the Cu(II) complex B′.23c,d Subsequently, the intramolecular SET process within B′ produces a cationic radical C′.18g The radical coupling reaction of C′ with CF2CO2Et affords the cationic intermediate D′. The obtained D′ undergoes deprotonation and demetallation to yield the desired product 4a as well as the regenerated Cu(I) catalyst for the next catalytic cycle. Further investigation is being conducted to provide evidence for the proposed mechanism.
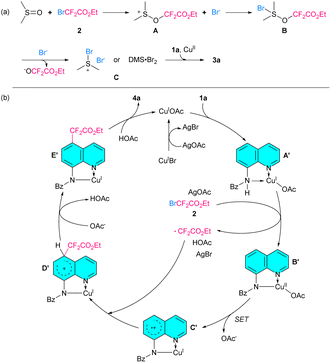 |
| Scheme 2 Proposed mechanisms. | |
Conclusions
In summary, we have established a simple and efficient method for copper-catalyzed selective C5-H bromination and difluoromethylation of 8-aminoquinoline amides with ethyl bromodifluoroacetate. The results showed that when cupric catalyst is combined with an alkaline additive, it leads to the C5-bromination reaction while the C5-difluoromethylation reaction is dominant for cuprous catalyst combined with a silver additive. This method has mild reaction conditions, moderate to excellent product yields, and a wide range of substrates. Future investigations will focus on mechanism details and other selective functionalization reactions employing halogenated hydrocarbons as bifunctional reagents.
Experimental
General procedure for the C5-bromination
A 35 mL sealed tube equipped with a stir bar was charged with N-(quinolin-8-yl)benzamide (49.6 mg, 0.2 mmol, 1.0 equiv.), BrCF2CO2Et (104 μL, 0.8 mmol, 4.0 equiv.), CuSO4·5H2O (10.0 mg, 0.04 mmol, 20 mol%), K3PO4 (42.5 mg, 0.2 mmol, 1.0 equiv.) and DMSO (1.0 mL). The tube was sealed with a Teflon cap under air, then the mixture was stirred at 100 °C for 12 h. After completion, the reaction mixture was diluted with ethyl acetate (20 mL) and washed successively with saturated sodium bicarbonate, saturated sodium sulfide, and brine. The organic layer was dried over anhydrous sodium sulfate and concentrated in vacuo. The residue was purified on preparative thin layer chromatography (PTLC) to afford the desired product 3a.
General procedure for the C5-difluoromethylation
A 35 mL Schlenk tube equipped with a stir bar was charged with N-(quinolin-8-yl)benzamide (49.6 mg, 0.2 mmol, 1.0 equiv.), CuBr (5.8 mg, 0.04 mmol, 20 mol%), Ac-Gly-OH (9.4 mg, 0.08 mmol, 40 mol%), and AgOAc (67 mg, 0.4 mmol, 2.0 equiv.) under air. The tube was sealed with a rubber stopper and then evacuated and backfilled with N2 five times. BrCF2CO2Et (104 μL, 0.8 mmol, 4.0 equiv.) and DMSO (1.0 mL) were injected via syringe. After the reaction was stirred at 100 °C for 12 h, it was allowed to cool to room temperature. The reaction mixture was diluted with ethyl acetate (20 mL) and then washed successively with saturated sodium bicarbonate, saturated sodium sulfide, and brine. The organic layer was dried over anhydrous sodium sulfate and concentrated in vacuo. The residue was purified on PTLC to afford the desired product 4a.
Conflicts of interest
There are no conflicts to declare.
Acknowledgements
This work was supported by the National Natural Science Foundation of China (21801088), the Natural Science Foundation of Jiangsu Province (BK20181065), and the Natural Science Foundation of the Higher Education Institutions of Jiangsu Province (18KJB150006).
Notes and references
-
(a) H. Nagata, K. Ochiai, Y. Aotani, K. Ando, M. Yoshida, I. Takahashi and T. Tamaoki, J. Antibiot., 1997, 50, 537 CrossRef CAS PubMed;
(b) C. C. Hughes, J. B. MacMillan, S. P. Gaudêncio, P. R. Jensen and W. Fenical, Angew. Chem., Int. Ed., 2009, 48, 725 CrossRef CAS PubMed.
