DOI:
10.1039/D2RA07108H
(Paper)
RSC Adv., 2023,
13, 4173-4181
A facile synthesis of highly efficient In2S3 photocatalysts for the removal of cationic dyes with size-dependent photocatalysis†
Received
9th November 2022
, Accepted 16th January 2023
First published on 31st January 2023
Abstract
In this study, a 3D thornball-like hierarchical β-In2S3, displaying extremely rapid photodegradation of cationic dyes, was synthesized by a facile method. The formation of a uniform thornball-like structure depended on the microwave reaction method and citric acid as the pH regulator. The size of In2S3 was easily adjusted by changing the microwave irradiation time from 5 min to 15 min. The morphology, structure, composition, energy level, charge separation, and surface properties of different-sized In2S3 were characterized. The results showed that In2S3 synthesized in 10 min (In2S3-10) displayed optimal interface property for the electron–hole separation, maximum hydrophilia with most surface negative charges for the surface adsorption, contributing to the complete photodegradation of rhodamine B (RhB) in just 25 minutes of visible light illumination. The photodegradation path of RhB was speculated with four possible paths, including the processes of de-ethylation, open-ring of xanthene, and rupture of carbon–carbon bonds up to the decomposition into small molecules. Finally, the reusability of In2S3-10 was tested, obtaining nearly 96% photodegradation efficiency after sequential 5 cycles.
1 Introduction
Recently, air and water pollution are major environmental problems threatening human health. The most ideal and feasible strategy to solve the problem is semiconductor photocatalytic technology. Common semiconductor photocatalysts such as TiO2, CdS, ZnS have been studied previously.1 However, these photocatalysts either do not have the high efficiency as TiO2 or2 do not have high stability as CdS and ZnS.3,4 Therefore, it is important to synthesize practical photocatalysts for application in photocatalytic technology. Normally, the synthesis strategy involves structural and compositional optimization because the photocatalysis is strongly dependent on crystal morphologies and structural features at the nanometer level.5
Indium sulphide is a nontoxic III–VI group semiconductor, as the most stable crystal phase, β-In2S3 displays the narrow bandgap of 1.9–2.3 eV.6 Many inherent vacancies are conducive to forming the transition defect zones in β-In2S3, contributing to the excellent light-harvesting ability in visible light range.7 Ordinary nano-photocatalysts with large specific surface areas have numerous surface active sites; however, the easy agglomeration results in considerable surface active sites being ineffective.8 Micro/nano hierarchical photocatalysts can retain the advantages of nano-photocatalysts by avoiding agglomeration effectively, for example, their large interface area not only affords abundant active sites for better contact of pollutant with catalyst but also contribute to the high separation of electron–hole pairs.5 Previous reports have confirmed that the application of 3D ZnO in the photodegradation of methyl orange displayed higher performance than ZnO fragments.9 Therefore, it is suggested that micro/nano hierarchical In2S3 has special advantages in the photocatalysis. Previously, micro/nano hierarchical In2S3 has been prepared by a solvothermal method10–15 or a hot injection method.16,17 However, the solvothermal reaction requires high temperature as 150–195 °C and a long reaction time of 2–30 h, and the hot injection method demands complicated reagents or surfactant (glycerinum, myristic acid, and octadecene) to react under an inert gas atmosphere at high temperature.
Based on the direct coupling of microwave energy with a reactant, the microwave irradiation method presents a very fast reaction rate in material synthesis.18 The extremely fierce reaction driven by microwave energy contributed to the advantageous three-dimensional growth of the product.19 Thus, products tend to aggregate into hierarchical clusters with more uniform size distribution and more complete crystal morphology. By changing the reaction parameters, such as temperature, time, or microwave power, the size and morphology can be adjusted easily to obtain the controllable preparation of the photocatalysts with excellent surface properties for high photocatalysis. Previously, preparation of In2S3 nanoflowers by PVP-assisted microwave irradiation method,19 dandelion flower-like In2S3 by the microwave solvothermal method20 and dendrites-type In2S3 by the microwave irradiation method under nitrogen atmosphere21 have been reported. In these reactions, long-chained surfactants, high reaction temperature, or complicated process are needed, resulting in the following disadvantages inevitably. (1) The surfactant covers the surface of In2S3 to generate the charge transfer obstacle between the photocatalysts and organic pollutes; (2) high reaction temperature and the complicated process lead to increased environmental contamination and synthesis cost. Therefore, how to obtain desired 3D micro/nano hierarchical In2S3 by a facile method requires further exploration.
In this work, we put forward a facile microwave strategy to synthesize surfactant-free thornball-like hierarchical In2S3. All reagents are nontoxic with deionized water as the solvent, and the reaction is achievable as fast as 5 min at the low temperature of 90 °C. Furthermore, different-sized In2S3 were synthesized easily by adjusting the microwave reaction time, which was applied in the photodegradation of rhodamine B (RhB) to obtain size-dependent photocatalysis. Mid-sized In2S3 (In2S3-10) displayed excellent photocatalysis, which was much better than that previously reported In2S3 using a similar structure,22–26 confirming its potential application in the treatment of environmental pollutants. The corresponding explanation was given based on the synergistic effect from the surface adsorption of dye molecules followed by the oxygenolysis of active specifies.
2 Experimental
2.1 Chemicals
Indium chloride tetrahydrate (InCl3·4H2O, AR), thioacetamide (TAA, AR), citric acid (CA, AR), oxalic acid (AR), ethanol (AR), rhodamine B (RhB, AR), crystal violet (CV, AR), malachite green (MG, BS), methyl orange (MO, Ind), congo red (CR, Ind), acid red (AR, Ind), isopropanol (IPA, AR), triethanolamine (TEOA, AR) and benzoquinone (BQ, AR) were purchased from the Sinopharm Chemical Reagent Co, Ltd.
2.2 Synthesis of In2S3 micro/nanoparticles
60 mL mixed solution containing InCl3·4H2O (0.025 mol L−1) and TAA (0.1 mol L−1) was adjusted to pH = 2.0 by CA. Then, the mixed solution was transferred to an XH-MC-1 microwave synthesis reactor to react at 90 °C, 200 W for 5, 10, and 15 min. After the reaction was completed, the products were centrifuged at 10
000 rpm for 10 min followed by washing and drying to obtain yellow powders, named In2S3-5, In2S3-10, and In2S3-15. As a comparison, the synthesis conditions of In2S3-10 were changed as follows: (1) chemical bath deposition (CBD) was used instead of the microwave method; (2) no pH regulator was used; (3) oxalic acid instead of CA was used as a pH regulator.
