DOI:
10.1039/D2RA06023J
(Review Article)
RSC Adv., 2023,
13, 7312-7328
Electrospun nanofibres in drug delivery: advances in controlled release strategies
Received
24th September 2022
, Accepted 14th December 2022
First published on 6th March 2023
Abstract
Emerging drug-delivery systems demand a controlled or programmable or sustained release of drug molecules to improve therapeutic efficacy and patient compliance. Such systems have been heavily investigated as they offer safe, accurate, and quality treatment for numerous diseases. Amongst newly developed drug-delivery systems, electrospun nanofibres have emerged as promising drug excipients and are coming up as promising biomaterials. The inimitable characteristics of electrospun nanofibres in terms of their high surface-to-volume ratio, high porosity, easy drug encapsulation, and programmable release make them an astounding drug-delivery vehicle.
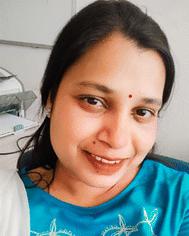 Mrunalini K. Gaydhane | Dr Mrunalini K. Gaydhane is a doctor of philosophy in Chemical Engineering from the Indian Institute of Technology, Hyderabad, India (2020). Her research area includes the design and formulation of controlled-release systems by the encapsulation of active molecules into polymeric electrospun nanofibres. The controlled-release systems include wound-healing mats, pain-relieving transdermal patches, and compressed nanofibrous oral tablets for the potential cure of leishmaniasis and mucormycosis. |
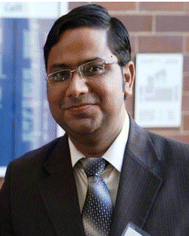 Chandra Shekhar Sharma | Dr Chandra Shekhar Sharma is a professor in the Department of Chemical Engineering, Indian Institute of Technology, Hyderabad, India. He graduated with a PhD in Chemical Engineering from the Indian Institute of Technology, Kanpur, India (2010). His areas of interest include polymer and carbon nanostructures, electrospun nanofibres for multiple applications, such as drug delivery, tissue engineering scaffolds, and bio-inspired multifunctional surfaces. |
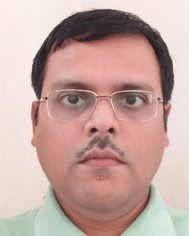 Saptarshi Majumdar | Dr Saptarshi Majumdar is a professor in the Department of Chemical Engineering, Indian Institute of Technology, Hyderabad, India. He graduated with a PhD in chemical engineering from the Indian Institute of Technology, Kharagpur, India (2008). His areas of interest include the multiscale design of biomaterials, the physico-chemical aspects of polyelectrolytes and polyampholytes, and the formulation of controlled drug-delivery systems for various applications, such as glaucoma, leishmaniasis, black fungus. |
The current review depicts the mechanism of drug release through nanofibres and emphasizes the strategies to tune their drug-release profiles for their applicability in therapeutics. It includes a detailed discussion on their pre- and post-electrospinning adaptation. Further, it discusses the common design criteria for oral, transdermal, topical, and trans-mucosal drug release through nanofibres together with the latest developments. Finally, the review unfolds the future perspectives in nanofibre fabrication to achieve programmable release encompassing the polymer-free approach, green electrospinning, herbal, and combinatorial drug delivery.