-
(a) G. White, S. M. Daluge, C. W. Sigel, R. Ferone and H. R. Wilson, Res. Vet. Sci., 1993, 54, 372 CrossRef CAS PubMed;
(b) R. Singh, A. Sran, D. C. Carroll, J. Huang, L. Tsvetkov, X. Zhou, J. Sheung, J. McLaughlin, S. D. Issakani, D. G. Payan and S. J. Shaw, Bioorg. Med. Chem. Lett., 2015, 25, 5199 CrossRef CAS PubMed.
-
(a) J. K. Baird, Clin. Microbiol. Rev., 2019, 32, e00011 CrossRef PubMed;
(b) J. E. Frampton, Drugs, 2018, 78, 1517 CrossRef CAS PubMed;
(c) N. Vale, R. Moreira and P. Gomes, Eur. J. Med. Chem., 2009, 44, 937 CrossRef CAS PubMed.
- J. A. Bond and T. W. Walker, Weed Technol., 2012, 26, 183 CrossRef CAS.
- R. Yang, W. Du, H. Yuan, T. Qin, R. He, Y. Ma and H. Du, Mol. Diversity, 2020, 24, 1065 CrossRef CAS PubMed.
-
(a) N. S. Mohamad, N. H. Zakaria, N. Daud, L. L. Tan, G. C. Ta, L. Y. Heng and N. I. Hassan, Sensors, 2021, 21, 311 CrossRef CAS PubMed;
(b) S. Lee, S. Park and J. Park, Mol. Cryst. Liq. Cryst., 2013, 583, 29 CrossRef CAS.
-
(a) Y.-H. Zhang, G.-F. Shi and J.-Q. Yu, in Comprehensive Organic Synthesis II, Elsevier, 2014, p. 1101 Search PubMed;
(b) C-H Activation, ed. J.-Q. Yu and Z. Shi, Springer Berlin Heidelberg, Berlin, Heidelberg, 2010, vol. 292 Search PubMed;
(c) R. G. Bergman, Nature, 2007, 446, 391 CrossRef CAS PubMed;
(d) V. G. Zaitsev, D. Shabashov and O. Daugulis, J. Am. Chem. Soc., 2005, 127, 13154 CrossRef CAS PubMed;
(e) J. A. Labinger and J. E. Bercaw, Nature, 2002, 417, 507 CrossRef CAS PubMed.
-
(a) S. St John-Campbell and J. A. Bull, Adv. Synth. Catal., 2019, 361, 3662 CrossRef CAS;
(b) B. Zhang, H. Guan, B. Liu and B. Shi, Chin. J. Org. Chem., 2014, 34, 1487 CrossRef CAS;
(c) G. Rouquet and N. Chatani, Angew. Chem., Int. Ed., 2013, 52, 11726 CrossRef CAS PubMed.
-
(a) L. S. Fitzgerald and M. L. O'Duill, Chem.–Eur. J., 2021, 27, 8411 CrossRef CAS PubMed;
(b) M. R. Yadav, R. K. Rit, M. Shankar and A. K. Sahoo, Asian J. Org. Chem., 2015, 4, 846 CrossRef CAS.
- W.-B. Kuang, R.-Z. Huang, Y.-L. Fang, G.-B. Liang, C.-H. Yang, X.-L. Ma and Y. Zhang, RSC Adv., 2018, 8, 24376 RSC.
-
(a) A. Corio, C. Gravier-Pelletier and P. Busca, Molecules, 2021, 26, 5467 CrossRef CAS PubMed;
(b) Z. Xu, X. Yang, S.-F. Yin and R. Qiu, Top. Curr. Chem., 2020, 378, 42 CrossRef CAS PubMed;
(c) T. Iwai and M. Sawamura, ACS Catal., 2015, 5, 5031 CrossRef CAS.
- A. M. Suess, M. Z. Ertem, C. J. Cramer and S. S. Stahl, J. Am. Chem. Soc., 2013, 135, 9797 CrossRef CAS PubMed.
