DOI:
10.1039/D2RA07916J
(Paper)
RSC Adv., 2023,
13, 3181-3185
Serendipitous synthesis of cross-conjugated dienes by cascade deconstructive esterification of thiomorpholinone-tethered alkenoic acids†
Received
11th December 2022
, Accepted 10th January 2023
First published on 23rd January 2023
Abstract
Functionalized 1,3-dienes are ubiquitous structural motifs in biologically pertinent molecules. They are frequently employed as precursors for a broad range of chemical transformations, including Diels–Alder reactions. The stereoselective construction of highly decorated 1,3-dienes therefore represents an important research objective. Medicinal chemists are becoming increasingly interested in synthetic methodologies that not only achieve expedient construction and peripheral editing of heterocycles, but also seek to modify their core framework in order to achieve skeletal remodeling. In a succinct manifestation of this ‘scaffold hopping’ concept, we herein describe a cascade reaction, which converts thiomorpholinone-tethered alkenoic acids to 1,1-disubstituted amino-1,3-dienes. This domino process involves esterification of the acid, base-assisted ring-opening, and concomitant 1,2-migration of the α-amino alkenyl group. Several control experiments have revealed that the alkenyl substituent is necessary for deconstruction to occur. Inherently more activated N-aryl-substituted thiomorpholinone acids react significantly faster than their less activated N-alkyl congeners.
Functionalized 1,3-dienes are omnipresent scaffolds in biologically pertinent molecules and also feature as precursors for a broad range of chemical transformations.1 Examples include metathesis, ene reactions, reductive aldolization, or cycloaddition. Specifically, the increased reactivity of amino-substituted 1,3-dienes in the heteroatom-assisted Diels–Alder reaction allows for the facile preparation of functional arrays, which would otherwise be difficult to obtain through conventional modes of reactivity.2 Indeed, bimolecular and intramolecular Diels–Alder reactions of 1-N-acylamino-1,3-dienes have been well-studied and typically proceed with a high degree of regioselectivity and facial selectivity.3 It is now fully appreciated that the alkene geometry influences the stereochemical outcome and the efficiency of reactions involving 1,3-dienes.4 Hence, several synthetic methods have been developed for the stereoselective construction of substituted 1,3-dienes.5
Sulfur-containing compounds are highly prevalent in natural and various biological systems.6 The divalent sulfur atom introduces a metabolic liability from a drug discovery standpoint.7,8 Specifically, the 1,4-thiomorpholine moiety has been widely encountered in a variety of bioactive products including DPP-IV inhibitors,9 anti-inflammatory drugs,10 antimycobacterial agents,11 and hypolipidemic compounds.12–15 We previously revealed that base-assisted methyl esterification of N-alkyl thiomorpholinone acids of type 1 (Fig. 1A) at room temperature, followed by Vilsmeier–Haack functionalization afforded dihydro-1,4-thiazines such as 2.16 During the course of the aforementioned studies, when a thiomorpholinone acid bearing a bulky tert-butyl group (i.e., 3a), was methylated at 50 °C, the expected lactam ester was not observed. Instead, ring-opened product 4a was obtained (Fig. 1B). Notably, 4a contains the β-enamino ester subunit, which is a well-recognized synthon for accessing azaheterocyclic architectures such as indoles, quinolines, pyridinones, and aminofuranones.17 Additionally, 4a harbors the highly versatile N,N-disubstituted-1,3-aminodiene motif.
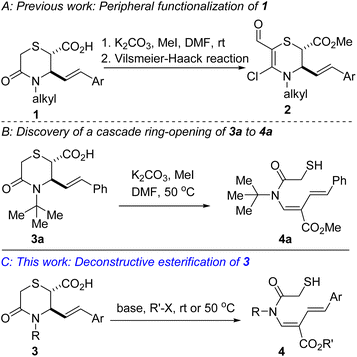 |
| Fig. 1 (A) Peripheral functionalization of N-alkylated allylic thiomorpholinone acids, (B) serendipitous discovery of a deconstructive esterification protocol, (C) proposed plan for the synthesis of cross-conjugated aminodienes of type 4. | |
Desiring an expedient and practical approach for accessing cross-conjugated aminodienes of type 4a, as well as to gain a full understanding of the mechanistic underpinnings of the transformation, we sought to build on the aforementioned lone example by examining the scope of deconstructive esterification of thiomorpholinone-tethered alkenoic acids (Fig. 1C). Intrinsic to our design was the prospect of exploiting this cascade reaction, given that such domino processes are inherently step and atom-economical. Cascade reactions often lead to a reduction in the amount of waste and in the number of purification steps. Efforts toward the elicitation of our ideals are described herein.