2.3 Photocatalysis experiments
In2S3-5, In2S3-10, and In2S3-15 were used to carry out the photocatalysis experiments (S1 in the ESI†). To detect the active species in the photodegradation of RhB with In2S3-10, IPA (1 mM), TEOA (1 mM), and BQ (1 mM) were used as the scavengers for ˙OH, h+, and ˙O2−, respectively, by adding them in the system. The reusability of In2S3-10 was tested using the equivalent photocatalyst in every experiment.
2.4 Characterization
The morphology of products was studied by scanning electron microscopy (FEI Sirion 200) and transmission electron microscopy (JEOL-2010). The crystal phase was measured using an X-ray diffractometer (MXP18AHF) with monochromated CuKα radiation (λ = 1.54056 Å). The optical measurements were performed by UV-vis diffused reflectance spectroscopy (UV 2550 spectrophotometer) and fluorescence spectra (an F-4500 spectrofluorometer) with an excitation wavelength of 218 nm. The photoelectrochemical measurements including cyclic voltammetry (C–V), linear sweep voltammetry (LSV), transient photocurrent-time (I–t) curves, and electrochemical impedance spectroscopy (EIS) were studied using a CHI-660C electrochemical workstation (S2 in the ESI†). The specific surface areas were measured on the NOVA 2000e instrument with Brunauer–Emmett–Teller (BET) method, and the pore-size distribution was estimated using the Barrett–Joyner–Halenda (BJH) method. The measurement of zeta potential was performed on Brookhaven 90 plus zeta potential analyser. The water contact angles were measured with a Kino SL250 contact angle meter (USA Kino Industry Co. Ltd). FT-IR spectra were recorded on a Nicolet Magna-IRTM 750 spectrometer. The photodegradation products were identified by liquid chromatography-mass spectrometry (Shimadzu LCMS-8030) with a mass scanning range of 50–450 m/z, similar to that reported in our previous paper.27,28
3 Results and discussion
3.1 Influence of synthetic condition on the morphology of In2S3
Fig. 1 shows the influence of the reaction method and the category of pH regulator. Fig. 1a and b show SEM images of In2S3 synthesized using CBD and the microwave method, respectively. With the CBD method, two morphologies of In2S3 including thornball-like structure with the size of 1–1.5 μm and irregular aggregated particles (as shown in a white dotted line) generated, however, the microwave method resulted in the formation of 500–600 nm sized uniform thornball-like structures. Furthermore, we changed the pH regulator, compared to that with CA as a pH regulator (Fig. 1b), extremely small-sized spherical nanoparticles were formed without the pH regulator (Fig. 1c), however, the oxalic acid regulator led to the formation of a flower-like structure with the size of 1 μm. Obviously, the microwave method contributed to the generation of monomorphic morphology, CA promoted the production of thornball-like structures. The formation of thornball-like In2S3 was possibly based on the followings. Firstly, In3+ coordinated with CA to form the In-CA compound. With the hydrolysis of TAA to release H2S, the In-CA compound was attacked by H2S to produce the In2S3 crystal nuclei followed by the oriented growth into nanoflakes. After more and more nanoflakes were formed, they tended to aggregate into hierarchical clusters as the microwave reaction promoted to three-dimensional growth of the products.19
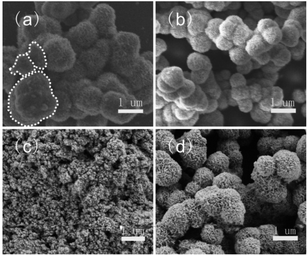 |
| Fig. 1 SEM images of In2S3 synthesized using different reaction methods as CBD (a) and microwave method (b–d), and different pH regulators, such s as CA (a and b), none (c), and oxalic acid (d). | |
3.2 Characterization
3.2.1 Morphologies, structures, and composition. Fig. 2 shows the SEM morphology and corresponding EDS spectrum (with the gold-spraying treatment) of In2S3 synthesized for different microwave reaction time. In2S3-5 displayed the thornball-like structure with a size of 400–500 nm, which was assembled by thin nanoflakes (Fig. 2a). The EDS spectrum confirmed the presence of In, S, C, O, and Au elements in the product with an In/S ratio of 0.692 (Fig. 2b). The high content of C originated from the presence of CA on its surface. In2S3-10 displayed almost the same morphology except the size increased to 500–600 nm (Fig. 2c). The EDS spectrum showed an increase in the In/S ratio of 0.717 and decreased content of carbon (Fig. 2d). Furthermore, the size obviously increased to 700–800 nm in In2S3-15 (Fig. 2e). The In/S ratio further increased to 0.728 and the content of carbon decreased further (Fig. 2f). Clearly, microwave reaction promoted the formation of micro/nano thornball-like In2S3, rapidly, the increase of the reaction time hardly resulted in the change in morphology, just promoting the increase in the size. Moreover, the increased time resulted in the increased ratio of In/S and decreased the content of carbon, which was originating from the gradual decomposition of In-CA composites for the transformation into In2S3.
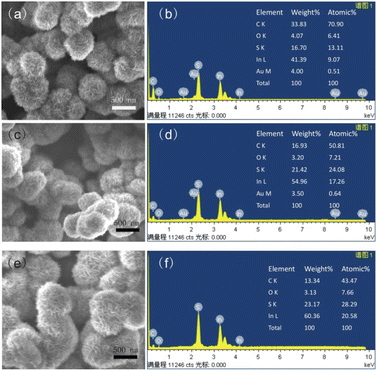 |
| Fig. 2 SEM (a) and EDS (b) of In2S3-5, SEM (c) and EDS (d) of In2S3-10, SEM (e) and EDS (f) of In2S3-15. | |
The phase structure of the products was characterized from powder XRD (Fig. 3a). The diffraction peaks at 2θ = 27.4°, 33.2°, 43.6° and 47.7° matched with (109), (0012), (1015) and (2212) crystal planes of tetragonal β-In2S3 (a = b = 7.619 A, c = 32.329 A, JCPDS PCPDFWIN #25-0390), respectively.7,19 With the reaction time prolonging, the full-width half maximum (FWHM) of (2212) peak decreased (FWHM = 2.5°, 2.2° and 1.5° for In2S3-5, In2S3-10, and In2S3-15, respectively), implying that the average size of In2S3 increased. Additionally, the appearance of the sharper peaks in large-sized In2S3 indicated better crystallinity of the products.