1. Introduction: significance of controlled/tuneable release
Conventional drug formulations are often allied with undesired side effects, anti-microbial resistance, and immunocompromised host. For ensuring immediate effects, drugs with a low biological half-life, such as non-steroidal anti-inflammatory drugs (NSAIDs), are delivered as a frequent bulk dosage, which can cause fluctuating drug plasma concentrations, resulting in systemic toxicity, including potentially peptic ulcers, intestinal bleeding, and damage to the gastric mucosa.1 Improvements in synthesizing technologies have enabled the synthesis of complex drug compounds. Approximately 60–70% of newly discovered drug molecules are poorly water soluble and have low permeability, causing poor absorption in the gastrointestinal (GI) tract. To enhance their bioavailability, they need to be delivered as lipid formulations.2 Another important category of therapeutic agents encompasses proteins, peptides, antibodies, vitamins, enzymes, gene-based drugs, etc., which are not suitable to be administered orally; the reason being protein based, as they undergo proteolytic/enzymatic degradation and cannot be absorbed efficiently in the systemic circulation due to their larger size.3 Many lipophilic drugs, such as immune-suppressants, HIV-1 protease inhibitors, anti-oxidants, anti-hypertensive agents, and anti-cancer drugs are well known to undergo pre-systemic metabolism.4 There are reports of some drugs being ionized by gastric acids into non-lipid soluble products and a few being inactivated in the harsh acidic environment of the stomach.5
The pitfalls associated with conventional drug-delivery systems, such as premature degradation, first-pass metabolism, discomfort or pain during administration, infusion-related toxicity, and systemic toxicity, need to be addressed.6 Thus, novel drug-delivery systems aim for controllable, programmed, sustained, or targeted drug delivery as a patient-friendly substitute. The most promising ones involve the polymeric encapsulation of drugs into nanocarriers, which can not only provide temporal control but also protect the therapeutic agent from the harsh physiological conditions.7,8
Nanocarriers, such as liposomes, nanospheres, solid-lipid or metal-based or magnetic or theranostic nanoparticles, dendrimers, micelles, and polymeric nanofibres, have emerged as astounding drug-delivery vehicles.9–12 The shape, size, composition, and surface chemistry of these nanocarriers, along with their drug interaction, aggregation, and dissolution, have been found to control the drug-release rate.8 However, due to their nano-sized diameters and other surface chemistries, such as zeta potential, most of these nano-drug vehicles are cleared in the GI tract or by the first-pass hepatic metabolism.13 In the case of nanoparticles, a high amount of drug is adsorbed on the surface, thereby undergoing a burst release.13 For most practical applications, therefore, these nano-drug vehicles further demand another carrier or ligands.6 Nanofibres are exceptions to this need due to their interconnected morphology and high drug-encapsulation efficiency, which can also withstand the harsh conditions in the GI tract, and further eliminate the surge from another carrier. Table 1 provides further insights into these newly developed drug-delivery systems, their fabrication techniques, and reported limitations.
Table 1 Comparison of various drug-delivery systems and their fabrication techniques
Type of nano-carriers and their size |
Fabrication mechanism: common techniques |
Technological advances |
Nature of the carrier |
Drug/bio-molecules |
Routes |
Limitations |
Ref. |
Micelles, 30–100 nm |
Self-assembly: dialysis, solution casting, oil-in-water emulsions |
Scalable, (since inexpensive and lower toxicity) |
Amphiphilic copolymer |
Both |
Intravenous, ocular, oral, nasal buccal |
Low stability upon dilution in to the blood stream, complex characterizations, few clinical trials |
14 |
Liposomes, 30 nm to a few micrometres |
Self-assembly: mechanical-dispersion method, solvent-dispersion, detergent-removal method |
Scalable, (only a few are approved for in vivo and clinical acceptance) |
Phospholipids |
Both |
Parenteral, ocular, transdermal, intravenous |
Low solubility, high cost, short life, leakage and fusion of drug/biomolecules, undergo oxidation and hydrolysis-like reactions |
15 and 16 |
Hydrogel, size in nano/micro/macro as per gel type |
Gelation: emulsions and moulding, crosslinking, H-bonding, grafting |
Scalable |
Water-based gel |
Both |
Oral, topical, transdermal, systemic injectable |
Poor drug loading and burst release need to be addressed, low mechanical strength, poor physical stability, very few clinical trials and in vivo studies |
17 and 18 |
Carbon nanotubes, 0.4–3 nm diameter to certain micron length |
Top down: chemical vapour deposition, electric arc discharge, laser ablation |
Not possible for industrial scale up due to high cost |
Carbon |
Both |
Injected through subcutaneous/abdominal/intravenous route |
Non-biodegradable, lack of FDA approval for CNTs, very costly fabrication, environmental impact |
19 |
Quantum dots, 2–10 nm |
Top down: molecular beam epitaxy, ion implantation, e-beam and X-ray lithography, bottom up: precipitation, sol–gel, microemulsion |
Not possible for industrial scale up due to high cost |
Semiconducting crystal |
Both |
Active and passive transport |
Toxicity, poor body clearance, synthesis protocol, manufacturing cost, environmental impact |
20 |
Nanoparticles, 1–100 nm |
Bottom up: hydrothermal route, chemical reduction, green synthesis, evaporation–condensation, sol–gel, and colloidal methods |
Limitations for scalability |
Organic or inorganic or hybrid material |
Both |
Intravenous, sub-cutaneous, inhalation spray |
Toxicity, needs testing protocols, only iron oxide nanoparticles are clinically proven and FDA approved; also, biocompatibility, drug targeting, transport and release, and interaction with biological barriers need to be investigated |
21–23 |
Nanofibres, 50–1000 nm |
Drawing: electrospinning |
Scalable (since cost effective, easily programmable, continuous process) |
Polymers/blend with inorganic materials |
Both |
Oral, transdermal, topical, buccal, and intravenous (injectable hydrogel), implantable |
Need to investigate controlled-release strategies, few only clinical trials, more focus needed for in vivo studies |
24–26 |
The benefits of selecting electrospun nanofibres lie in their scalability, cost, easy programmability, and of course controllability over the morphology.
