DOI:
10.1039/D2RA08022B
(Paper)
RSC Adv., 2023,
13, 5134-5148
Spirocyclic rhodamine B benzoisothiazole derivative: a multi-stimuli fluorescent switch manifesting ethanol-responsiveness, photo responsiveness, and acidochromism†
Received
16th December 2022
, Accepted 24th January 2023
First published on 9th February 2023
Abstract
Multi-stimuli fluorescent switching materials have been extensively employed in chemistry, biochemistry, physics, and materials science. Although rhodamine-based spirolactams have been specifically considered for metal ion sensing by photoluminescence, only some of them manifest photochromic behavior, and further development of rhodamine B (RHB)-based photochromic materials is required. RHB and its cyclic amides are advantageous in various sensing applications owing to their colorimetric responses to external stimulation. Hence, the current work reports a novel multifunctional active molecular material (3′,6′-bis(diethylamino))-2-(5-nitrobenzo[c]isothiazol-3-yl)spiro[isoindoline-1,9′-xanthen]-3-one (RHBIT) by linking rhodamine B with 3-amino,5-nitro[2,1]benzoisothiazole (ANB) in a facile synthetic pathway; that perceives both emission color change and switching between off–on states. RHBIT shows acidochromism, photochromism, and pH sensitivity accompanied by unique ethanol responsiveness, with potential applications in anti-counterfeiting and drug delivery. Notably, RHBIT is highly acid sensitive and reverts to the ring-closed form on treatment with triethylamine (base), visible with the naked eye amidst colorless–pink–colorless transformations. On short UV irradiation, RHBIT provides a two-fold rise in the lifetime for the ring-open form in CHCl3 and DCM compared to the spirolactam (closed form). DFT and TDDFT studies provide electronic characterization for the absorption spectra of the open and closed forms. Using the photoresponsive feature of RHBIT, an information protection application has been enacted via a rewritable platform.
1 Introduction
Photoresponsive materials have attained great importance due to their potential applications as fluorescence sensors,1 photocontrollable switches,2 memory devices,3 and in super-resolution spectroscopy4 and volumetric displays.5 Multi-stimuli-responsive materials have been established in diverse fields like photoresponsive chemosensors,6 photodynamic therapy,7 photopatterning,2 and photoacoustic imaging.8 Hitherto, multifunctional photoresponsive materials were developed by introducing various stimuli, including pH, light, heat, solvent, mechanical force, and electric fields.9,10 Among these, light is a peerless stimulus due to its explicit properties like unambiguous stimulation besides its response, eco-friendliness, and remote non-destructive control. To date, photoresponsive molecular systems like spiropyrans, diarylethenes, azobenzenes, spirooxazine, viologen, etc., have experienced color changes by photoirradiation and been used in various fields such as physical, chemical, biological, optical, and nanotechnology.11–13 Nevertheless, rhodamine B (RHB), a class of xanthene dye with excellent spectroscopic properties, quantum efficiency, and good photostability, and a well-known sensitive switching material, undergoes fluorescence changes due to its reversible isomerization between open-ring (fluorescent) and closed-ring (non-fluorescent) forms in solution, with other surrounding conditions and sustainability, and has been used to sense metal ions in environmental samples.14 Recently, we detected that certain open-chain amide derivatives of RHB exhibited a dual effect of fluorescence and anticancer activity.15
Cyclized spirolactam is non-fluorescent due to interruption of conjugation in the xanthene moiety, while the reversible structural transition from a non-fluorescent representative form to a highly fluorescent zwitterionic form is influenced by pH, solvent polarity, and ultraviolet irradiation. Such reversible off–on behavior facilitates the importance of rhodamine spirolactams in sensing/dye activation off–on switching applications. Therefore, rhodamine B derivatives are promising candidates for contriving multifunctional fluorescent switches, and so far much effort has been put into constructing them.16,17
Gleiter et al. first disclosed the photochromic features of N-aryl spirolactam rhodamines which transform between off–on states by UV illumination, with color changes persisting for milliseconds to minutes depending upon the solvent medium.18 Hell et al. introduced a photoactivatable rhodamine phthalimide spirolactam into localization-based super-resolution imaging.19 Li et al. provided a new strategy for developing multifunctional photoresponsive materials of a 2,4-dihydroxybenzaldehyde rhodamine B hydrazone Schiff base with metal complexes as reversible, photoresponsive systems which were favorable for constructing photocontrolled logic gates with tunable performance.17 Recently, Zhiyong et al. brought about remarkable multi-stimuli-responsive behaviors, including a protonation effect, photochromism, and piezochromism with significant color changes of iso-cis/trans-aminobenzopyranoxanthenes as potential candidates for sensors, indicators, and detectors.9 Murugesapandian et al. reported an excited-state intramolecular proton transfer (ESIPT)-active multi-functional molecular switch, a coumarin rhodamine B hydrazine-based material that exhibits methanol responsiveness and reversible photochromic feature of its zinc ensemble in the presence of a 365 nm UV lamp.16 Very recently Fogerty et al. designed and synthesized a dual spirolactam moiety utilizing p-phenylene diamine that exhibited sensitivity to both pH and UV illumination; solvatochromic fluorescence emission was centered in the blue region of the spectrum, but an explanation of the linker chemistry that influences the open–closed equilibrium as well as solvatochromic emission in the dimer is lacking.20 This color change is instantaneous in the case of chloroform and takes place over a few hours in the case of dichloromethane.
The transition of rhodamine spirolactams between the closed and open ring forms by pH sensitivity and UV light irradiation (∼250–400 nm) depends on the steric and electron-withdrawing/releasing nature of the substituent groups attached to the nitrogen of the amide.4,5,21 Generally, pKa values increase when there is an increase in the size and electron-withdrawing character of the substituent group; furthermore, smaller electron-withdrawing groups favor faster ring-opening kinetics and show an open form of the spirolactam in equilibrium.22 Ring-opening kinetics also depend on solvent polarity and acidity; acidic solvents mostly favor the open form of rhodamine spirolactams.5,21 In this context, Ma et al. reported a multi-stimuli-responsive rhodamine tetraphenylethylene (RHTPE) exhibiting aggregation-induced emission (AIE), methanol responsiveness, selectivity, photochromism, and mechanochromism.23 The photoresponsive properties of RHTPE were studied in ethanol, chloroform and DMSO solvents (red emissive) under 365 nm UV light, and the solutions returned to colorless when the UV light was removed. Interestingly, though spirolactams have been explored especially for metal ion sensing through photoluminescence, only a few exhibited photochromic behavior and acid-sensing (HCl, HNO3, picric acid, TFA, etc.) as mentioned above. It should be noted, that p-toluenesulfonic acid (PTSA) is an efficient catalyst in organic synthesis and is widely used as a counterion for basic drugs in the pharmaceutical industry owing to its strong acidic and hydrophilic properties; therefore, determining the content of PTSA in drug substances is a prerequisite for drug quality control and authenticity testing. Several analytical methods utilized for the detection of PTSA in drug and environmental samples are sophisticated and need expensive instruments.24 Hence, an alternative fluorescence sensing method has become more prominent due to its high sensitivity and selectivity, operational lucidity, low cost, less time, and visible naked-eye detection.
Accordingly, we have chosen 3-amino,5-nitro[2,1]benzoisothiazole (ANB) possessing rich π-conjugation and an electron-withdrawing group; to investigate its emerging optical characteristics. In one of our earlier reports, ANB with PTSA resulted in a protonated charge transfer complex.25 Nonetheless, interestingly, in the current study, we synthesized non-fluorescent (3′,6′-bis(diethylamino))-2-(5-nitrobenzo[c]isothiazol-3-yl)spiro[isoindoline-1,9′-xanthen]-3-one (RHBIT) spirolactam that exhibits spectacular properties, such as acidochromism, solvatochromism, and photochromic properties, especially by itself. The presence of an electron-withdrawing group (–NO2) in ANB possibly favored a faster ring-opening mechanism and generated a highly fluorescent open form of RHBIT under acid and UV irradiation stimuli. Henceforth, in this article, we present a comprehensive study on the photophysical and photoresponsive attributes of an easily acquired novel rhodamine cyclized spirolactam RHBIT (obtained by linking ANB to RHB through a facile synthesis); with a crystal description, and its sensing application with PTSA in solution. This study will perhaps contribute to the development of multi-stimuli-responsive materials which can be successfully applied for multicolor inks, sensors, photo printing, displays, and biological imaging.