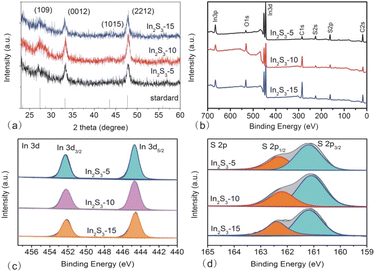 |
| Fig. 3 XRD (a), XPS survey (b), In spectrum (c), and S spectrum (d) of In2S3-5, In2S3-10, and In2S3-15. | |
To characterize the product composition more accurately, XPS spectra were obtained. The survey spectrum displayed the existence of In, S, C, and O elements in three In2S3 samples (Fig. 3b). The oxygen peak originated from the adsorption of O2 or H2O molecules in the atmosphere onto the samples, while the carbon peak was due to the residual CA molecules and CO2 from the atmosphere.29 To obtain information on the valence states and binding energy, In and S spectra were collected. There were two peaks located at 445 eV and 452 eV in In 3d spectrum (shown in Fig. 3c), which corresponded to In 3d5/2 and In 3d3/2, respectively. The spin–orbit separation of In was 7 eV, indicating that In was present in the form of In(III). In the S 2p spectrum, two peaks at 161 eV and 162 eV related to S 2p3/2 and S 2p1/2 could be seen (shown in Fig. 3d). The spin–orbit separation of 1 eV suggested that S existed as S2− in the In2S3.22
Fig. 4 shows TEM images of three In2S3. In2S3-5 displayed a 400–500 nm spherical structure assembled by 10–20 nm nanoflakes. The nanoflakes interleaved to form thornball-like structures with irregular edges (Fig. 4a). In2S3-10 presented similar morphology to In2S3-5 except for the increased size to 600–700 nm (Fig. 4b), and the size of In2S3-15 further increased to 700–800 nm (Fig. 4c). Obviously, as the reaction time increased, the size of the as-synthesized In2S3 increased largely. HRTEM images showed the interplanar spacing of 0.324 (or 0.323) nm and 0.194 nm corresponding to (109) and (2212) planes of β-In2S3. The polycrystalline rings, as indicated in SAED, resulted from (109), (2212), and (419) planes of tetragonal β-In2S3.
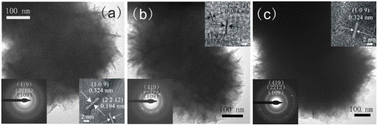 |
| Fig. 4 TEM of In2S3-5 (a), In2S3-10 (b), and In2S3-15 (c), the insets are HRTEM and SAED of three samples, respectively. | |
3.2.2 Energy level. To obtain the information on the energy level for In2S3, we firstly investigated UV-vis DRS of different-sized In2S3 (Fig. 5a), they absorbed visible light within 200–650 nm with the absorption band edge around 610–630 nm. On the basis of DRS results, the optical bandgap (Eg−O) of In2S3 was calculated using the Tauc plot method30 (inset to Fig. 5a), which was 2.12–2.20 eV for three In2S3 (as shown in Table 1). Obviously, with the size of In2S3 increasing, the bandgap decreased with the absorption band edge red-shifted. Small-sized In2S3 had a large bandgap, which was unhelpful for light-harvesting, however, the large specific surface area contributed to strong light reflection and scattering ability. Comprehensively, In2S3-10 displayed complementary light absorption, reflection, and scattering ability, obtaining the maximum light-harvesting ability. Next, we calculated EVB (valence band edge potential) and ECB (conduction band edge potential) according to the empirical equation23 (S3 in the ESI†). Obviously, with the size of In2S3 increasing, EVB decreased and ECB increased gradually (as shown in Table 1), originating from the quantum size effect.31
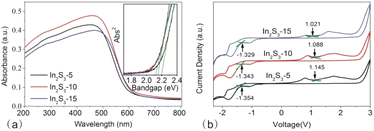 |
| Fig. 5 UV-vis DRS (a) and C–V curve (b) of In2S3-5, In2S3-10, and In2S3-15, the inset to (a) is the spectrum, which shows the determination of bandgap by the direct bandgap method. | |
Table 1 The corresponding data of energy level for different In2S3 based on optical and electrochemical methodsa
sample |
In2S3-5 |
In2S3-10 |
In2S3-15 |
Note: Eox and Ered represent the onset potential of the oxidation peak and reduction peak in C–V curves. |
Eg−O (V) |
2.20 |
2.15 |
2.12 |
EVB−cal (eV) |
1.30 |
1.28 |
1.26 |
ECB−cal (eV) |
−0.90 |
−0.88 |
−0.86 |
Eox (V) |
0.651 |
0.647 |
0.602 |
EVB−CV (eV) |
1.304 |
1.300 |
1.255 |
Ered (V) |
−1.402 |
−1.364 |
−1.345 |
ECB−CV (eV) |
−0.749 |
−0.711 |
−0.692 |
Eg−E (V) |
2.053 |
2.011 |
1.947 |
C–V measurement is an effective method to obtain the energy level of nanoparticles.28,29 Based on the quantitative correlation between the band edge potential with the onset potential of the oxidation and reduction peaks (S3 in the ESI†), we obtained the ionization potential (IP) and the electron affinity (EA). From Fig. 5b, Eox negatively shifted gradually from In2S3-5 to In2S3-15, resulting in decreased IP, however, Eox positively shifted gradually for increased EA. With the size of In2S3 increasing, the electrochemical bandgap (Eg−E = IP − EA) decreased gradually.
3.2.3 Charge separation. PL spectra that resulted from the recombination of the photoexcited charge carriers also reflect the charge separation ability. Fig. 6a shows the spectra of different-sized In2S3 with the emission peaks appearing at 570–580 nm, near the peak at 587 nm reported in the literature.32 Different from In2S3-10 and In2S3-15, an obvious blue-shifted peak in In2S3-5 attributed to the quantum size effect was observed. Among the three samples, In2S3-10 presented the lowest emission peak intensity, indicating that the electrons and holes were not easy to recombine.33 It is well-known that the electron–hole recombination rate is connected closely with the surface states. Small-sized nanoparticles with a large special surface area are helpful to afford the interface for electron–hole separation, however, the presence of more surface atoms producing surface defects inevitably acted as the recombination centre.34 In2S3-10 balanced the effect originating from special surface area and surface defects, therefore, obtaining the highest electron–hole separation efficiency.