-
(a) B. Khan, H. S. Dutta and D. Koley, Asian J. Org. Chem., 2018, 7, 1270 CrossRef CAS;
(b) L. Zhu, X. Cao, Y. Li, T. Liu, X. Wang, R. Qiu and S. Yin, Chin. J. Org. Chem., 2017, 37, 1613 CrossRef CAS.
-
(a) D. Liu, Z. Xia, Y. Xiao, Y. Yu, L. Yu, Z. Song, Q. Wu, J. Zhang and Z. Tan, Eur. J. Org. Chem., 2021, 2021, 5012 CrossRef CAS;
(b) W. Xu, M. Wu and N. Yoshikai, Synthesis, 2021, 53, 3144 CrossRef CAS;
(c) W.-Y. Shi, Y.-N. Ding, C. Liu, N. Zheng, X.-Y. Gou, M. Li, Z. Zhang, H.-C. Liu, Z.-J. Niu and Y.-M. Liang, Chem. Commun., 2020, 56, 12729 RSC;
(d) F. Zhan, W. Zhang and H. Zhao, Synthesis, 2020, 52, 1007 CrossRef CAS;
(e) C. Wen, R. Zhong, Z. Qin, M. Zhao and J. Li, Chem. Commun., 2020, 56, 9529 RSC;
(f) A. Mariappan, K. M. Das and M. Jeganmohan, Org. Biomol. Chem., 2018, 16, 3419 RSC;
(g) M. Cui, J.-H. Liu, X.-Y. Lu, X. Lu, Z.-Q. Zhang, B. Xiao and Y. Fu, Tetrahedron Lett., 2017, 58, 1912 CrossRef CAS;
(h) M. D. Reddy, F. R. Fronczek and E. B. Watkins, Org. Lett., 2016, 18, 5620 CrossRef CAS PubMed;
(i) X. Cong and X. Zeng, Org. Lett., 2014, 16, 3716 CrossRef CAS PubMed;
(j) K. Wang, J. Hou, Y. Lv, T. Wei, R. Bai and Y. Xie, Asian J. Org. Chem., 2021, 10, 559 CrossRef CAS;
(k) S. Han, Q. Wu, L. Mele, L. Ding, J. Li, D. Zou, Y. Wu and Y. Wu, Tetrahedron Lett., 2019, 60, 151077 CrossRef CAS;
(l) T. Sahoo, C. Sen, H. Singh, E. Suresh and S. C. Ghosh, Adv. Synth. Catal., 2019, 361, 3950 CrossRef CAS;
(m) P. B. Arockiam, L. Guillemard and J. Wencel-Delord, Adv. Synth. Catal., 2017, 359, 2571 CrossRef CAS.
-
(a) M. Pei, C. Zu, Z. Liu, F. Yang and Y. Wu, J. Org. Chem., 2021, 86, 11324 CrossRef CAS PubMed;
(b) R. Zhao, Y. Yang, X. Wang, P. Ren, Q. Zhang and D. Li, RSC Adv., 2018, 8, 37064 RSC;
(c) Y. Yin, J. Xie, F.-Q. Huang, L.-W. Qi and B. Zhang, Adv. Synth. Catal., 2017, 359, 1037 CrossRef CAS;
(d) H. Yi, H. Chen, C. Bian, Z. Tang, A. K. Singh, X. Qi, X. Yue, Y. Lan, J.-F. Lee and A. Lei, Chem. Commun., 2017, 53, 6736 RSC;
(e) Y. Dou, Z. Xie, Z. Sun, H. Fang, C. Shen, P. Zhang and Q. Zhu, ChemCatChem, 2016, 8, 3570 CrossRef CAS;
(f) C. J. Whiteoak, O. Planas, A. Company and X. Ribas, Adv. Synth. Catal., 2016, 358, 1679 CrossRef CAS;
(g) Y. He, N. Zhao, L. Qiu, X. Zhang and X. Fan, Org. Lett., 2016, 18, 6054 CrossRef CAS PubMed.