2 Experimental section
2.1 Materials
All reagents were procured from commercial suppliers indicated below (in brackets) and used without further purification: rhodamine B (Sigma-Aldrich), 3-amino,5-nitro[2,1]benzoisothiazole (Sigma Aldrich), 1-hydroxybenzotriazole (HOBT) (Spectrochem Pvt. Ltd.), 1-(3-dimethylaminopropyl)-3-ethyl carbodiimide hydrochloride (EDC.HCl) (Spectrochem Pvt. Ltd.), N,N-diisopropylethylamine (DIPEA) (Spectrochem Pvt. Ltd.), N,N-dimethylformamide (DMF) (SRL Pvt. Ltd.), dichloromethane (DCM) (SRL Pvt. Ltd.), acetonitrile (SRL Pvt. Ltd.), absolute ethyl alcohol (Hychem Laboratory), p-toluene sulfonic acid monohydrate (PTSA) (SRL Pvt. Ltd.) recrystallized in acetonitrile for further use. Column chromatography was accomplished using 60–325 mesh neutral alumina. Reactions were monitored by thin layered chromatography (TLC) on F254 plates (Merck & Co.) pre-coated with silica gel 60 G; visualized by a UV lamp.
2.2 Methods and measurements
FT-IR spectra were taken on a Shimadzu IR Affinity-1S FTIR (400–4000 cm−1) using KBr as a matrix. Electron spray ionization mass spectroscopy (ESI-MS) was acquired on a Shimadzu LCMS-8040. 1H, and 13C NMR spectra were recorded on a Bruker Advance DRX (400–500 MHz) with deuterated chloroform solvent; and tetramethylsilane (TMS) as the internal standard. CDCl3 (7.26 ppm in 1H and 77.2 ppm in 13C) and chemical shifts are reported in ppm, and multiplicities are shown as s (singlet), d (doublet), t (triplet), q (quartet), dd (doublet of doublet), or br (broad). The absorption measurements of RHBIT, before and after UV irradiation, were done on a JASCO V-650 spectrophotometer. Emission studies and quantum yield measurements were carried out on Fluorolog–Horiba, using a 1 cm path length quartz cuvette with an excitation/emission slit width of 2 nm. Solid UV absorption studies were measured on a JASCO V-670 (UV-VIS NIR) spectrophotometer. Time-resolved fluorescence measurements were recorded using a time-correlated single-photon counting (TCSPC) Horiba DeltaFlex Modular fluorescence system using the instrumental parameters: 375 nm, 510 nm nano LED excitation source, bandpass of 4 nm, peak preset of 10
000 counts; the time resolution was 25 ps. All measurements were performed at room temperature. The effect of pH was studied using a digital pH meter (LI 120 – Elico M) equipped with a glass electrode.
The morphology of drop-casted films for RHBIT on a silicon wafer with gold sputtering was studied under an Oxford X-maxN LEICA EM ACE200 scanning electron microscope. The silicon wafers were washed thoroughly with water and acetone and dried in an oven at 100 °C before use. Thermogravimetric analysis (TGA)-differential thermal analysis (DTA) was carried out on a Shimadzu DTG-60 simultaneous DTA-TG instrument with an increase in temperature at a rate of 10 °C min−1 from 30 °C to 700 °C under an N2 atmosphere. Differential scanning calorimetry (DSC) was recorded on a Shimadzu DSC-60 instrument under similar conditions to those mentioned above with a temperature range of 30 °C to 350 °C.
2.3 Synthesis and characterization
2.3.1 (3′,6′-Bis(diethylamino))-2-(5-nitrobenzo[c]isothiazol-3-yl)spiro[isoindoline-1,9′-xanthen]-3-one; RHBIT. In a 25 mL round-bottomed flask, RHB (0.1 g, 0.2 mmol) was dissolved in 3 mL of DMF at 0 °C under an N2 atmosphere followed by the addition of DIPEA (0.051 g, 0.4 mmol). The reaction was stirred for 10 min, then HOBT (0.054 g, 0.4 mmol) and EDC·HCl (0.076 g, 0.4 mmol) were added and stirred at 0 °C for about 20 min. Later ANB (0.12 g, 0.6 mmol) was added to the resulting mixture and stirred at 20 °C, for another 16 h (ref. 14) (Scheme 1). After completion of the reaction (monitored by TLC), 15 mL of cool water was added to the resultant mixture and left for some time. After 20 min a solid formed at the bottom of the flask, which was filtered through a vacuum and dried in a desiccator. A purple solid was obtained. It was purified by column chromatography on neutral alumina using DCM solvent alone. The final product RHBIT was collected immediately after 2 fractions as a single spot in TLC and was evaporated, resulting in a yellow solid with (0.078 g) 65% yield. Subsequently, the yellow solid was dissolved in a 3
:
2 ratio acetonitrile
:
ethanol mixture, heated at 50 °C for 5–10 minutes, and evaporated slowly at room temperature. Within ∼48 h, clear brownish blocks of RHBIT single crystals could be obtained. mp 290–293 °C, ESI-MS m/z+ calculated for C35H33N5O4S: 619.23, found ([M + H]+) 620 (Fig. S1†); HRMS (EI): C35H33N5O4S [M + H]+ calcd m/z: 619.23; found m/z: 620 (Fig. S2†). IR (νmax/cm−1) as KBr pellets: 2968 (–C–H s), 1716 (–C
O s), 1610 (–C
C s), 1515 (–C–NO2), 1411 (–C–H b), 1335, 1214, 1122 (C–N s), 882, and 815 (–C–S s) cm−1 (Fig. S3†). 1H NMR (400 MHz, CDCl3) δ 8.55 (d, 1H, J = 1.9 Hz, Ar–H), 8.03–8.00 (dd, 1H, Ar–H), 7.97 (dd, 1H, J = 9.7, 2.3 Hz, Ar–H), 7.55–7.47 (m, 3H, Ar–H), 7.15 (dd, 1H, J = 6.4, 1.3 Hz, Ar–H), 6.60 (d, 2H, J = 8.9 Hz, Ar–H), 6.28 (d, 2H, J = 2.5 Hz, Ar–H), 6.22 (dd, 2H, J = 8.9, 2.6 Hz, Ar–H), 3.24 (q, 8H, J = 7.2 Hz, –CH2), 1.06 (t, 12H, J = 7.1 Hz, –CH3) (Fig. S4†). 13C NMR (101 MHz, CDCl3) δ 164.6, 162.1, 158.9, 152.6, 152.4, 148.5, 142.3, 133.4, 127.8, 127.2, 123.3, 123.1, 121.5, 120.8, 120.3, 107.5, 102.6, 97.0, 68.4, 43.3, 11.4 (Fig. S5†). The molecular structure of the cyclic RHBIT was confirmed by single crystal X-ray study with corresponding CCDC No. 2202193.†
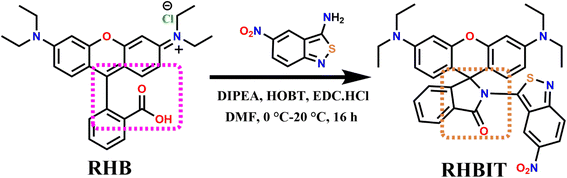 |
| Scheme 1 Facile and single-step synthetic route for the preparation of compound RHBIT. | |
2.4 Crystallographic data and refinement
Selected suitable single crystals of RHBIT were mounted on a Rigaku XtaLAB P200 diffractometer at 100 K with graphite monochromated Cu-Kα radiation (λ = 1.54184 Å). CrysAlisPro (Rigaku Oxford Diffraction) software was used to collect and reduce the data. The Olex2 (ref. 26) with ShelXT27 structure solution program was used to solve the structure, and the ShelXL28 refinement package was used to refine the data. Basic crystallographic data is tabulated in Table S1 (ESI).† Complete crystallographic details are given in the ESI (Tables S2–S7†).
2.5 UV-vis absorption and fluorescence measurements
The stock solution was prepared by weighing 1 mg of RHBIT (100 ppm) dissolved in 10 mL of acetonitrile solvent and further diluted to 25 ppm (40 μM) for absorption and emission studies. For the Job plot experiment, a total concentration of 100 μM of RHBIT and PTSA solutions (where the mole fraction of PTSA varied from 0 to 1) was considered. For the pH study, 500 μL of (25 ppm) RHBIT was taken and mixed with 1500 μL of different pH solutions (pH = 2 to 10), prepared by using standard buffer capsules with pH 4,7 and 9; further adjusted to acidic and basic conditions using small volumes of 2 M HCl or 2 M NaOH solutions. The corresponding absorption and emission spectra were recorded at various pH conditions. Solvatochromic and other sample pictures were captured by a cellphone camera.