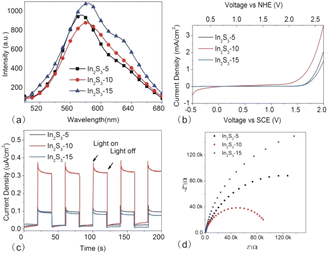 |
| Fig. 6 PL spectra (a), LSV (b), I–t (c) and EIS (d) curves of In2S3-5, In2S3-10 and In2S3-15. | |
The photoelectrochemical measurements are useful to probe electron–hole separation. Fig. 6b showed LSV curves of In2S3 particles. The increase in the current density of In2S3-10 was significantly higher than that of In2S3-5 and In2S3-15, indicating that In2S3-10 had a faster transfer rate of the photogenerated electrons. Moreover, we compared the onset potential of three samples, which represented the position of the Fermi level.33 In2S3-10 with the low onset potential suggested the low potential for the photoanode to integrate with the photocathode, contributing to the efficient separation of the photogenerated carriers.
Fig. 6c shows the transient I–t curves of different-sized In2S3. Generally, strong photocurrent response under illumination implies high separation efficiency of the charge carrier. Among the three samples, In2S3-10 exhibited the highest photocurrent density, which was about three times that of the other two samples, indicating that the material was highly efficient in separating the photogenerated electron–hole pairs. It was noted that the photocurrent of three samples came back rapidly to zero once the light was switched off. Good reproducibility of the photocurrent suggested the rapid photoresponse and recovery properties of the as-synthesized photocatalysts.
EIS was performed to evaluate the charge transfer resistance of In2S3-5, In2S3-10, and In2S3-15 (as shown in Fig. 6d). The diameter of the semicircle (or arc) in the Nyquist plots at high frequency provided the information on the charge transfer, typically, small diameter represented the low charge transfer resistance (Rct).35 Obviously, In2S3-10 displayed the lowest Rct in three samples, indicating the fastest charge transfer rate from the photocatalyst to the photoelectrode,36 which was beneficial to the electron–hole separation.
3.2.4 Surface property. The specific surface areas of the catalysts are important to determine their photocatalysis. Large specific surface areas not only afforded more interface active sites between photocatalysts and the dye molecules for effective adsorption but also enhanced charge separation efficiency to produce more oxidant radicals.11 Fig. 7 is the N2 adsorption–desorption isotherm of different-sized In2S3. The adsorption curves displayed hysteresis loops in the range of 0.45–0.99 P/P0 and presented type IV isotherms, which were identified as characteristic of mesoporous materials.37 The mesoporous structures originated from the space among the interleaved nanoflakes, indirectly evidencing that the as-synthesized In2S3 were hierarchically constructed.37 The pore size distribution was within 3–120 nm (using BJH analysis), typically presenting a bimodal shape with two maxima at ∼3.8 and ∼31 nm (insets to Fig. 7a–c). The pore size distribution at ∼3.8 nm is possibly attributed to the formed funnel-like pores by the interleaved nanoflakes in the centre of thornball-like structures,38 while that at ∼31 nm originated from the intervals among the nanoflakes. In2S3-10 had a slightly smaller pore size (31.45, 30.54, and 31.40 nm for In2S3-5, In2S3-10 and In2S3-15, respectively), indicating that nanoflakes were stacked tightly with small intervals, in accordance with a slightly lower adsorption amount in the high pressure region (Fig. 7b). Quantitative calculation showed that the BET surface area decreased with In2S3 size increasing (34.26, 26.17 and 20.73 m2 g−1 for In2S3-5, In2S3-10 and In2S3-15, respectively).
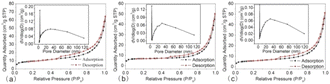 |
| Fig. 7 Nitrogen adsorption–desorption curves of In2S3-5 (a), In2S3-10 (b), and In2S3-15 (c) (the insets show the pore size distribution curves). | |
Besides the specific surface areas, the groups adsorbed on the surface of catalysts which impact the hydrophilicity and surface charge also influence the catalysis. FT-IR spectra were recorded to probe the surface groups of the three samples (as shown in Fig. 8a). The peak at 806 cm−1 corresponding to the In–S vibration peak39,40, and that at 476 cm−1 corresponding to the In–O vibration peak41,42 were observed in all three samples. The presence of In–O vibration originated from the interaction of In3+ with the oxygen-containing surface functional groups in CA molecules.43 Compared to In2S3-5 and In2S3-15, In2S3-10 displayed stronger peaks of ν(O–H) at 3425 cm−1, 1627 cm−1 and ν(In–O–C) at 1078 cm−1,24 respectively, indicating that –OH groups were the main adsorbed surface groups. The presence of –OH groups from CA molecules confirmed that the generation of In2S3 was originated from the decompostion of In-CA compound.
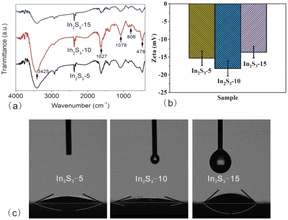 |
| Fig. 8 FT-IR spectra (a), zeta potential (b), and water contact angle measurements (c) of In2S3-5, In2S3-10, and In2S3-15. | |
Next, zeta potential, which is deemed as an indicator for the surface charge of the solid particles was measured. Zeta potential values of In2S3-5, In2S3-10 and In2S3-15 were −15.32, −18.25 and −13.63 mV, respectively (as shown in Fig. 8b). The negative charge taken on the surface was related to the adsorption of –OH groups on the surface. In2S3-10 had the most negative charges on its surface, indicating that lots of –OH groups were adsorbed. Finally, surface hydrophilicity of different-sized In2S3 samples were quantified from water contact angle measurements. Fig. 8c shows the contact angles of In2S3-5, In2S3-10 and In2S3-15 to be 24.85°, 14.38° and 42.39°, respectively. Obviously, three In2S3 presented hydrophilicity, in particular, In2S3-10 presented the maximum hydrophilicity, which was connected with more adsorbed –OH groups based on the complementary effects of the specific surface area and crystallinity.