We commenced these studies by benchmarking our optimization efforts toward the synthesis of cross-conjugated aminodienes with the reaction conditions described in Table 1. In the event, using acid 3a, K2CO3 emerged as the most effective base and DMF emerged as the solvent of choice.
Table 1 Optimization of the deconstructive functionalization of allylic thiomorpholinone acid 3a
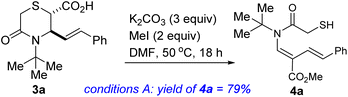
|
Entry |
Deviation from conditions A |
% Yield of 4a |
1 |
Tetrahydrofuran (THF) as solvent |
66 |
2 |
2-Methyltetrahydrofuran (2-MeTHF) as solvent |
70 |
3 |
Acetonitrile (MeCN) as solvent |
52 |
4 |
N,N-Dimethylacetamide (DMA) as solvent |
73 |
5 |
DMSO as solvent |
59 |
6 |
Na2CO3 in place of K2CO3 |
75 |
7 |
Cs2CO3 in place of K2CO3 |
70 |
8 |
CsF in place of K2CO3 |
59 |
9 |
NaHCO3 in place of K2CO3 |
38 |
10 |
KHCO3 in place of K2CO3 |
57 |
11 |
After 48 h at room temperature |
28 |
Under these mild conditions, the scope of this cascade esterification and ring-opening protocol with respect to the organic bromide was explored. In the event, the differentially substituted β-enaminoates depicted in Scheme 1 were obtained. Benzyl-, allyl-, alkyl-, and propargyl bromides are all competent electrophiles. The synthesis of ring-closing metathesis-suitable trienes such as 4d/e/i sets the stage for the late-stage construction of medium- and large-sized lactones.
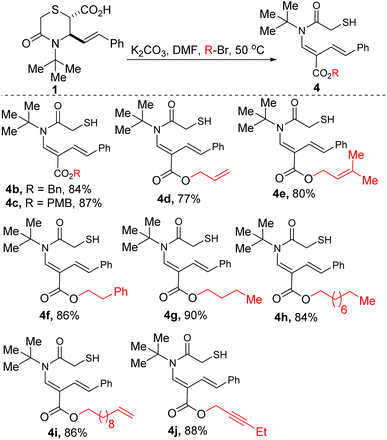 |
| Scheme 1 Deconstructive esterification of allylic thiomorpholinone acid 3a with various organic bromides. | |
We have explored the scope of this deconstructive esterification protocol with respect to the electronic properties of the alkenyl moiety. After noticing that the N-aryl substituted thiomorpholinone acids underwent deconstructive methylation faster than 3a, the former were used to evaluate the scope. In the event, we find that lactam acids harboring both electron-rich and electron-deficient styrenyl groups react competently (Scheme 2, 4k–r). At present, the synthesis of alkenoic acid 3 is limited to cinnamaldehyde-derived α,β-unsaturated imines, which explains why we have not evaluated other alkene groups. Knowing that the nature of the nitrogen substituent present on a nitrogen-containing compound can have a dramatic effect on its biological activity and reactivity, lactam acids bearing diverse aryl substitution on nitrogen have been surveyed (see 4s–y). The results show that electronically diverse N-aryl substituents are amenable to this deconstructive esterification protocol. Additionally, ortho-substituted aryl moieties can be installed (see 4t). The incorporation of a fluorinated moiety (see 4o) generally increases the solubility, lipophilicity, and metabolic stability of the parent molecules, thus, explaining why ∼25% of existing preclinical drugs and 40% of agrochemicals contain at least one fluorine atom.18 Specifically, the CF3 group enjoys a privileged role because its incorporation often enhances efficacy by promoting electrostatic interactions with targets, improving cellular membrane permeability, and increasing robustness toward oxidative metabolism of the drug.19 It is therefore noteworthy that diene 4u, which bears a CF3 group, is obtainable in good yield. Simultaneous variation of the N-aryl substituent and the styrenyl unit has also been achieved (see 4z–z3).21 Iodoarylated substituents are well tolerated in this transformation (see 4x, 4y, and 4z3), which bodes well for late-stage diversification given that the iodo group is an excellent requisite group for cross-coupling purposes.20