2.6 Computational details
The cyclic and open structures of RHBIT were modeled in Gaussview29 and optimized using density functional theory30 in the Gaussian16 software package.31 The optimization and frequency calculations were done using the ωb97XD functional32 and 6-31G(d,p) basis set33 for all atoms. Molecules were optimized without any geometric or symmetry constraint in the gas phase. Analytic frequencies were computed to confirm the minima of all stationary points. The HOMO–LUMO energy difference was calculated from the minimum-energy structure. TDDFT calculations were employed to obtain the vertical excitation energy and oscillator strength (representing the absorption intensity) at the ground-state optimized geometry. The natural transition orbitals were determined using the Multiwfn program.34
3 Results and discussion
RHBIT could be achieved by a facile synthesis in a single step (Scheme 1), by modification of our previously reported procedure.14 The chemical structure was confirmed from spectroscopic data (Fig. S1–S5†), and RHBIT was found to be thermally stable under ambient conditions with a decomposition temperature of ∼294 °C. The explored results are fortified by fluorescence lifetime and quantum yield measurements. The basic conception of acid-sensing is explained by fluorescence-based titration studies. The molecular functionality of RHBIT was confirmed by a new characteristic peak at 1716 cm−1, which corresponds to the carbonyl stretching frequency of lactam, and 2968 cm−1 attributed to the C–H stretching of a methylene group.35 A shift in the wavenumber from 1591 cm−1 in RHB (–C
O) to 1716 cm−1 (–C
O) in RHBIT (Fig. S3†) indicates the formation of spirolactam. The cyclized structure was also supported by a characteristic signal in the 13C NMR spectrum near 68.48 ppm (spiro-carbon) (Fig. S5†), representing the formation of 3° carbon of RHBIT in deuterated chloroform.36 The spirolactam (–C
O) group appeared at δ = 162.09 ppm in the 13C NMR spectrum. The above interpretations clearly confirmed that RHBIT existed in the closed-ring spirolactam form (Scheme 1).
3.1 Crystallography study
A molecular ORTEP diagram with a 50% probability of thermal ellipsoids is shown in Fig. 1(a). A crystallography study of RHBIT reveals the unique spirolactam ring structure at N8–C58–C57; N3–C7–C22 in (Fig. 1(a)); also supported by the characteristic peak of 68.48 ppm (spiro-carbon) in the 13C NMR spectrum (Fig. S5†). RHBIT crystallized in the monoclinic P21 space group. The asymmetric unit (Fig. S6(a)†) contains two complete RHBIT units each with an empirical formula C35H33N5O4S. The angle between the planes of the xanthene ring and the spirolactam moiety is found to be 87.58° (Fig. S6(b)†), slightly twisted when compared to RHB, wherein the angle between the xanthene and the phenyl ring with carboxylic acid functionality is 90° in RHB; while the angle between the planes of the spirolactam unit and the ANB moiety is observed to be 42.54° (Fig. S6(c)†); the angle between ANB and the xanthene ring planes is 81.15° (Fig. S6(d)†). The torsion angle between C6, C7, C22, and C23 is 63.33°; that between C8, C7, N3, and C29 is 67.57°. Despite extensive short contacts (<sum of van der Waals radii) in the range of 2.249 Å to 3.373 Å existing in RHBIT units, prominent short contacts (Fig. 1(b)) were between O2⋯O6 (2.992 Å), C28⋯C63 (3.373 Å), S1⋯O5 (3.190 Å), O1⋯S2 (3.179 Å), H12⋯S2 (2.989 Å), H53B⋯O7 (2.679 Å), C5⋯O6 (3.075 Å), C52⋯H10 (2.764 Å), O2⋯H44 (2.249 Å), H19A⋯H56B (2.357 Å), and C61⋯H19C (2.851 Å). The antiparallel alignment of the RHBIT moiety was noticed along the axes ob and oc, while parallel arrays were observed along the oa axis (Fig. S6(e)†).
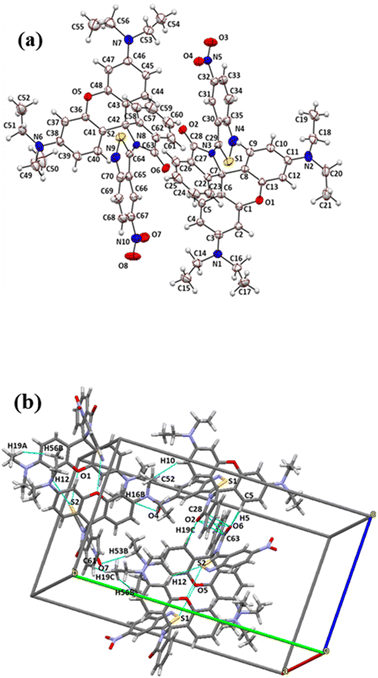 |
| Fig. 1 (a) ORTEP diagram of RHBIT showing 50% probability thermal ellipsoids. (b) Prominent short contacts within the range 2.249 Å to 3.373 Å manifested between the RHBIT molecules. | |
3.2 Effect of pH
Rhodamine spirolactam derivatives are well-known fluorescent pH sensors under acidic conditions via the ring-opening process by protonation. However, RHBIT exhibits very weak absorption and emission in the pH range of 6–10 in the acetonitrile–water system and exists as a spirocyclic form that is colorless and non-fluorescent. As the pH value decreased (<5), an increase in the intensity of the absorption band at λmax ∼ 564 nm was observed and the colorless solution of RHBIT quickly turned pink due to the ring-opening mechanism induced by the proton. Additionally, emission spectra resemble the same response at pH < 5 and show high emission intensity at ∼575 nm wavelength. While increasing the pH > 6 by subsequent addition of NaOH resulted in a colorless solution, very weak emission in alkaline solution at ∼575 nm was observed. This implies reversible coordination between RHBIT and H+ where the spectral and color changes showed that RHBIT can probe pH reversibly, and this detection process is visible to the naked eye. However, the emission wavelength of the probe gradually increased with a ∼19 nm bathochromic shift from 575 nm to 594 nm at pH 2. Also, the fluorescence emission intensity at lower pH (2) was ∼18-fold larger than that at higher pH (10). Moreover, pH-dependent variations were measured to estimate the stability of the spirolactam moiety. Remarkable changes in emission intensity were only perceived below pH 6, and the spirocyclic form of RHBIT was stable in the pH range 6–10 (Fig. S7†). The observed stability of the spirolactam ring should enable RHBIT to be suitable for bioassays since most biofluids have a pH of ∼7.4. Hence, RHBIT can be considered an effective fluorescence “turn-on” sensor for pH detection under more acidic conditions. Interestingly, a similar phenomenon was remarked in a rhodamine 6G hydrazide derivative37 and it was proposed for the detection of pH levels in the human stomach/certain acidic environments.
3.3 Optical studies
3.3.1 Ethanol responsiveness of RHBIT. Initially, the solvatochromic behavior was studied by dissolving RHBIT (25 ppm) in 12 common organic solvents, which demonstrated unique sensitivity with respect to solvent polarities. RHBIT was more soluble in chlorinated solvents, such as chloroform and dichloromethane, compared to polar protic or aprotic solvents. Interestingly, RHBIT solutions were colorless in the rest of the solvents, except that ethanol showed a pink color in ambient light (Fig. 2(a1)), indicative of RHBIT as an ethanol-responsive molecule. To ascertain the reason behind the distinct color change in ethanol; absorption and emission measurements were investigated in 12 different solvents, and the results obtained are shown in Fig. 2. The UV-vis absorption spectra showed absorption peaks of ∼389 nm for the cyclized form of RHBIT in almost all solvents considered, except for ethanol (Fig. 2(a)). In ethanol, along with a 409 nm peak, an apparent sharp intense absorption peak at 560 nm was noted, compared to n-propanol and n-butanol (at 554 nm), which was accountable for the observed pink color in ethanol, suggesting that ethanol is favorable to ring-open species of RHBIT. There is no evident peak in the 560 nm range for aprotic solvents where only a 389 nm (n → π* transition) peak is perceived. At the excitation wavelength of 340 nm, RHBIT exhibits dual emission in all the solvents quoted above (∼400 nm, ∼428 nm); except for ethanol (∼429 nm and ∼589 nm) (Fig. 2(b)). Indeed, the fluorescence spectra display a strong emission band with high intensity at ∼589 nm only in ethanol, verifying the isomerization of closed and open forms in ethanol, owing to the strong hydrogen-bonding interactions of the aminobenzoisothiazole moiety. A similar phenomenon was observed by Bag et al., where a rhodamine-based FRET signaling system with a nitrobenzoxadizole fluorophore amidst an acyclic amino receptor displayed prominent absorption and emission intensities in protic solvents like MeOH, EtOH, and H2O due to the possible H-bonding interactions with the receptor, revealing a key role in the ring-opening process.38 Moreover, Wang et al. reported that (i) the strong polarity of the solvents is favorable for intramolecular charge separation in its ring-open state and further stabilized by strong electron-withdrawing groups; (ii) strong H-bonding interactions of the protic solvents with the amide moiety perhaps giving stability to the ring-open form of cyano-rhodamine spirolactams.39 Generally, a low pKa value hastens the isomerization rate of ring closed and open forms, and high polarity stabilizes the conjugated zwitterion with a large dipole moment.23 The pKa of ethanol is 15.9, and the polarity value is 0.65, closer to the pKa (15.6) and polarity of methanol (0.76), and comparatively higher than other solvents according to CRC Handbook40 (Table 1). The pH value of RHBIT in ethanol is ∼4.4 before exposure to 254 nm UV light, which is under acidic conditions when juxtaposed with other solvents. As mentioned in Section 3.2, highly acidic conditions favor the ring-opening mechanism of spirocyclic RHBIT. Thus, RHBIT manifests good ethanol responsiveness due to its lower pH value and strong H-bonding interactions with ethanol. This is hardly seen in rhodamine derivatives and it exists as a spironolactone (colorless solution) form in aprotic solvents.