The current review discusses and highlights the role of nanofibres as a therapeutic excipient and their customization to achieve a desired drug-release profile. When it comes to the customization or tuning of their release properties, the most versatile, programmable, and commendable nanofibre-generation technique is electrospinning. The technique is known to provide excellent control and command over the morphology, orientation, size, and the dimension of nanofibres along with various encapsulation strategies for the active pharmaceutical agents (APIs).27 It gives freedom to choose polymers from natural, semisynthetic, or synthetic origins or blends of polymers formed in aqueous or organic solvents or directly spun as a melt. To date, APIs with different bioactivities, such as anti-microbial, anti-inflammatory, cardiovascular, palliative, anti-histamine, anti-pyretic, and anti-cancer, have been successfully loaded into the polymeric nanofibres.6 The fact that nanofibres mimic the extra cellular matrix has further expanded their application in translational research and it is believed they may change the impending landscape of the pharmaceutical and biotechnological industries.28 API-loaded electrospun nanofibres are being widely explored and studied in regenerative medicines and tissue culture, skin and osteo-regeneration, enteral drug delivery, pain-relieving transdermal patches, bioactive wound dressings, implants, stents, nerve guides, and in 3D in vitro cancer models.29–34
The upcoming section unveils the theoretical background and operation of electrospinning to produce nano/microfibres.
2. Electrospinning
Electrospinning is a highly distinguished facile technique to produce polymeric nanofibres. It offers benefits over other nanofibre-generation techniques, such as drawing, phase separation, template synthesis, and self-assembly, for obtaining a controllable morphology, scalability, and ease of handling.35,36 This technique is based on the principle of electrohydrodynamics, where the drawing of polymeric threads in the diameter range of 10–1000 nm takes place under the influence of an electric field.37 The simple instrumentation comprises a syringe, syringe pump, voltage supply, and a grounded metallic conductor, as shown in Fig. 1(a). The theory behind nanofibre generation was first given by Sir Geoffrey Ingram Taylor in 1964.37
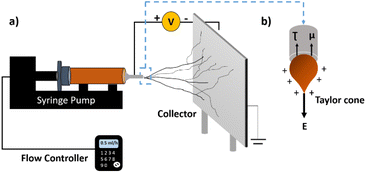 |
| Fig. 1 (a) Basic set up and (b) working principle of electrospinning, where μ is the visco-elastic force, τ is the surface tension, and E is the electrostatic force. | |
In the fibre generation process, a syringe is loaded with a polymer-based conducting solution and rested on the syringe pump assembly. As the syringe is pushed at a definite flow rate, a polymer drop exudes from the needle orifice. Under the influence of the electric field on the needle, surface tension and visco-elastic forces try to pull the polymer droplet inwards. The electrostatic forces, however, stretch the droplet outwards, forming a pendant-shaped “Taylor cone”.38 Once the threshold voltage is attained, a highly unstable polymeric jet is generated due to the repulsive-like charges. This polymeric jet travels towards the lower potential region, and during the process, the solvent gets evaporated. One-dimensional dried nanofibres are then deposited on the oppositely charged collector. This whole theory is also known as the jet instability theory.39 Though this theory looks comprehensible, several factors impact the size, orientation, and morphology of the nanofibres. The fibre formation and morphology are governed by the solution properties, electrospinning or process parameters, and ambient conditions.36–38 A brief description of the effect of these parameters on electrospinning was provided by Seeram Ramakrishna et al. (2005), and is mentioned below.25
2.1. Solution parameters
2.1.1. Molecular weight and viscosity. The molecular weight of the polymer depends on the length and entanglement of the polymer chain. A higher degree of entanglement presents more drag and intermolecular attraction, imparting a high resultant viscosity. As the solution viscosity increases, the diameter of the fibre increases. If the solution is highly viscous, then it cannot be pumped out from the syringe. The low viscous solution forms beads under high surface tension.
2.1.2. Surface tension. Surface tension decreases the surface area per unit mass of fluid, causing bead formation in fibres. Generally, surfactants are added to encourage smooth fibre formation.
2.1.3. Solution conductivity. Conductivity increases the charge-carrying capacity, stretching the polymer jet, and the high bending instability. An increase in conductivity decreases the diameter and increases the area of deposition of the nanofibres.
2.1.4. Dielectric effect of the solvent. The bending instability of the electrospinning jet increases with the dielectric constant. A greater dielectric property reduces the diameter of the nanofibres and bead formation, and increases the area of deposition.