3.3 Photocatalysis
3.3.1 The photodegradation of RhB with different-sized In2S3. As a semiconductor with a narrow band gap, In2S3 can be used as the photocatalyst. RhB was selected as the dye to measure the photocatalysis of In2S3. Fig. 9a shows the concentration decrease of RhB (characterized by Ct/C0) as a function of the reaction time. C0 and Ct corresponded to the maximum absorbance of RhB at the original and particular moment, respectively. Before the photodegradation, the suspension was kept in the dark for 30 minutes. During the initial 10 minutes, the absorbance decrease was relatively high, then it was almost not changing. We used (Ct − C0)/C0 during this period to represent the adsorption efficiency (ADE). Obviously, In2S3-10 displayed the highest ADE, which was possibly connected with the strongest hydrophilicity and most negative charges taken. With the light on, the concentration of RhB went on decreasing. Using a similar formula to obtain the photodegradation efficiency (PDE), In2S3-10 displayed a maximum PDE of up to 99.99%, just after 25 min of illumination, much higher than that previously reported with the similarly structured In2S3 (as shown in Table 2). As a comparison, the concentration change of RhB without In2S3 only displayed 2.34% absorbance attenuation under illumination. Obviously, In2S3 as the catalyst can photodegrade RhB efficiently.
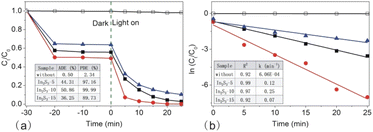 |
| Fig. 9 Photodegradation of RhB without In2S3 (□), with In2S3-5 (■), In2S3-10 (●), In2S3-15 (▲) (a) and the corresponding plots of ln (Ct/C0) versus irradiation time (b), the insert tables to (a) and (b) show adsorption efficiency (ADE), photodegradation efficiency (PDE) and correlation coefficient (R2), rate constant (k) of different samples. | |
Table 2 The reported efficiency and tested conditions of In2S3 from previous literature
Catalyst |
Light source |
Time (min) |
Degradation rate (%) |
Reference |
In2S3-10 |
300 W Xe lamp |
25 |
99.9 |
This work |
In2S3 |
500 W Xe lamp |
60 |
97.5 |
25 |
Ca2+ doped In2S3 |
300 W Xe lamp |
100 |
99.9 |
23 |
YTIS-4 |
300 W Xe lamp |
100 |
98.4 |
22 |
In2S3/5G |
300 W Xe lamp |
180 |
99.23 |
24 |
Ag: In2S3 (2) |
150 W Xe lamp |
180 |
99.5 |
26 |
Next, we measured the photodegradation of RhB during the photoreaction (Fig. 9b). It was approximately fitted with the liner equation of ln (Ct/C0) = −kt and the obtained R2 value was over 0.9, suggesting that the photodegradation reaction meets the pseudo-first-order kinetics as a function of the time. From the equation, the k value was obtained easily. With the size of In2S3 increasing, the k value increased first and then decreased largely. In2S3-10 showed the maximum k value up to 0.25 min−1, probably, the fastest rate in the photodegradation of RhB.
3.3.2 Photodegradation path. Mass spectra (MS) were collected to determine the photodegradation products of RhB. Fig. 10a shows the influence of the illumination time on the product of RhB in the case of In2S3-5. It showed the peak of the RhB matrix at m/z = 443 at the initial moment. With the illumination time increasing to 5 min, more peaks appeared with increased abundance in the range of m/z = 250–400. Furthermore, when the illumination time increased to 20 min, not only did the peaks in the range of m/z = 250–400 increase but also the peaks in the range of m/z = 50–150 increased with higher abundance. Obviously, with photoreaction time increasing, more and more small molecules with higher abundance appeared, indicating RhB was decomposed but not adsorbed by In2S3. Fig. 10b displayed the photodegradation product of RhB using different In2S3 as photocatalysts after 20 min of the illumination time. The abundance of small molecules instead of RhB matrix became relatively stronger in those systems containing In2S3-5, especially In2S3-10. However, the RhB matrix kept a relatively strong abundance in the system containing In2S3-15. These facts confirmed that In2S3-5 and In2S3-10, especially In2S3-10 almost decomposed RhB completely after 20 minutes of illumination.
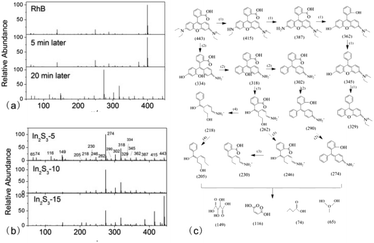 |
| Fig. 10 MS of RhB photodegradation products with In2S3-5 as the photocatalysts for 0 min, 5 min and 20 min (a) MS of RhB photodegradation products with In2S3-5, In2S3-10 and In2S3-15 as photocatalysts for 20 min (b) and the possible photodegradation path of RhB (c). | |
Next, we speculated several possible photodegradation path from the RhB matrix to small molecular products. (1) Firstly, RhB experienced a series of de-ethylation reactions, such as from m/z = 443 to m/z = 415, 387.29 Next, the intermediates were attacked by ˙OH, followed by the removal of –NH2 and the addition of –OH (from m/z = 387 to m/z = 362), then the gradual removal of –OH (from m/z = 362 to m/z = 345, 329). (2) ˙OH attacked the xanthene ring, followed by the ring opening, the removal of –N(C2H5)2, and the addition of –OH (m/z = 443 to m/z = 334),44 then the removal of –OH (m/z = 334 to 318, 302). Next, the carboxyl groups were removed partially from the xanthene ring after the loss of all ethyl groups (m/z = 302 to m/z = 290), followed by the removal of –OH (m/z = 290 to m/z = 274). (3) The intermediate product at m/z = 318 experienced a ring-opening reaction and the rupture of carbon–carbon bonds (m/z = 318 to m/z = 262),44 followed by the gradual removal of –OH groups (from m/z = 262 to m/z = 246, 230). (4) The intermediate at m/z = 262 removed the –COOH groups (m/z = 262 to m/z = 218) directly,44 followed by the removal of –NH2+ (m/z = 218 to m/z = 205). Finally, all of the obtained residues further decomposed to small molecules (m/z = 149, m/z = 116, m/z = 74, m/z = 65) through the ring-opening reactions.29,45–47 According to the above results, we speculated the possible path of RhB photodegradation (as shown in Fig. 10c).