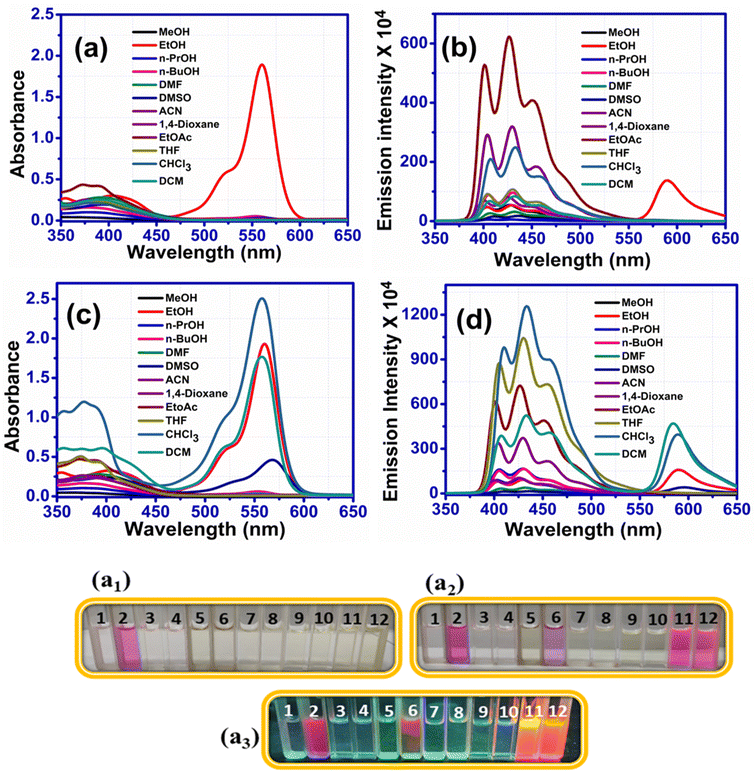 |
| Fig. 2 Absorption and emission spectra of RHBIT in different solvents (40 μM, λex = 340 nm): (1) methanol, (2) ethanol, (3) n-propanol, (4) n-butanol, (5) DMF, (6) DMSO, (7) ACN, (8) 1,4-dioxane, (9) ethyl acetate, (10) THF, (11) CHCl3, (12) DCM; representing (a) absorption and (b) emission spectra before UV irradiation; similarly (c) absorption and (d) emission spectra after UV irradiation. Photographic images taken under (a1) ambient light for RHBIT before UV exposure and (a2) after UV exposure; where (a1) shows RHBIT appearing as pink in ethanol solvent and colorless in the remaining solvents under ambient light. (a3) Presents a photographic image of (a2) taken under short-wavelength UV light (254 nm). (a2) and (a3) depict the color transformation of RHBIT from colorless to pink/orange in DMSO, chloroform, and DCM solvents when observed under short-wavelength UV (254 nm) illumination. | |
Table 1 pH, absorption, and emission maxima and Stokes shift of RHBIT in different solvents before and after exposure to UV light with polarities and pKa values of respective screened common solvents. A blue color indicates variation in pH and wavelength values before and after exposure to short-wavelength UV light (254 nm)
3.3.2 Photoinduced absorbance and fluorescence emission of RHBIT in different solvents. Irradiation of RHBIT in varied solvents under short-wavelength UV (254 nm) light surprisingly led to an instantaneous color change, i.e. from colorless to orange fluorescence, in less than 1 min primarily in chloroform, after 5 min in DCM and ∼20 min in DMSO and remained unchanged (pink color) in ethanol solvent (Fig. 2(a2) and (a3)). Such a color change was not observed in the presence of long-wavelength UV (365 nm) or with other solvents. The absorption and emission spectra were recorded before and after exposure to UV light. The vibronic absorption spectrum of the orange-colored species is very similar to that of the zwitterionic species of RHBIT.18RHBIT showed absorbance at ∼560 nm for protic solvents and no absorbance above ∼450 nm (Fig. 2(a)) before UV light irradiation for aprotic solvents. Fig. 2(b) presents emission spectra before UV irradiation. Interestingly, after UV light (of 254 nm) irradiation for 15–20 min of RHBIT in various solutions, intense absorbance peaks were noticed only in chloroform (∼557 nm), DCM (∼558 nm), and DMSO (∼568 nm) solvents (Fig. 2(c)). The intensity of the absorbance peak in the unirradiated ethanol solution of RHBIT was slightly enhanced (0.04 units) after UV exposure and the absorbance changes after irradiation for CHCl3, DCM, and DMSO correlated well with the color changes (colorless to pink under visible light and short-wavelength UV (254 nm)), which can be noted clearly in the photographs in Fig. 2(a2) and (a3). RHBIT in CHCl3 and DCM manifested strong dual emission at 588 nm and 584 nm, and weak emission at 594 nm in DMSO along with the usual peak at 433 nm, while emission at ∼589 nm for ethanol remains unchanged (Fig. 2(d)). CHCl3 and DCM solutions of RHBIT show a highly emissive strong band at 584 nm due to the longer lifetime and higher quantum yield of the ring-open state. The variation in emission intensity at ∼584–594 nm and the color changes after UV illumination in ethanol, DMSO, CHCl3, and DCM solvents are clearly depicted in Fig. 3, and no considerable change in emission intensity after irradiation was observed in ethanol solvent. These spectral and color changes indicate that RHBIT isomerizes to a zwitterionic form by cleavage of the C–N bond in spironolactone resulting in an open form by a photoinduced ring-opening mechanism along with an extended conjugated structure with strong absorbance and emission intensities.41 Moreover, the electron-withdrawing nitro group attached to the benzenoisothiazole moiety of RHBIT facilitates less conjugation into an extended conjugated structure; also chlorinated solvents like CHCl3 and DCM have a hydrogen-bonding character; they are able to behave as H-bond donors.42 Hence, RHBIT exhibits typical rhodamine spirolactam ring-opening behavior in the presence of acid and also under UV light illumination.20 We have further studied the emission intensity of the newly formed photoinduced products at regular intervals for 48 h. After 48 h, the emission intensity decreased ∼4-fold and 140-fold for CHCl3 (λmax,emi = 588 nm) and DCM (λmax,emi = 584 nm) solutions, respectively (Fig. S8†). In contrast, the emission intensity remains unaltered for ethanol and DMSO and the color of the solutions remains light pink after subsequent storage in the dark, at room temperature for 5 days. In DMSO, the solvent pink color persists even after 2 months. This indicates that the photoresponsive behavior of RHBIT in CHCl3 and DCM was reversible as they returned to their original states and irreversible in the case of DMSO and the color changes persevered for minutes to months, depending upon the solvent medium. After irradiation, the CHCl3 and DCM solutions of RHBIT were subjected to heating at 40–60 °C, and still the pink color of the solutions was retained, suggesting the thermal stability and irreversible photochromic feature of RHBIT. To gain mechanistic insight into the photochemical reaction of RHBIT, the 1H NMR spectra of RHBIT before and after UV exposure were recorded in CDCl3. The 1H NMR spectrum showed a severe broadening of lines and the splitting pattern was unclear with UV light irradiation compared to that before irradiation (Fig. S9†). The color of the solution changed from yellow to prominent pink in deuterated CDCl3 after short-wavelength UV illumination. From the spectral behavior, it is presumed that photoisomerization takes place from the closed ring to the open form41 of RHBIT and a plausible mechanism for the photoinduced ring-opening process is proposed in Scheme 2. Variation of pH, quantum yield, and lifetime decay in the above-mentioned solvents, studied before and after UV irradiation, disclosed that pH in CHCl3, DCM, and DMSO was reduced to an acidic range (3.3–5.5) (Table 1), which as mentioned before is evocative of strong protonation of the spirocyclic form of RHBIT, which further transforms to an open form in an acidic environment. Moreover, variation in pH was not observed for the remaining solvents before or after UV illumination.