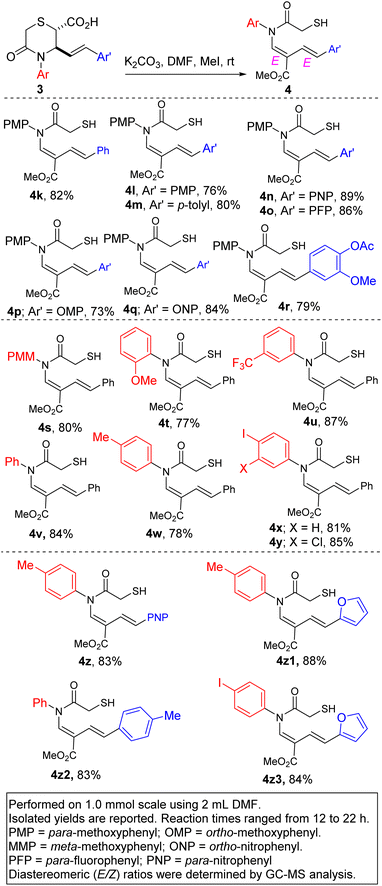 |
| Scheme 2 Scope of deconstructive methylesterification of allylic thiomorpholinone acids. | |
A plausible mechanism for the deconstructive esterification, which takes advantage of the relatively weak C–S bond, involves base-assisted O-methylation of 3b to arrive at 5, which undergoes base-assisted elimination to furnish 6 (Fig. 2). The cascade process continues with concomitant 1,2-styryl migration to afford the 1,3-diene (i.e., 4a), following aqueous workup.
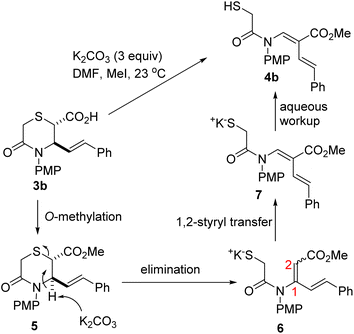 |
| Fig. 2 Proposed pathway for the deconstructive esterification (substrate 3b is chosen for simplicity). | |
Some mechanistically intuitive experiments have revealed that N-aryl-substituted thiomorpholinone acids bearing internally trisubstituted styrenes are not amenable to base-mediated ring-opening at room temperature (Scheme 3, see 8a/b). However, a thiomorpholinone-tethered externally trisubstituted alkenoic acid does undergo successful deconstructive methylation (see 4z4). We attribute the failure to observe ring-opening in 8a/b to steric encumbrance imposed by the methyl group, which is closer to the reactive center. In the case of 4z4, although the additional phenyl group is sterically more imposing than a methyl group, it is positioned further away from the reactive site. The sensitivity of the deconstructive esterification to steric congestion is further highlighted by the observation that no ring-opening occurs when the alkenyl group resident in 3b is replaced by an electronically more suitable aryl group (see 10a). An alkyl-substituted thiomorpholinone does not undergo ring-opening (see 10b), probably because in this case, the α-amino proton is not acidic enough for deprotonation by K2CO3.
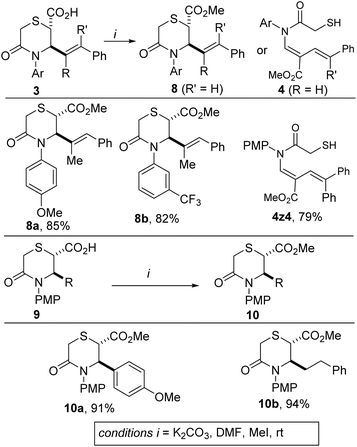 |
| Scheme 3 Control experiments on the methylesterification of diverse thiomorpholinone acids. | |
In summary, versatile cross-conjugated thiol-bearing aminodienes have been prepared in a stereocontrolled and modular manner, by engaging thiomorpholinone-tethered alkenoic acids in a cascade process featuring esterification, base-mediated ring opening and concomitant 1,2-styryl migration. Whereas N-arylated allylic thiomorpholinones readily undergo deconstructive esterification, the less activated N-alkylated congeners are less charitable substrates and require heating to 50 °C. The C6-alkenyl substituent is necessary for ring-opening to occur. However, the deconstructive esterification is quite sensitive to the stereoelectronic properties of the alkene. The interrogation of 4 in Diels–Alder reactions as well as the evaluation of their leishmanicidal activities are underway.