- H. Qiao, S. Sun, Y. Zhang, H. Zhu, X. Yu, F. Yang, Y. Wu, Z. Li and Y. Wu, Org. Chem. Front., 2017, 4, 1981 RSC.
-
(a) C.-K. Liu, M.-Y. Chen, X.-X. Lin, Z. Fang and K. Guo, Green Chem., 2020, 22, 6437 RSC;
(b) T. Liang, X. He, D. Ji, H. Wu, Y. Xu, Y. Li, Z. Wang, Y. Xu and Q. Zhu, Eur. J. Org. Chem., 2019, 2019, 2513 CrossRef CAS;
(c) G. Wang, J. Han, K. Wang, H. Li, G. Duan, C. Xia and F. Li, Catal. Commun., 2018, 114, 37 CrossRef CAS;
(d) X. Yao, X. Weng, K. Wang, H. Xiang and X. Zhou, Green Chem., 2018, 20, 2472 RSC;
(e) Z. Begum, G. Bhavani, B. Sridhar and B. Reddy, Synthesis, 2018, 50, 4089 CrossRef CAS.
-
(a) X. Liu, H. Zhang, F. Yang and B. Wang, Org. Biomol. Chem., 2019, 17, 7564 RSC;
(b) K. Wang, G. Wang, G. Duan and C. Xia, RSC Adv., 2017, 7, 51313 RSC;
(c) G. Chen, X. Zhang, Z. Zeng, W. Peng, Q. Liang and J. Liu, ChemistrySelect, 2017, 2, 1979 CrossRef CAS;
(d) S. Liang and G. Manolikakes, Adv. Synth. Catal., 2016, 358, 2371 CrossRef CAS;
(e) C. Xia, K. Wang, J. Xu, Z. Wei, C. Shen, G. Duan, Q. Zhu and P. Zhang, RSC Adv., 2016, 6, 37173 RSC;
(f) Q. Yu, Y. Yang, J.-P. Wan and Y. Liu, J. Org. Chem., 2018, 83, 11385 CrossRef CAS PubMed;
(g) J. Chen, T. Wang, T. Wang, A. Lin, H. Yao and J. Xu, Org. Chem. Front., 2017, 4, 130 RSC;
(h) L. Zhu, R. Qiu, X. Cao, S. Xiao, X. Xu, C.-T. Au and S.-F. Yin, Org. Lett., 2015, 17, 5528 CrossRef CAS PubMed.
-
(a) X. He, Y. Jiang, H. Zhou and Q. Zhu, ChemistrySelect, 2021, 6, 5191 CrossRef CAS;
(b) X. Yang, Q.-L. Yang, X.-Y. Wang, H.-H. Xu, T.-S. Mei, Y. Huang and P. Fang, J. Org. Chem., 2020, 85, 3497 CrossRef CAS PubMed;
(c) J. Hou, K. Wang, C. Zhang, T. Wei, R. Bai and Y. Xie, Eur. J. Org. Chem., 2020, 2020, 6382 CrossRef CAS;
(d) D. R. Motati, D. Uredi and E. B. Watkins, Chem. Sci., 2018, 9, 1782 RSC;
(e) L. Qiao, X. Cao, K. Chai, J. Shen, J. Xu and P. Zhang, Tetrahedron Lett., 2018, 59, 2243 CrossRef CAS;
(f) H. Chen, P. Li, M. Wang and L. Wang, Eur. J. Org. Chem., 2018, 2018, 2091 CrossRef CAS;
(g) J.-Y. Jiao, Y.-J. Mao, A.-W. Feng, X.-F. Li, M.-T. Li and X.-H. Zhang, Tetrahedron, 2017, 73, 1482 CrossRef CAS;
(h) J. Ding, Y. Zhang and J. Li, Org. Chem. Front., 2017, 4, 1528 RSC;
(i) H. S. Dutta, B. Khan, A. A. Khan, Raziullah, A. Ahmad, R. Kant and D. Koley, ChemistrySelect, 2017, 2, 6488 CrossRef CAS;
(j) H. Guo, M. Chen, P. Jiang, J. Chen, L. Pan, M. Wang, C. Xie and Y. Zhang, Tetrahedron, 2015, 71, 70 CrossRef CAS.