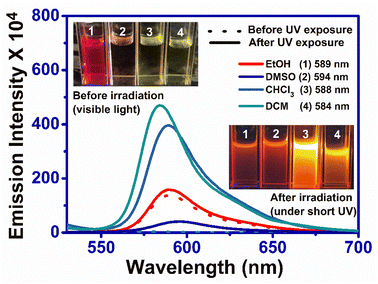 |
| Fig. 3 Enhancement in emission intensity of RHBIT at ∼584–594 nm in ethanol, DMSO, CHCl3, and DCM after exposure to short-wavelength UV light (254 nm), showing intense orange emission in the case of chloroform and DCM. Inset shows a change in color variation before irradiation (photograph taken in visible light) and after exposure to UV (photograph taken in short-wavelength UV light). | |
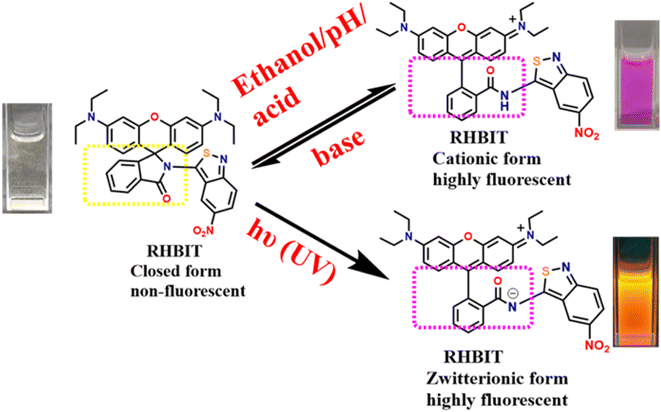 |
| Scheme 2 Plausible mechanism of closed and open forms of RHBIT by external stimulation. | |
The optical band gap of solid RHBIT was calculated using a Tauc plot before and after UV light irradiation and they were found to be similar, ∼4.7 eV (before UV exposure) and 4.6 eV (after UV exposure) (Fig. S10(a) and (b)†), suggesting an insulating property of RHBIT. The absorption wavelength of solid RHBIT occurred at 433 nm along with a weak charge-transfer band at ∼1681 nm, representing the weak charge-transfer interactions of ANB with the phenyl group of the xanthene moiety. Similar responses in the solid RHBIT suggested no photochemical reaction happening (Fig. S10(c)–(e)†). No prominent characteristic emission peak was observed.
The RHBIT molecule adopts two different isomers, with its ANB ring intact and with the ring-open form. The latter form is predominantly observed in an acidic medium. The highest occupied molecular orbital (HOMO) and the lowest unoccupied molecular orbital (LUMO) energies of both the forms of RHBIT are given in Fig. 4. The HOMO–LUMO energy gap decreases from 6.3 eV to 5.9 eV when the cyclized form changes to the open form. The strain in the cyclized form of RHBIT increases the energy of the individual frontier orbitals and also the HOMO–LUMO energy gap.
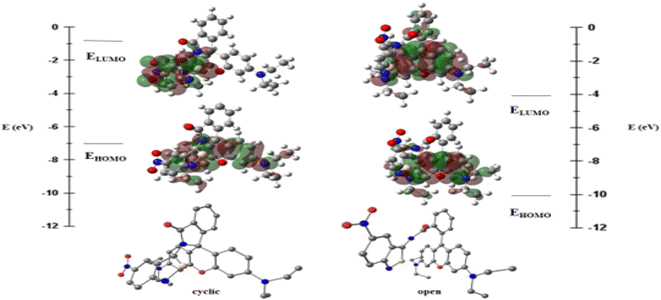 |
| Fig. 4 The HOMO–LUMO energy diagram of the cyclized and open RHBIT system. The optimized structures, the calculated HOMO and LUMO energy levels, and the HOMO–LUMO energy gaps (in units of eV) are presented for cyclized and open forms. | |
Natural transition orbital43,44 (NTO) analysis was performed for thirty electronic excitations in the UV visible region from the ground state with the TDDFT method. In the cyclic form, the most intense band (corresponding to the TDDFT transition with the highest oscillator strength) is calculated for the excited state where the electronic transition is from HOMO−2 → LUMO+5 with a wavelength of 214 nm. The NTOs show that the electronic transitions are a combination of π–π* from the RHB moiety along with charge transfer from the RHB moiety to the NO2 group of the ANB moiety, as shown in Fig. 5(a). This agrees well with the low-wavelength band in the absorbance data of the RHBIT system with most of the solvents. The S0 → S1 excitation in the cyclic RHBIT is calculated at 401 nm, corresponding to the HOMO–LUMO transition, with zero oscillator strength (Fig. 5(b)). The corresponding NTO shows a charge transfer between π–π* of the RHB moiety to the ANB group of RHBIT. This forbidden transition at 401 nm confirms the absence of the high-wavelength band of the RHBIT system with most of the solvents. Unlike in the cyclic form, the most intense excitation in the open form of RHBIT is calculated for the S0 → S1 transition with a wavelength of 424 nm. From the NTOs, we observe that the electronic transitions involve locally excited π–π* transition from the RHB moiety, as shown in Fig. 5(c). This high-wavelength low-energy band also agrees well with the absorbance data.
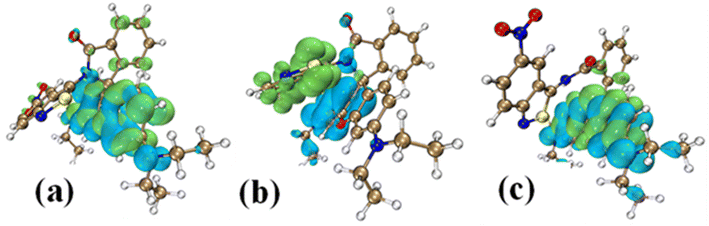 |
| Fig. 5 The natural transition orbital (green: hole and cyan: electron) associated with the electronic excitation of cyclic RHBIT at (a) 214 nm and (b) 401 nm (with zero oscillator strength), and that of ring-open RHBIT at (c) 424 nm. | |
3.3.3 Lifetime and quantum yield measurements. Fluorescence intensity enhancement motivated us to examine fluorescence decay through excited-state lifetime studies. The resultant decay components before (Fig. S11(a)†) and after UV irradiation (Fig. S11(b) and (c)†) were analyzed as bi-exponential functions by adjusting the chi-squared value between 0.8 and 1.2. The decay parameters are listed in Table S8 (ESI†). The fluorescence lifetime decays of RHBIT in ethanol at ∼589 nm before and after UV exposure were alike (1.16 ns and 1.11 ns) (Fig. S11(c)†), suggesting that the decay originated from a similar excited state. In contrast, the lifetime values for CHCl3, DCM, and DMSO (∼584–588 nm) solutions of RHBIT after UV irradiation had slightly higher values, i.e., 2.4 ns, 3.4 ns, and 1.1 ns (Fig. S11(c)†), compared to the closed form i.e. before UV exposure (1.26 ns, 1.28 ns, 0.78 ns); these results suggest that the short-wavelength UV irradiation increases the lifetime values, which enhances the stability of the xanthylium cation representing the ring-opened state of RHBIT.45 Hence, the accomplished results agreed with the steady-state fluorescence spectral data. The equations used for calculating average lifetime, radiative (kr) and non-radiative (knr) decay constants, and quantum yield are provided in the ESI;† the values are tabulated in Table S8.† It is evident that the radiative decay constant and luminescence quantum yield of RHBIT in CHCl3, DCM, and DMSO increase drastically after UV illumination; non-radiative decay values decrease but remain similar for the other solvents (Table S8†), in accordance with the variation trends of fluorescence intensities. Thus, an enormous enhancement in emission intensity is principally due to increased radiative decay values compared to radiationless relaxation.46 The above results indicate the photoisomerization between fluorescence off and on states of RHBIT in the different solvents considered in the study.
3.3.5 Protonation of RHBIT with p-toluene sulfonic acid. To a greater extent, the switching between the closed ring and open form of RHBIT was demonstrated by sensing different acids like HCl, H2SO4, HNO3, benzoic acid, 4-chloro,3-nitrobenzoic acid, 4-aminobenzoic acid, anthranilic acid and p-toluene sulfonic acid (PTSA) through protonation of the nitrogen atom in the amide linkage. 40 μM of RHBIT in acetonitrile was treated with 50 μM of different acids, and the relevant absorbance, emission spectra were recorded (Fig. S12†). Characteristic absorption and emission peaks were not found in RHBIT above 500 nm; representing a non-fluorescent cyclized form. However, after the addition of the above-specified acids, strong absorption and emission peaks originated at 560 nm and ∼585 nm, respectively, except for 4-aminobenzoic acid, and anthranilic acid with weak emission intensity. Among these, the emission intensity of PTSA with RHBIT at 585 nm was enhanced ∼150-fold with respect to RHBIT. Furthermore, sensing studies were carried out with disparate concentrations of PTSA in acetonitrile without changing the concentration of the probe (40 μM). For each titration, 1800 μL of the probe and 200 μL of PTSA with concentrations of 10 to 120 μM were added to a 3 mL quartz cuvette. Absorption spectra revealed that λmax absorption is at 560 nm; (Fig. 6(a)); on excitation at 520 nm, an orange-red emissive peak at 585 nm was noticed for the open form of RHBIT, and simultaneously the intensity of the probe increased with an increase in the concentration of PTSA (Fig. 6(b)), designated to the extended π-conjugated planar structure of the open form. This results in complex formation by donating a proton from the sulfonic acid group of PTSA to the amide linkage of RHBIT, promoting the ring-opening mechanism. Recently, we realized that benzoisothiazole forms a simple protonated hydrogen-bonding complex with PTSA.25 Also, herein, an enhanced emission intensity at 585 nm manifested a good linear relationship with the concentration of PTSA in the ∼45 to 61 μM range, as shown in the inset of Fig. 6(b); the bright color change of the probe from colorless to prominent pink was pronounced in Fig. 6(c)–(e).