Author contributions
A. O. F. – investigation, data curation, validation; J. G. – investigation, methodology; C. B. – investigation, methodology; T. K. B. – conceptualization, project administration, investigation, supervision, writing – original draft, funding acquisition.
Conflicts of interest
There are no conflicts of interest to declare.
Acknowledgements
We are grateful to Central Washington University for financial support through startup funds to T. K. B. The School of Graduate Studies and Research is thanked for partial support of this work through a Faculty Research Award to T. K. B. and a graduate fellowship to A. O. F. Provost DenBeste is thanked for research fellowships to J. G. and C. B.
Notes and references
-
(a) W. C. White, Chem.-Biol. Interact., 2007, 166, 10–14 CrossRef CAS PubMed;
(b) G. Evano, N. Blanchard and M. Toumi, Chem. Rev., 2008, 108, 3054–3131 CrossRef CAS PubMed;
(c) M. S. McCammant, L. Liao and M. S. Sigman, J. Am. Chem. Soc., 2013, 135, 4167–4170 CrossRef CAS PubMed;
(d) Q.-A. Chen, D. K. Kim and V. M. Dong, J. Am. Chem. Soc., 2014, 136, 3772–3775 CrossRef CAS PubMed;
(e) Y. Zhu, R. G. Cornwall, H. Du, B. Zhao and Y. Shi, Acc. Chem. Res., 2014, 47, 3665–3678 CrossRef CAS PubMed;
(f) J. R. Chen, X.-Q. Hu, L.-Q. Lu and W.-J. Xiao, Chem. Rev., 2015, 115, 5301–5365 CrossRef CAS PubMed;
(g) J. Han, A. X. Jones and X. Lei, Synthesis, 2015, 47, 1519–1533 CrossRef CAS;
(h) S. Kotha, A. S. Chavan and D. Goyal, ACS Comb. Sci., 2015, 17, 253–302 CrossRef CAS PubMed;
(i) V. Saini, M. O'Dair and M. S. Sigman, J. Am. Chem. Soc., 2015, 137, 608–611 CrossRef CAS PubMed;
(j) Z.-L. Tao, A. Adili, H.-C. Shen, Z.-Y. Han and L.-Z. Gong, Angew. Chem., Int. Ed., 2016, 55, 4322–4326 CrossRef CAS PubMed;
(k) N. J. Adamson, E. Hull and S. J. Malcolmson, J. Am. Chem. Soc., 2017, 139, 7180–7183 CrossRef CAS PubMed;
(l) A. H. Christian, Z. L. Niemeyer, M. S. Sigman and F. D. Toste, ACS Catal., 2017, 7, 3973–3978 CrossRef CAS PubMed;
(m) N. K. Mishra, S. Sharma, J. Park, S. Han and I. S. Kim, ACS Catal., 2017, 7, 2821–2847 CrossRef CAS;
(n) X.-H. Yang and V. M. Dong, J. Am. Chem. Soc., 2017, 139, 1774–1777 CrossRef CAS PubMed.
- J. Barluenga, A. Suarez-Sobrino and L. A. Lopez, Aldrichimica Acta, 1999, 32, 4 CAS.
-
(a) L. E. Overman and L. A. Clizbe, J. Am. Chem. Soc., 1976, 98, 2352 CrossRef CAS;
(b) L. E. Overman, G. F. Taylor, K. N. Houk and L. S. Domelsmith, J. Am. Chem. Soc., 1978, 100, 3182 CrossRef CAS;
(c) W. Oppolzer, L. Bieber and E. Francotte, Tetrahedron Lett., 1979, 20, 4537 CrossRef;
(d) L. E. Overman, R. L. Freerks, C. B. Petty, L. A. Clizbe, R. K. Ono, G. F. Taylor and P. J. Jessup, J. Am. Chem. Soc., 1981, 103, 2816 CrossRef CAS;
(e) A. D. Brosius, L. E. Overman and L. Schwink, J. Am. Chem. Soc., 1999, 121, 700 CrossRef CAS.