3.3.3 Photodegradation mechanism. It is well-known that the active species in a photodegradation reaction is ˙OH (originating from the combination of holes with H2O or OH−) and ˙O2− (originating from the combination of electrons with O2) to oxidize dye molecules at the active sites. The indirect detection of active species for the photodegradation mechanism by the use of scavengers has been confirmed in many reports.48,49 Similar to those in previous literatures,50,51 isopropyl alcohol (IPA), benzoquinone (BQ), and triethanolamine (TEOA) as the scavengers were added to the system to verify the presence of ˙OH, ˙O2− and h+, respectively. Fig. 11a shows the change in the photodegradation of RhB with In2S3-10 as the photocatalyst after the addition of scavengers. Not only TEOA but BQ also suppressed the photodegradation greatly, implying that h+ and ˙O2− were the active specifies, which was attributed to the relative potential. The potential of holes (+1.28 eV, EVB in Table 1) was more negative than φ (H2O/˙OH) (+2.68 eV) or φ (OH−/˙OH) (+1.99 eV), which was unable to oxidize H2O or OH− to ˙OH. However, the potential of electrons (−0.88 eV, ECB in Table 1) was more negative than φ (O2/˙O2−) (−0.33 V), which was effective to make O2 into ˙O2−. Therefore, h+ and ˙O2− were the active specifies. Note that, although h+ could not oxidize H2O to ˙OH directly, the protonation of ˙O2− into H2O2 followed by its decomposition resulted in the generation of bits of ˙OH inevitably.52 Compared to In2S3-5 and In2S3-15, In2S3-10 produced more electrons and holes owing to higher electron–hole separation (as shown in Fig. 6), contributing to the participation of more active specifies in the reaction for faster photodegradation rate.
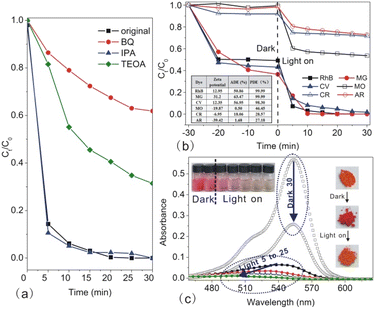 |
| Fig. 11 Photodegradation of RhB with In2S3-10 as the photocatalyst after the addition of different scavengers (a), photodegradation of different dyes with In2S3-10 as the photocatalyst (b) and absorption spectrum of RhB at different moments (c), the insets on the left and right represent the colour change of RhB solution and In2S3-10, respectively. | |
The active site is one more factor influencing the photocatalysis reaction, which is connected to interfacial adsorption. Next, we carried out the photodegradation of different dye molecules with In2S3-10 as the photocatalyst (as shown in Fig. 11b). Clearly, In2S3-10 displayed excellent photodegradation of cationic dyes such as RhB, MG, and CV. However, it showed poor photodegradation of anionic dyes such as MO, CR, and AR. It is especially noteworthy that In2S3 displayed high ADE for three cationic dyes, therefore, the excellent photodegradation was reasonably attributed to the adsorption of –OH groups on the surface of In2S3-10 for the increased hydrophilicity and surface negative charges to afford more reaction active sites. High ADE resulted in high substrate concentration for efficient decomposition of the adsorbed molecules.
Finally, we observed the colour change of the photocatalyst and substrate before and after the illumination. In the dark, the significantly darker colour of In2S3-10 confirmed that RhB was adsorbed on its surface. After turning on the light, In2S3-10 changed back to its original colour, indicating that the absorbed RhB on the surface of photocatalysts decomposed completely (as shown in the inset to Fig. 11c on the right). At the same time, RhB faded slightly in the dark, however, it turned colourless quickly after turning on the light (as shown in the inset in Fig. 11c on the left). The change in the absorption spectrum also clearly demonstrated the same results (Fig. 11c). Keeping it in the dark for initial 30 min, the peak intensity decreased without shifting of the peak position. However, after illumination for 5 min to 25 min, peak intensity decreased significantly with the gradual hypsochromic shifting of the peak position from 554 nm to 498 nm, which was caused by the step-by-step N-deethylation of RhB,53 implying that RhB matrix was decomposed by In2S3.
3.3.4 Reusability. As a catalyst, long-term stability is required to be considered in practical applications. Based on the excellent photocatalysis of In2S3-10, we carried out the photodegradation of RhB in 5 sequential cycles (as shown in Fig. 12a). With the reused times of In2S3-10 increasing, ADE decreased gradually, resulting in decreased PDE in the initial 25 min. A similar phenomenon has been reported in previous literature.54 If the illumination time was prolonged, PDE increased obviously as it improved from 74.96% to 95.94% in the case of In2S3-10 after recycling 5 times. To detect the influence of the adsorbed molecules on the property of photocatalysts, we carried out SEM image of 5-time recycled and used In2S3-10 after 25 min of illumination (as shown in Fig. 12b). Different from 500–600 nm distinct thornball-like structures assembled by thin nanoflakes in Fig. 2b, 5-time recycled In2S3-10 showed such ambiguous edges that one could barely distinguish the nanoflakes. Moreover, we measured N2 adsorption–desorption isotherms (Fig. 12c). Compared to the isotherm shown in Fig. 7b for the original In2S3-10 with a BET surface area of 26.17 m2 g−1 and maximum pore size of 30.54 nm, 5-time recycled and used In2S3-10 displayed a smaller BET surface area of 11.87 m2 g−1 and wider pore size distribution map without the maximum peak. Moreover, the hysteresis loop was invisible, indicating that the mesoporous among thornball-like structures disappeared, very likely to be filled by RhB molecules. The adsorbed high concentration of RhB on the surface of photocatalysts led to disabling adsorption of RhB in the solution, therefore, the deactivation of the reused In2S3 in the photodegradation of RhB was closely related to the reduction of the adsorption content.54 With the adsorbed RhB on the surface of photocatalysts decomposing after the illumination, RhB in the solution was further adsorbed onto the photocatalysts for decomposition. In our next work, we will try to carry out surface treatments on the reused photocatalysts to improve the adsorption of dye molecules for increased reusability.