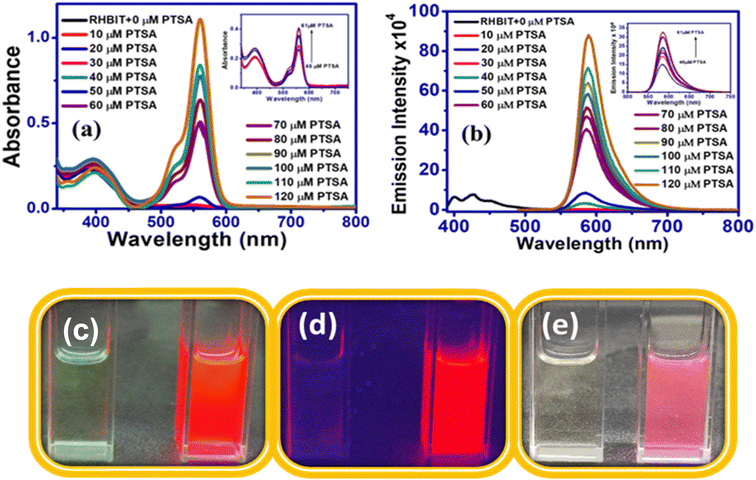 |
| Fig. 6 The (a) absorption and (b) emission spectra of RHBIT (40 μM) on titration with different concentrations of PTSA (0–120 μM), depicting an increase in intensity with an increase in concentration. The inset figures represent an increase in intensities of absorbance and emission in the 45–61 μM range. (c), (d) and (e) display the colorimetric responses of pure RHBIT (left) and after adding PTSA (right) in short-wavelength UV, long-wavelength UV, and visible light, respectively. | |
Moreover, the binding mechanism of RHBIT with PTSA is supported by FT-IR (Fig. S13(a)†) and 1H NMR spectra. A shift in the carbonyl (C
O) group stretching frequency was observed from 1716 cm−1 (RHBIT) to 1591 cm−1 (RHBIT–PTSA). The lower frequency shift resembles the stretching frequency of C
O in rhodamine B, indicating the open-ring form of rhodamine spirolactam through protonation by PTSA. To gain better insight into the ring-opening mechanism, we accomplished an 1H NMR titration experiment of RHBIT in CDCl3 using a continuous titration method by adding different volumes (10–100 μL) of PTSA in DMSO-d6. The peak shape of RHBIT becomes broadened and a slight downfield shift of the aliphatic and aromatic protons was observed. Both methyl and aromatic protons of PTSA were also recognized in the spectra after adding 100 μL of PTSA. A new signal at δ = ∼11.4 ppm was found, suggesting that the signal is due to the –N–H proton of the amide functionality, indicating the opening of RHBIT in the presence of PTSA (Fig. 7). The switching between closed and open forms of RHBIT with PTSA can be seen visually by a prominent color change to pink (Fig. 7), which clearly supports the involvement of the amide carbonyl oxygen in the ring-opening mechanism.45 From the above observation, a plausible mechanism for the proton-induced ring-opening process in RHBIT is proposed in Scheme 2. Several attempts to crystallize RHBIT–PTSA were in vain. Acetonitrile solutions of RHBIT and PTSA at a ratio of 1
:
1 were mixed and heated gently at 50 °C and kept for evaporation at room temperature; after 3 days pale brown-colored crystals were formed at the bottom, while the supernatant solution was pink (Fig. S13(b)†), consistent with the spirocyclic form of RHBIT. A similar observation was reported by Fogerty et al. when they attempted to crystallize rhodamine B phenylene diamine dimer with HCl.20
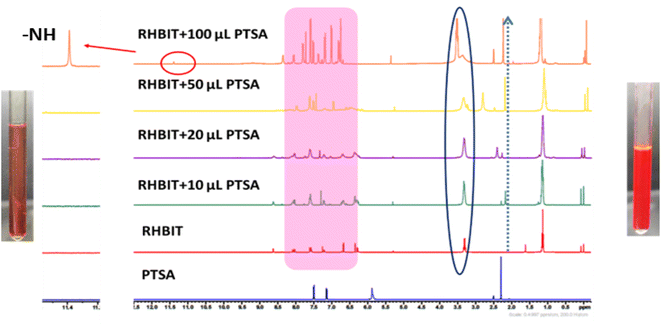 |
| Fig. 7 The 1H NMR titration spectra of RHBIT with an increase in the concentration of PTSA. The variation in signals after the addition of PTSA is marked on the spectrum. Inset pictures show the color transformation (left) RHBIT after adding PTSA, (right) RHBIT–PTSA. | |
3.3.4.1 Limit of detection and binding stoichiometry of RHBIT with PTSA. The sensitivity of RHBIT towards PTSA has been determined by estimating the limit of detection (LOD), as shown in Fig. 8(a). The limit of detection was calculated by the following method (LOD = 3σ/K),14 where σ is the standard deviation for blank measurement (σ = 72.81) and K is the slope of linear fitting lines of the titration data. To determine σ, the emission intensity values of the blank solutions were each measured thrice. The LOD value for RHBIT towards PTSA was calculated as 23 nM, suggesting that RHBIT is highly sensitive towards PTSA through the ring-opening mechanism via protonation. The binding stoichiometry between RHBIT and PTSA was evaluated by Job's method (Fig. 8(b)). A Job plot was constructed by considering the changes in absorption intensity (Y-axis) at 560 nm as a function of mole fraction (X-axis) of PTSA after adding it to RHBIT and the binding stoichiometry between RHBIT and PTSA was found to be 1
:
1.
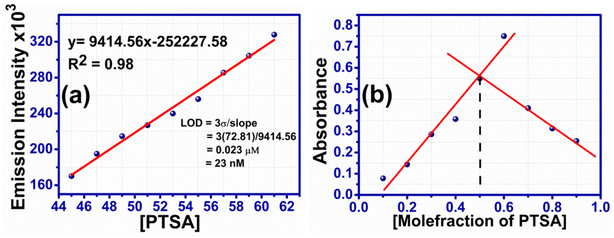 |
| Fig. 8 (a) Limit of detection limit of RHBIT after the addition of PTSA considering the linear range from 45 to 61 μM. (b) Job's plot of RHBIT with PTSA, indicating 1 : 1 binding ratio. | |
Reversibility and regeneration of chemosensors play a key role in practical and bioimaging applications. Herein, to demonstrate the reversibility of RHBIT (25 ppm) in acetonitrile, PTSA (25 ppm) in acetonitrile and triethyl amine were introduced to the probe solution and the respective emission spectra were recorded. As mentioned above, RHBIT was non-fluorescent and colorless. When PTSA was added, the probe formed strong complexation with PTSA through protonation, and fluoresced highly at ∼592 nm, exhibiting a visually pink color. Later, triethylamine (TEA) (20 μL) was added to the solution containing the probe and PTSA. The experimental results showed that emission intensity was quenched at ∼592 nm with the disappearance of the pink color due to the decomplexation of RHBIT by TEA, returning it to the ring-closed form (Fig. 9(a)). A similar experiment was executed after short-wavelength UV light irradiation of RHBIT in chloroform. Before UV exposure RHBIT shows an emission peak at ∼433 nm representing a cyclized form; after irradiation at 254 nm UV light it shows an orange emission peak at ∼585 nm, signifying the conversion of the cyclized form to the open-ring form. Upon further addition of small aliquots of TEA, the emission intensity was quenched at ∼585 nm and there was a simultaneous increase in the emission peak at 433 nm, suggesting return of the open-ring form back to spirolactam. In the current situation, TEA push–pulls the open ring to the cyclized form (Fig. 9(b)). Therefore, RHBIT could be considered a potentially reversible chemosensor,46 and, since switchable color changes could be visualized with the naked eye (Fig. 9), it could perhaps employed for information protection applications.