- Y. N. Timsina, S. Biswas and T. V. RajanBabu, J. Am. Chem. Soc., 2014, 136, 6215–6218 CrossRef CAS PubMed.
-
(a) T.-J. Hu, M.-Y. Li, Q. Zhao, C.-G. Feng and G.-Q. Lin, Angew. Chem., Int. Ed., 2018, 57, 5871–5875 CrossRef CAS PubMed;
(b) N. Miyaura and A. Suzuki, Chem. Rev., 1995, 95, 2457–2483 CrossRef CAS;
(c) A. Suzuki, J. Organomet. Chem., 1999, 576, 147–168 CrossRef CAS;
(d) M. W. Van Laren and C. J. Elsevier, Angew. Chem., Int. Ed., 1999, 38, 3715–3717 CrossRef CAS;
(e) B. M. Trost and T. Schmidt, J. Am. Chem. Soc., 1988, 110, 2301–2303 CrossRef CAS;
(f) C. Guo and X. Lu, Tetrahedron Lett., 1992, 33, 3659–3662 CrossRef CAS;
(g) C. Guo and X. Lu, Synlett, 1992, 405–406, DOI:10.1055/s1992-21360;
(h) B. M. Trost and U. Kazmaier, J. Am. Chem. Soc., 1992, 114, 7933–7935 CrossRef CAS;
(i) Y. Wang and F. G. West, Synthesis, 2002, 99–103, DOI:10.1055/s-2002-19297.
-
(a) C. M. M. Santos and A. M. S. Silva, Prog. Heterocycl. Chem., 2013, 25, 409–453 CAS;
(b) C.-V. T. Vo, G. Mikutis and J. W. Bode, Angew. Chem., Int. Ed., 2013, 52, 1705–1708 CrossRef CAS PubMed;
(c) L. Wang, W. He and Z. Yu, Chem. Soc. Rev., 2013, 42, 599–621 RSC;
(d) Y.-Q. Wang, C.-B. Yu, D.-W. Wang, X.-B. Wang and Y.-G. Zhou, Org. Lett., 2008, 10, 2071–2074 CrossRef CAS PubMed;
(e) H. Zhang, X. Ye and M. Cai, J. Chem. Res., 2008, 305–307 CrossRef CAS;
(f) M. Ichimaru, A. Kato and Y. Hashimoto, J. Nat. Prod., 2000, 63, 1675–1676 CrossRef CAS PubMed;
(g) D. Meng, W. Chen and W. Zhao, J. Nat. Prod., 2007, 70, 824–829 CrossRef CAS PubMed;
(h) Y. Fukata, K. Asano and S. Matsubara, J. Am. Chem. Soc., 2015, 137, 5320–5323 CrossRef CAS PubMed;
(i) A. St-Gelais, J. Legault, V. Mshvildadze and A. Pichette, J. Nat. Prod., 2015, 78, 1904–1909 CrossRef CAS PubMed;
(j) W. Li, C. Schlepphorst, C. Daniliuc and F. Glorius, Angew. Chem., Int. Ed., 2016, 55, 3300–3303 CrossRef CAS PubMed.
- K. Shukla, D. V. Ferraris, A. G. Thomas, M. Stathis, B. Duvall, G. Delahanty, J. Alt, R. Rais, C. Rojas, P. Gao, Y. Xiang, C. V. Dang, B. S. Slusher and T. Tsukamoto, J. Med. Chem., 2012, 55, 10551–10563 CrossRef CAS PubMed.
- F. Pierre, C. F. Regan, M.-C. Chevrel, A. Siddiqui-Jain, D. Macalino, N. Streiner, D. Drygin, M. Haddach, S. E. O'Brien, W. G. Rice and D. M. Ryckman, Bioorg. Med. Chem. Lett., 2012, 22, 3327–3331 CrossRef CAS PubMed.
- B. Han, J. L. Liu, Y. Huan, P. Li, Q. Wu, Z. Y. Lin, Z. F. Shen, D. L. Yin and H. H. Huang, Chin. Chem. Lett., 2012, 23, 297 CrossRef CAS.
- P. Theodosis-Nobelos, M. Kourti, A. Gavalas and E. A. Rekka, Bioorg. Med. Chem. Lett., 2016, 26, 910 CrossRef CAS PubMed.