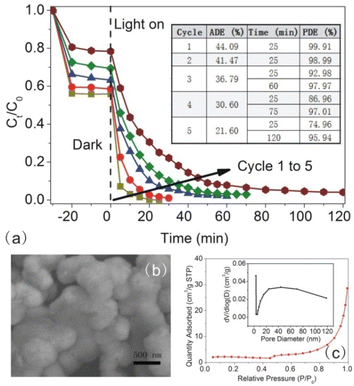 |
| Fig. 12 RhB photodegradation with In2S3-10 as the photocatalyst in sequential 5 cycles (a), the table inside (a) displayed the photodegradation efficiency of RhB for different cycle times with different illumination time, SEM (b) and nitrogen adsorption–desorption curve (c) of In2S3-10 after the photodegradation for 5 cycles, the inset to (c) was the pore size distribution curve. | |
4 Conclusions
A facile synthesis method for different-sized thornball-like In2S3 with controllable photocatalysis was reported. Three In2S3 samples within the pore size of 400–800 nm were synthesized rapidly by one-pot microwave irradiation method at low temperature in an aqueous solution. Different-sized tetragonal β-In2S3 assembled by nanoflakes displayed obviously different charge separation abilities and surface properties, resulting in various photodegradation rates of RhB. In2S3-10 showed maximum electron–hole separation efficiency and surface attraction, contributing to the complete photodegradation of RhB in just 25 min of illumination, which was possibly the fastest rate as far as we know. The extremely rapid photodegradation was attributed to the strong adsorption of dye molecules on the In2S3 surface followed by its highly effective decomposition on the substrate. MS results showed that small molecules generated after RhB experienced the process of de-ethylation, ring-opening of xanthene, and rupture of carbon–carbon bonds. Additionally, In2S3-10 displayed comparable reusability only by prolonging the illumination time.
Author contributions
Chaofan Zheng: methodology, investigation; Ziyao Wang: writing-the original draft; Jialong Yuan and Xiaoyi Lu: formal analysis; Qingfeng Xu and Haixin Li: validation; Jiangang Gao: reviewing and editing; Wenjin Yue: conceptualization, supervision, funding acquisition.
Conflicts of interest
There are no conflicts to declare.
Acknowledgements
This work was supported by Universities' Scientific Research Projects in Anhui Province (KJ2021A0498), Anhui Laboratory of Clean Energy Materials and Chemistry for Sustainable Conversion of Natural Resources (LCECSC-02), and the National Undergraduate Innovation Entrepreneurship Project in Local University (20221036306).
Notes and references
- Y. Yuan, R.-t. Guo, L.-f. Hong, X.-y. Ji, Z.-d. Lin, Z.-s. Li and W.-g. Pan, Mater. Today Energy, 2021, 21, 100829 CrossRef CAS.
- J. Arun, S. Nachiappan, G. Rangarajan, R. P. Alagappan, K. P. Gopinath and E. Lichtfouse, Environ. Chem. Lett., 2022, 1 Search PubMed.
- K. Yang, Z. Yang, C. Zhang, Y. Gu, J. Wei, Z. Li, C. Ma, X. Yang, K. Song, Y. Li, Q. Fang and J. Zhou, Chem. Eng. J., 2021, 418, 129344 CrossRef CAS.
- P. Li and T. He, J. Mater. Chem. A, 2021, 9, 23364 RSC.
- X. Li, J. Yu and M. Jaroniec, Chem. Soc. Rev., 2016, 45, 2603 RSC.
- J. Zhang, H. Wang, X. Yuan, G. Zeng, W. Tu and S. Wang, J. Photoch. Photobio. C, 2019, 38, 1 CrossRef CAS.
- W. Gao, W. Liu, Y. Leng, X. Wang, X. Wang, B. Hu, D. Yu, Y. Sang and H. Liu, Appl. Catal., B, 2015, 176–177, 83 CrossRef CAS.
- N. Yahya, F. Aziz, N. A. Jamaludin, M. A. Mutalib, A. F. Ismail, W. N. W. Salleh, J. Jaafar, N. Yusof and N. A. Ludin, J. Environ. Chem. Eng., 2018, 6, 7411 CrossRef CAS.
- L. Qi, H. Li and L. Dong, Mater. Lett., 2013, 107, 354 CrossRef CAS.
- A. K. Nayak, S. Lee, Y. Sohn and D. Pradhan, CrystEngComm, 2014, 16, 8064 RSC.
- C. Wei, W. Guo, J. Yang, H. Fan, J. Zhang and W. Zheng, RSC Adv., 2014, 4, 50456 RSC.
- T. Li, S. Zhang, S. Meng, X. Ye, X. Fu and S. Chen, RSC Adv., 2017, 7, 6457 RSC.
- L. Liu, W. Xiang, J. Zhong, X. Yang, X. Liang, H. Liu and W. Cai, J. Alloys Compd., 2010, 493, 309 CrossRef CAS.
- R. Amutha, S. Akilandeswari, B. Ahmmad, M. Muruganandham and M. Sillanpaa, J. Nanosci. Nanotechnol., 2010, 10, 8438 CrossRef CAS PubMed.
- S. Rengaraj, S. Venkataraj, C.-w. Tai, Y. Kim, E. Repo and M. Sillanpää, Langmuir, 2011, 27, 5534 CrossRef CAS PubMed.
- X. Sheng, L. Wang, G. Chen and D. Yang, J. Nanomater., 2011, 2011, 280216 Search PubMed.
- B. Xue, F. Xu, B. Wang and A. Dong, CrystEngComm, 2016, 18, 250 RSC.
- P. L. Saldanha, V. Lesnyak and L. Manna, Nano Today, 2017, 12, 46 CrossRef CAS.
- Z. Pang, M. Zhang, L. Huang, R. Wen, J. Lu, Y. Zhao, A. Wei, L. Tao, D. Luo, J. Liu, Y. Yang, Y. Xiao and Z. Xiao, Mater. Lett., 2018, 210, 66 CrossRef CAS.
- S. D. Naik, T. C. Jagadale, S. K. Apte, R. S. Sonawane, M. V. Kulkarni, S. I. Patil, S. B. Ogale and B. B. Kale, Chem. Phys. Lett., 2008, 452, 301 CrossRef CAS.
- C. R. Patra, S. Patra, A. Gabashvili, Y. Mastai, Y. Koltypin, A. Gedanken, V. Palchik and M. A. Slifkin, J. Nanosci. Nanotechnol., 2006, 6, 845 CrossRef CAS PubMed.