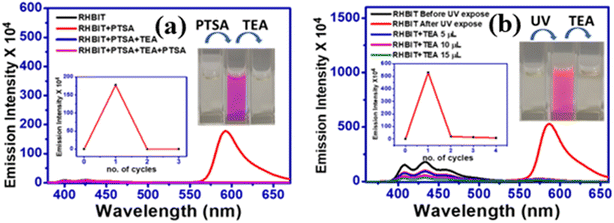 |
| Fig. 9 Changes in the emission intensity of RHBIT (40 μM) in ACN (1500 μL): (a) upon alternate addition of a fixed amount of PTSA (500 μL) and TEA (20 μL); (b) upon the addition of different volumes of TEA (5, 10, 15 μL) to RHBIT (2000 μL) after UV irradiation. Insets in (a), (b) show the reversibility along with the fluorescence response vs. no. of cycles, performed at an emission wavelength 585 nm and the colorimetric response of RHBIT. | |
3.4 Thermal analysis and scanning electron microscopy images
The thermal stability of RHBIT was determined by thermogravimetric analysis (TGA) and DSC differential scanning calorimetry (DSC) (Fig. S14†). TGA analysis reveals that RHBIT was moisture free and thermally stable up to 294 °C; it then decomposed in a single step with a significant weight loss of 52.2%, corresponding to a mass of 323 g from the total mass of 619 g. A gradual weight loss eventuates when the temperature exceeds 294 °C (Fig. S14(a)†). This might be due to the thermal decomposition of two diethyl amino groups and the 3-nitro,benzoisothiazole moiety in RHBIT.47 Finally, the residual mass after the decomposition process was ∼47.8% i.e., 296 g corresponding to the xanthene moiety with the carbonyl phenyl group. DSC analysis (Fig. S14(b)†) illustrates a sharp melting peak at ∼293 °C, indicating the purity and thermal stability of RHBIT. The decomposition temperature from TGA and melting point from DSC were in good agreement. To gain insight into the morphology, 25 ppm of RHBIT in acetonitrile, isopropanol, n-butanol and DMF solutions were each separately drop-cast on silicon wafers and dried at room temperature for 48 hours. The morphology in acetonitrile solution depicted flowers at 5 μm magnification, while bent petal-like structures were observed at 20 and 100 μm (Fig. S15†). Aggregates were noted in i-propanol, n-butanol and DMF solutions at 1 μm. Incompatible morphologies could probably be due to different rates of solvent evaporation. Consequently, the molecular interactions with solvent/diffusion capability result in molecular assemblies and emerge in different growth kinetics.47
3.5 Application of RHBIT as a rewritable platform
The fluorescence switching properties with magnificent bright color changes inspire the use of these materials as a platform for reusable optical reading and to detect base analytes. To further confirm the photoresponsive property and reversibility of RHBIT, we distributed a chloroform/DCM solution of RHBIT on a small piece of paraffin film placed on a glass slide and wrote “BITS” (the short form of the name of our institute) with a small capillary on the film, as shown in Fig. 10(a). Then the film was exposed to short-wavelength UV light for 5 min by placing it in a UV chamber. A swift intense color change was observed from “colorless to prominent pink” in the word “BITS”. This represents a ring-open form of RHBIT after irradiation by 254 nm short-wavelength UV light. The pink letters were erased by overwriting them with TEA. A similar phenomenon was observed on a flower-shaped cut paraffin film. The flower was placed on a Petri dish, and RHBIT chloroform solution dropped on it. The flower film emits a visually intense pink color under short-wavelength UV light (254 nm). The film was preserved on a Petri dish and dripped with TEA; consequently, “pink changes to colorless” (Fig. 10(b)). This scrutiny reveals the visible transformations of closed–open–closed isomerization of spirocyclic RHBIT. Thus, a striking and rewritable bright color change is easily seen in very affordable small molecules. Likewise, different patterns can be printed and easily erased on paraffin films, indicating that RHBIT can serve as a security ink for photopatterning, smart labels, indicators, packing materials for laboratory purposes, and medical diagnostics.
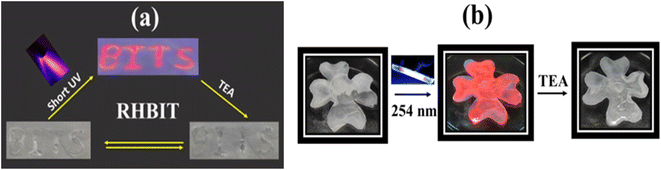 |
| Fig. 10 Demonstration of writing and erasing on paraffin film: (a) writing and erasing process carried out on a film with chloroform solution of RHBIT and TEA under 254 nm UV light. (b) Images of flower-shaped film and its color appearance of RHBIT after 254 nm short-wavelength UV exposure followed by dropping of TEA. | |
The switching between on/off states of RHBIT by acid/base treatment is also achieved by TLC-coated strips. A silica-coated TLC plate was dipped in RHBIT chloroform solution and taken out, and the color of the plate turned pink (Fig. 11). The acidic nature of the TLC silica strips induces protonation of the spirolactam nitrogen group, further leading to simultaneous ring-opening and a transition from the colorless cyclized form to the pink-colored open-state form.48 Furthermore, the pink-colored TLC plate, when dipped in or exposed to TEA turns colorless. TEA deprotonated the ring-open form and returned it to the colorless spirocyclic form. Therefore, RHBIT could be successfully applied as a fluorescent turn-on sensor on paraffin film and TLC plates.
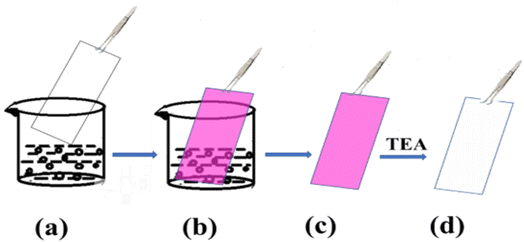 |
| Fig. 11 Schematic representation of silica-coated TLC plates (a) dipped in chloroform solution of RHBIT. (b) and (c) The TLC plate turned a pink color after dipping in chloroform solution. (d) The TLC plate change to colorless when dipped in/exposed to TEA. | |
4 Conclusion
In summary, we report the successful synthesis and detailed characterization of a novel multi-functional (multi-stimuli-responsive) molecular material RHBIT via fusing low-cost RHB with ANB. RHBIT showcased selective ethanol/pH responsiveness, acidochromism, and UV light stimulation; evidenced by off–on switching and emission color changes by the naked eye/under UV light. A single-crystal X-ray diffraction study of RHBIT reveals a spirolactam ring structure. Short-wavelength UV light (254 nm) stimulates the RHBIT into a ring-open form, mostly in chlorinated solvents like CHCl3, DCM, and DMSO (the color persists even after 2 months) with long lifetimes. The DFT and TDDFT calculations support the absorption properties of the closed and open forms. The acid chromic behavior of RHBIT and its reversible switching on–off nature with acid/base could be exploited for information protection applications. RHBIT exhibited high sensitivity toward p-toluene sulfonic acid with a limit of detection of 23 nM and shows 1
:
1 binding stoichiometry between RHBIT and PTSA. The outcome of this work reveals that the fusion of RHB with a fused heterocycle ring accompanied by an electron-withdrawing substituent and the primary amino group could provide a new strategy to accomplish a broad approach for the development of multi-stimuli-responsive molecular fluorescent switches.
Author contributions
Himabindu Battula: Investigation, Methodology, Data curation, Writing-rough draft. Moromi Nath: DFT, TD-DFT calculations, Formal analysis, Documentation. Sabyashachi Mishra: DFT, TD-DFT calculations, Write up, Editing of computational study. Subbalakshmi Jayanty: Supervision, Writing-original draft, review and editing, Investigation, Methodology, Formal analysis, Crystallography, validation, Funding acquisition, Project administration, and organization.
Conflicts of interest
The authors declare no conflict of interest.
Acknowledgements
SJ thanks SERB-DST (Reference No. DST-EMR/2016/002209) Govt. India for the financial support. The authors gratefully acknowledge the Central Analytical Laboratory (CAL) facility provided by the BITS-Pilani, Hyderabad campus. The DST-FIST grant facility is also highly accredited. M. N. acknowledges IIT Kharagpur for fellowship. S. M. acknowledges SERB, DST, Government of India (SR/FST/CSII-026/2013). This work employed the resources of the Paramshakti supercomputing facility of IIT Kharagpur established under the National Supercomputing Mission of the Government of India and supported by CDAC, Pune.
References
- W. Du, X. Liu, L. Liu, J. W. Y. Lam and B. Z. Tang, ACS Appl. Polym. Mater., 2021, 3, 2290–2309 CrossRef CAS.
- Y. Li, Q. Peng, S. Li, C. Yang, J. He, Q. Hu and K. Li, Dyes Pigm., 2019, 171, 107750–107756 CrossRef.
- L. Wang and Q. Li, Chem. Soc. Rev., 2018, 47, 1044–1097 RSC.
- M. K. Lee, P. Rai, J. Williams, R. Twieg and W. E. Moerner, J. Am. Chem. Soc., 2014, 136, 14003–14006 CrossRef CAS PubMed.
- S. K. Patel, J. Cao and A. R. Lippert, Nat. Commun., 2017, 8, 15239–15247 CrossRef CAS PubMed.
- M. Ansari, R. Bera, S. Mondal and N. Das, ACS Omega, 2019, 4, 9383–9392 CrossRef CAS PubMed.
- P. Agostinis, K. Berg, K. A. Cengel, T. H. Foster, A. W. Girotti, S. O. Gollnick, S. M. Hahn, M. R. Hamblin, A. Juzeniene, D. Kessel, M. Korbelik, J. Moan, P. Mroz, D. Nowis, J. Piette, B. C. Wilson and J. Golab, Ca-Cancer J. Clin., 2011, 61, 250–281 CrossRef PubMed.