- M. Biava, G. C. Porretta, D. Deidda, R. Pompei, A. Tafi and F. Manetti, Bioorg. Med. Chem., 2003, 11, 515 CrossRef CAS PubMed.
- K.-K. Tooulia, P. Theodosis-Nobelos and E. A. Rekka, Arch. Pharm., 2015, 348, 629 CrossRef CAS PubMed.
-
(a) M. H. Keylor, J. E. Park, C.-J. Wallentin and C. R. J. Stephenson, Tetrahedron, 2014, 70, 4264–4269 CrossRef CAS;
(b) S. Marcaccini, R. Pepino, T. Torroba, D. Miguel and M. A. García-Valverde, Tetrahedron Lett., 2002, 43, 8591–8593 CrossRef CAS;
(c) A. J. Williams, S. Chakthong, D. Gray, R. M. Lawrence and T. Gallagher, Org. Lett., 2003, 5, 811–814 CrossRef CAS PubMed;
(d) S. Indumathi, S. Perumal and J. C. Menéndez, Tetrahedron, 2011, 67, 7101–7105 CrossRef CAS;
(e) Z. Liu and A. Nefzi, Tetrahedron Lett., 2012, 53, 1013–1014 CrossRef CAS;
(f) A. Otto and J. Liebscher, Synthesis, 2003, 8, 1209–1214 Search PubMed.
-
(a) H. M. Davies and R. E. Beckwith, Chem. Rev., 2003, 103, 2861–2904 CrossRef CAS PubMed;
(b) R. Giri, B. F. Shi, K. M. Engle, N. Maugel and J. Q. Yu, Chem. Soc. Rev., 2009, 38, 3242–3272 RSC;
(c) R. H. Crabtree and A. Lei, Chem. Rev., 2017, 117, 8481–8482 CrossRef CAS PubMed;
(d) N. Y. S. Lam, K. Wu and J. Q. Yu, Angew. Chem., 2021, 133, 15901–15924 CrossRef;
(e) T. Cernak, K. D. Dykstra, S. Tyagarajan, P. Vachal and S. W. Krska, Chem. Soc. Rev., 2016, 45, 546–576 RSC.
-
(a) J. R. Donald and W. P. Unsworth, Chem.–Eur. J., 2017, 23, 8780–8799 CrossRef CAS PubMed;
(b) J. B. Roque, Y. Kuroda, L. T. Gottemann and R. Sarpong, Nature, 2018, 564, 244–248 CrossRef CAS PubMed;
(c) Y. Hu, D. Stumpfe and J. Bajorath, J. Med. Chem., 2017, 60, 1238–1246 CrossRef CAS PubMed;
(d) A. M. Szpilman and E. M. Carreira, Angew. Chem., Int. Ed., 2010, 49, 9592–9628 CrossRef CAS PubMed;
(e) Z. C. Cao and Z. J. Shi, J. Am. Chem. Soc., 2017, 139, 6546–6549 CrossRef CAS PubMed.
- T. K. Beng, A. O. Farah and V. Shearer, RSC Adv., 2020, 10, 37153 RSC.
-
(a) J. Maruyama, H. Yamashita, T. Watanabe, S. Arai and A. Nishida, Tetrahedron, 2009, 65, 1327–1335 CrossRef CAS;
(b) N. Toyooka, M. Okumura and H. Nemoto, J. Org. Chem., 2002, 67, 6078–6081 CrossRef CAS PubMed.
- T. Liang, C. N. Neumann and T. Ritter, Angew. Chem., Int. Ed., 2013, 52, 8214–8264 CrossRef CAS PubMed.
- K. Muller, C. Faeh and F. Diederich, Science, 2007, 317, 1881–1886 CrossRef PubMed.
- T. K. Beng and A. Moreno, New J. Chem., 2020, 44, 4257–4261 RSC.
- The synthesis of alkenoic acid 3 using the 1,3-azadiene-anhydride reaction is currently limited to N-alkyl- and N-aryl-α,β-unsaturated imines. Strongly electron-withdrawing N-substituents such as N-Boc- and N-Cbz-α,β-unsaturated imines are not tolerated. See H. Braunstein, S. Langevin, M. Khim, J. Adamson, K. Hovenkotter, L. Kotlarz, B. Mansker and T. K. Beng, Org. Biomol. Chem., 2016, 14, 8864–8872 RSC.
|
This journal is © The Royal Society of Chemistry 2023 |