-
(a) H. Chen, P. Li, M. Wang and L. Wang, Org. Lett., 2016, 18, 4794 CrossRef CAS PubMed;
(b) C. Chen, R. Zeng, J. Zhang and Y. Zhao, Eur. J. Org. Chem., 2017, 2017, 6947 CrossRef CAS;
(c) S. Han, A. Liang, X. Ren, X. Gao, J. Li, D. Zou, Y. Wu and Y. Wu, Tetrahedron Lett., 2017, 58, 4859 CrossRef CAS;
(d) C. Jin, R. Zhu, B. Sun, L. Zhang, X. Zhuang and C. Yu, Asian J. Org. Chem., 2019, 8, 2213 CrossRef CAS;
(e) P. B. Arockiam, L. Guillemard and J. Wencel-Delord, Adv. Synth. Catal., 2017, 359, 2571 CrossRef CAS;
(f) X. Jiang, Y. Jiang, Q. Liu, B. Li, D.-Q. Shi and Y. Zhao, J. Org. Chem., 2022, 87, 3546 CrossRef CAS PubMed.
-
(a) Y. Du, Y. Liu and J.-P. Wan, J. Org. Chem., 2018, 83, 3403 CrossRef PubMed;
(b) D. Li, Z. Jia, Y. Jiang, J. Jia, X. Zhao, Z. Li and Z. Xu, ChemistrySelect, 2019, 4, 13964 CrossRef CAS;
(c) B. Ma, F. Lu, H. Yang, X. Gu, Z. Li, R. Li, H. Pei, D. Luo, H. Zhang and A. Lei, Asian J. Org. Chem., 2019, 8, 1136 CrossRef CAS;
(d) X. Lin, C. Zeng, C. Liu, Z. Fang and K. Guo, Org. Biomol. Chem., 2021, 19, 1352 RSC;
(e) B. Huang, Y. Zhao, C. Yang, Y. Gao and W. Xia, Org. Lett., 2017, 19, 3799 CrossRef CAS PubMed;
(f) V. Botla, A. Akudari and C. Malapaka, Tetrahedron Lett., 2019, 60, 115 CrossRef CAS;
(g) Y. Liang, F. Lin, Y. Adeli, R. Jin and N. Jiao, Angew. Chem., Int. Ed., 2019, 58, 4566 CrossRef CAS PubMed;
(h) F. Liu, N. Wu and X. Cheng, Org. Lett., 2021, 23, 3015 CrossRef CAS PubMed.
-
(a) S. Luo, M. Min, Y. Wu, S. Jiang, Y. Xiao, R. Song and J. Li, Adv. Synth. Catal., 2020, 362, 2921 CrossRef CAS;
(b) R. Ding, Z.-D. Huang, Z.-L. Liu, T.-X. Wang, Y.-H. Xu and T.-P. Loh, Chem. Commun., 2016, 52, 5617 RSC;
(c) X.-H. Ouyang, R.-J. Song, M. Hu, Y. Yang and J.-H. Li, Angew. Chem., Int. Ed., 2016, 55, 3187 CrossRef CAS PubMed;
(d) Y. Yu and U. K. Tambar, Chem. Sci., 2015, 6, 2777 RSC.
-
(a) X. Chen, W. Li, W. Zhang, Z. Wang and H. Cui, Asian J. Org. Chem., 2022, 11, e202200342 CAS;
(b) J.-Q. Li, X.-H. Chen, X.-X. Wang and H.-L. Cui, Tetrahedron Lett., 2021, 82, 153375 CrossRef CAS;
(c) G.-H. Pan, R.-J. Song and J.-H. Li, Org. Chem. Front., 2018, 5, 179 RSC;
(d) J. Wei, J. Jiang, X. Xiao, D. Lin, Y. Deng, Z. Ke, H. Jiang and W. Zeng, J. Org. Chem., 2016, 81, 946 CrossRef CAS PubMed.
|
This journal is © The Royal Society of Chemistry 2023 |