- Y. Zhu, C. Gu, Y. Miao, B. Yu, Y. Shen and H. Cong, J. Mater. Chem. B, 2019, 7, 6576–6584 RSC.
- A. Li, N. Chu, L. Huang, L. Han, Y. Geng, S. Xu, L. Pan, H. Cui, W. Xu and Z. Ma, Dyes Pigm., 2019, 162, 831–836 CrossRef CAS.
- K. Zheng, Q. Zou, Y. Yang, Y. Mao, J. Zhang and J. Cheng, Ind. Eng. Chem. Res., 2018, 57, 13283–13290 CrossRef CAS.
- M. Natali and S. Giordani, Chem. Soc. Rev., 2012, 41, 4010–4029 RSC.
- G. Berkovic, V. Krongauz and V. Weiss, Chem. Rev., 2000, 100, 1741–1754 CrossRef CAS PubMed.
- V. W. W. Yam, J. K. W. Lee, C. C. Ko and N. Zhu, J. Am. Chem. Soc., 2009, 131, 912–913 CrossRef CAS PubMed.
- H. Battula, S. Muduli, S. Priyanka Bandi, S. Kapoor, S. Mishra, H. Aggarwal, V. Vamsi Krishna Venuganti and S. Jayanty, J. Photochem. Photobiol. Chem., 2022, 426, 113748–113766 CrossRef CAS.
- H. Battula, S. Bommi, Y. Bobde, T. Patel, B. Ghosh and S. Jayanty, J. Photochem. Photobiol., 2021, 6, 100026–100038 CrossRef.
- M. Mathivanan, B. Tharmalingam, O. Anitha, C. H. Lin, V. Thiagarajan and B. Murugesapandian, Mater. Chem. Front., 2021, 5, 8183–8196 RSC.
- Y. Li, Z. Feng, Y. Li, W. Jin, Q. Peng, P. Zhang, J. He and K. Li, Spectrochim. Acta Mol. Biomol. Spectrosc., 2020, 230, 118069–118076 CrossRef CAS PubMed.
- K. H. Knauer and R. Gleiter, Angew. Chem., Int. Ed., 1977, 16, 113 CrossRef.
- J. Fölling, V. Belov, R. Kunetsky, R. Medda, A. Schoenle, A. Egner, C. Eggeling, M. Bossi and S. W. Hell, Angew. Chem., Int. Ed., 2007, 46, 6266–6270 CrossRef PubMed.
- B.-F. C. Stratton, A. J. Pierre, E. A. Riser, N. J. Grinalds, C. W. Edwards, A. M. Wohlwend, J. S. Bauer, R. J. Spera, L. S. Pferdmenges, K. M. Griffith, B. W. Hunter, P. Bobadova-Parvanova, C. S. Day, P. M. Lundin and K. H. Fogarty, J. Phys. Chem. A, 2022, 126, 4211–4220 CrossRef CAS PubMed.
- B. Li, U. Haris, M. Aljowni, A. Nakatsuka, S. K. Patel and A. R. Lippert, Isr. J. Chem., 2021, 61, 244–252 CrossRef CAS.
- W. L. Czaplyski, G. E. Purnell, C. A. Roberts, R. M. Allred and E. J. Harbron, Org. Biomol. Chem., 2014, 12, 526–533 RSC.
- L. Huang, Y. Qiu, C. Wu, Z. Ma, Z. Shen and X. Jia, J. Mater. Chem. C, 2018, 6, 10250–10255 RSC.
-
(a) T. Kaleemullah, M. Ahmed and H. K. Sharma, J. Chem. Pharm. Res., 2012, 4, 483–490 CAS;
(b) G. E. Taylor, M. Gosling and A. Pearce, J. Chromatogr., A, 2006, 1119, 231–237 CrossRef CAS PubMed.
- H. Battula, S. A. Nahata, L. D. Patnaik, S. Ranga and S. Jayanty, J. Mol. Struct., 2021, 1225, 129090–129100 CrossRef CAS.
- L. J. Bourhis, O. v. Dolomanov, R. J. Gildea, J. A. K. Howard and H. Puschmann, Acta Crystallogr. A, 2015, 71, 59–75 CrossRef CAS PubMed.
- G. M. Sheldrick, SHELXTL. Version 6.10, Bruker AXS Inc., Madison, Wisconsin, USA, 2000 Search PubMed.
- G. M. Sheldrick, SHELXS97 and SHELXL97, University of Göttingen, Germany, 1997 Search PubMed.
- R. Dennington, T. A. Keith, and J. M. Millam, Gaussview Version 6, Semichem Inc., Shawnee Mission KS, 2019 Search PubMed.
- R. G. Parr, Density functional theory of atoms and molecules, in Horizons of quantum chemistry, Springer, 1980, pp. 5–15 Search PubMed.
- M. J. Frisch, G. W. Trucks, H. B. Schlegel, G. E. Scuseria, M. A. Robb, J. R. Cheeseman, G. Scalmani, V. Barone, G. A. Petersson, H. Nakatsuji, X. Li, M. Caricato, A. V. Marenich, J. Bloino, B. G. Janesko, R. Gomperts, B. Mennucci, H. P. Hratchian, J. V. Ortiz, A. F. Izmaylov, J. L. Sonnenberg, D. Williams-Young, F. Ding, F. Lipparini, F. Egidi, J. Goings, B. Peng, A. Petrone, T. Henderson, D. Ranasinghe, V. G. Zakrzewski, J. Gao, N. Rega, G. Zheng, W. Liang, M. Hada, M. Ehara, K. Toyota, R. Fukuda, J. Hasegawa, M. Ishida, T. Nakajima, Y. Honda, O. Kitao, H. Nakai, T. Vreven, K. Throssell, J. A. Montgomery Jr, J. E. Peralta, F. Ogliaro, M. J. Bearpark, J. J. Heyd, E. N. Brothers, K. N. Kudin, V. N. Staroverov, T. A. Keith, R. Kobayashi, J. Normand, K. Raghavachari, A. P. Rendell, J. C. Burant, S. S. Iyengar, J. Tomasi, M. Cossi, J. M. Millam, M. Klene, C. Adamo, R. Cammi, J. W. Ochterski, R. L. Martin, K. Morokuma, O. Farkas, J. B. Foresman and D. J. Fox, Gaussian 16 Revision C.01, Gaussian Inc., Wallingford CT, 2016 Search PubMed.
- J. da Chai and M. Head-Gordon, Phys. Chem. Chem. Phys., 2008, 10, 6615–6620 RSC.
- M. J. Frisch, J. A. Pople and J. S. Binkley, J. Chem. Phys., 1984, 80, 3265–3269 CrossRef CAS.
- T. Lu and F. Chen, J. Comput. Chem., 2012, 33, 580–592 CrossRef CAS PubMed.
- A. Pipattanawarothai and T. Trakulsujaritchok, Dyes Pigm., 2020, 173, 107946–107955 CrossRef CAS.
- F. Ali, S. Saha, A. Maity, N. Taye, M. K. Si, E. Suresh, B. Ganguly, S. Chattopadhyay and A. Das, J. Phys. Chem. B, 2015, 119, 13018–13026 CrossRef CAS PubMed.
- M. Tian, X. Peng, J. Fan, J. Wang and S. Sun, Dyes Pigm., 2012, 95, 112–115 CrossRef CAS.
- B. Bag and A. Pal, Org. Biomol. Chem., 2011, 9, 915–925 RSC.
- H. Li, H. Guan, X. Duan, J. Hu, G. Wang and Q. Wang, Org. Biomol. Chem., 2013, 11, 1805–1809 RSC.
- R. C. Weast, Handbook of Data on Organic Compounds, Vol. I and II, CRC Press, Boca Raton/Florida, 1985 Search PubMed.
- M. Tanioka, S. Kamino, N. Koga and D. Sawada, J. Mater. Chem. C, 2020, 8, 543–549 RSC.
- A. Jordan, P. Stoy and H. F. Sneddon, Chem. Rev., 2021, 121, 1582–1622 CrossRef CAS PubMed.
- R. L. Martin, J. Chem. Phys., 2003, 118, 4775–4777 CrossRef CAS.
- R. Zhou and Y. Kanai, J. Chem. Phys., 2021, 154, 054107–054115 CrossRef CAS PubMed.
- A. Dhara, N. Guchhait and S. K. Kar, J. Fluoresc., 2015, 25, 1921–1929 CrossRef CAS PubMed.
- H. Lin, X. Chang, D. Yan, W. H. Fang and G. Cui, Chem. Sci., 2017, 8, 2086–2090 RSC.
- A. Syed, S. Mishra and S. Jayanty, J. Fluoresc., 2022, 32, 115–124 CrossRef CAS PubMed.
- H. F. Nour and T. el Malah, New J. Chem., 2020, 44, 6068–6074 RSC.
|
This journal is © The Royal Society of Chemistry 2023 |