- Z. Wu, X. Yuan, G. Zeng, L. Jiang, H. Zhong, Y. Xie, H. Wang, X. Chen and H. Wang, Appl. Catal., B, 2018, 225, 8 CrossRef CAS.
- S. Yang, C. Y. Xu, B. Y. Zhang, L. Yang, S. P. Hu and L. Zhen, J. Colloid Interface Sci., 2017, 491, 230 CrossRef CAS PubMed.
- L. Chen, G. Yang, X. Wei, H. Xu and S. Jin, J. Alloys Compd., 2022, 895, 162589 CrossRef CAS.
- W. Wang, W. Zhu and L. Zhang, Res. Chem. Intermed., 2009, 35, 761 CrossRef CAS.
- S. Alhammadi, B. G. Mun, S. Gedi, V. R. Minnam Reddy, A. M. Rabie, M. S. Sayed, J.-J. Shim, H. Park and W. K. Kim, J. Mol. Liq., 2021, 344, 117649 CrossRef CAS.
- Q. Xu, C. Zheng, Z. Wang, Z. Zhang, X. Su, B. Sun, G. Nie and W. Yue, J. Mater. Sci., 2022, 57, 7531 CrossRef CAS.
- Q. Xu, Z. Wang, H. Yang, Y. Xiang, G. Nie and W. Yue, J. Alloys Compd., 2022, 904, 163966 CrossRef CAS.
- W. Yue, Z. Wang, J. Gong, Z. Wang and Y. Dong, Mater. Sci. Semicond. Process., 2021, 126, 105671 CrossRef CAS.
- P. Makula, M. Pacia and W. Macyk, J. Phys. Chem. Lett., 2018, 9, 6814 CrossRef CAS PubMed.
- H. Zhong, S. S. Lo, T. Mirkovic, Y. Li, Y. Ding, Y. Li and G. D. Scholes, ACS Nano, 2010, 4, 5253 CrossRef CAS PubMed.
- J. Xu, C. Liu, J. Niu and M. Chen, Sep. Purif. Technol., 2020, 230, 115861 CrossRef CAS.
- Q. Wang, J. Huang, H. Sun, K.-Q. Zhang and Y. Lai, Nanoscale, 2017, 9, 16046 RSC.
- J. Chang and E. R. Waclawik, RSC Adv., 2014, 4, 23505 RSC.
- S. B. Kokane, R. Sasikala, D. M. Phase and S. D. Sartale, J. Mater. Sci., 2017, 52, 7077 CrossRef CAS.
- R. He, K. Xue, J. Wang, T. Yang, R. Sun, L. Wang, X. Yu, U. Omeoga, W. Wang, T. Yang, Y. Hu and S. Pi, J. Mater. Sci., 2019, 54, 14690 CrossRef CAS.
- J. Chen, W. Liu and W. Gao, Appl. Surf. Sci., 2016, 368 Search PubMed.
- L.-Y. Chen, Z.-D. Zhang and W.-Z. Wang, J. Phys. Chem. C, 2008, 112, 4117 CrossRef CAS.
- J. Parhizkar and M. R. M. Shafiee, Nanochem. Res., 2020, 5, 168 CAS.
- N. M. Huang, J. Nanomater., 2011, 2011, 815709 Search PubMed.
- X. Yuan, L. Jiang, J. Liang, Y. Pan, J. Zhang, H. Wang, L. Leng, Z. Wu, R. Guan and G. Zeng, Chem. Eng. J., 2019, 356, 371 CrossRef CAS.
- C. Chen, D. Chen, X. Jiao and C. Wang, Chem. Commun., 2006, 4632 RSC.
- P. Berki, Z. Németh, B. Réti, O. Berkesi, A. Magrez, V. Aroutiounian, L. Forró and K. Hernadi, Carbon, 2013, 60, 266 CrossRef CAS.
- J. Wu, N. Qin, E. Lin, B. Yuan, Z. Kang and D. Bao, Nanoscale, 2019, 11, 21128 RSC.
- Y. A. B. Neolaka, Z. S. Ngara, Y. Lawa, J. N. Naat, D. Prasetyo Benu, A. Chetouani, H. Elmsellem, H. Darmokoesoemo and H. Septya Kusuma, J. Environ. Chem. Eng., 2019, 7, 103482 CrossRef CAS.
- M. Rakibuddin, S. Gazi and R. Ananthakrishnan, Catal. Commun., 2015, 58, 53 CrossRef CAS.
- S. Vigneshwaran, C. M. Park and S. Meenakshi, Sep. Purif. Technol., 2021, 258, 118003 CrossRef CAS.
- A. Das, M. K. Adak, N. Mahata and B. Biswas, J. Mol. Liq., 2021, 338, 116479 CrossRef CAS.
- V. H. Nguyen, L. A. Phan Thi, P. S. Chandana, H. T. Do, T. H. Pham, T. Lee, T. D. Nguyen, C. Le Phuoc and P. T. Huong, Chemosphere, 2021, 276 Search PubMed.
- T. D. Munusamy, C. S. Yee and M. M. R. Khan, Adv. Powder Technol., 2020, 31, 2921 CrossRef CAS.
- H. Wang, Z. Ye, C. Liu, J. Li, M. Zhou, Q. Guan, P. Lv, P. Huo and Y. Yan, Appl. Surf. Sci., 2015, 353, 391 CrossRef CAS.
- A. Ajmal, I. Majeed, R. N. Malik, H. Idriss and M. A. Nadeem, RSC Adv., 2014, 4, 37003 RSC.
- J. Zhuang, W. Dai, Q. Tian, Z. Li, L. Xie, J. Wang, P. Liu, X. Shi and D. Wang, Langmuir, 2010, 26, 9686 CrossRef CAS PubMed.
- S. Ge, L. Cai, D. Li, W. Fa, Y. Zhang and Z. Zheng, J. Nanopart. Res., 2015, 17, 488 CrossRef.
Footnotes |
† Electronic supplementary information (ESI) available. The details on Photocatalysis experiments (S1), Electrochemical measurements (S2) and Calculation of band edge potential based on empirical equation and C–V measurement (S3). See DOI: https://doi.org/10.1039/d2ra07108h |
‡ These authors contributed equally to this work. |
|
This journal is © The Royal Society of Chemistry 2023 |