2.2. Processing parameters
Processing parameters are external factors that act on the electrospinning jet and that are less significant than the solution parameters. The key ones are described below.
2.2.1. Voltage. A higher voltage will lead to greater coulombic forces and a stronger electric field. High voltage effectively reduces the fibre diameter and facilitates fast solvent evaporation to yield dry fibres. When a less viscous solution is used under a high electrostatic field, a secondary jet emerges from the orifice, forming fine fibres. At low voltage, the reduced acceleration of the jet and weaker electrostatic field will increase the flight time and may facilitate finer fibre formation.
2.2.2. Feed rate. As the feed rate increases, the greater volume of solution is drawn from the tip of the needle and the less flight time is available to dry the solution. An increase in feed rate increases the fibre diameter and bead size. Since less flight time is available, therefore wet fibre webs are formed because of the lower solvent evaporation. Generally, a low feed rate is favourable.
2.2.3. Temperature. An increase in temperature of the solution increases the evaporation rate and decreases its viscosity, ultimately reducing the fibre diameter. The use of high temperatures causes the biopolymers, like proteins and enzymes, to lose their functionality.
2.2.4. Effect of the collector. Generally, a conducting collector, like aluminium foil, is used because it helps in dissipating charges on the fibres and hence, allows accumulating more fibres, thus increasing the fibre packing density. In the case of a non-conducting collector, the like charges will repel each other, leading to less deposition and sometimes 3D fibres. A porous collector induces faster solvent evaporation, while a patterned collector changes the texture of the fibre mat, and a rotating collector gives an improved morphology as there is more time to evaporate the solvent.
2.2.5. Diameter of the orifice. A smaller internal needle diameter reduces clogging, the bead size, and ultimately the diameter of the fibre. However, if the diameter is too small, it will be difficult to extrude a single drop out of the needle.
2.2.6. Distance between the tip and the collector. The distance affects the flight time as well as the electric field. Decreasing the distance has the same effect as increasing the voltage.
2.3. Ambient conditions
The effects of the surrounding conditions on electrospinning have been poorly investigated. These include the following.
2.3.1. Humidity. High humidity causes the development of circular pores on the fibres. The reason behind this is because electrospinning water drops will condense on the polymer surface, leaving pores after drying.
2.3.2. Type of atmosphere. The composition of air will affect the electrospinning. Different gases behave differently under high electrostatic fields.
2.3.3. Pressure. A low pressure does not support electrospinning. If the pressure is below atmospheric pressure, the solution will flow out of the needle, causing an unstable jet initiation. As the pressure decreases, a rapid bubbling of the solution will occur.Table 2 summarizes the parameters controlling the morphology and performance of electrospinning.
Table 2 List of the parameters controlling the morphology and performance of nanofibres
Category |
Parameter |
Effect on the morphology and performance |
Solution property |
Molecular weight and solution viscosity |
• The threshold values of both parameters need to be met to commence the electrospinning or else the solution is not spinnable |
• Molecular weight↑ solution viscosity↑ fibre diameter↑ |
• Area of deposition↓, continuous and smooth fibres obtained (vice versa) |
Surface tension |
• Threshold value of surface tension needs to be met for Taylor cone initiation |
• If surface tension↑ and viscosity↑ fibre diameter↑ continuous and smooth fibre formation↑ |
• If surface tension↑ and viscosity↓, fibre formation is discontinuous and bead formation↑ |
• Surface tension↑ fibre diameter↓ |
Solution conductivity and dielectric constant |
• Solution conductivity↑ dielectric constant↑ fibre diameter↓ area of deposition↑ |
• Solution conductivity↓ dielectric constant↓ bead formation↑ |
Processing conditions |
Voltage |
• Voltage↑ fibre diameter↓ |
• Voltage↑ surface tension↑ bead formation↑ or fibre thickness↑ |
• If voltage↑ and flight time↑, fibre order↑ crystallinity of fibre↑ |
Feed rate |
• A lower feed rate is desired to obtain finer fibre formation |
• If feed rate↑ and solvent–evaporation rate↓, web formation↑ |
• Feed rate↑ fibre diameter↑ |
Temperature |
• Temperature↑ viscosity↓ fibre diameter↓ |
• Temperature↑ biofunctionality of active molecules↓ |
Effect of the collector |
• Conductive collector: dense and packed nanofibres |
• Non-conductive collector: 3D and loosely packed nanofibres |
• Patterned collector: same patterned nanofibres with the same orientation |
Diameter of the needle |
• If too small a needle diameter, the solution cannot ooze out |
• Diameter of needle↓ fibre diameter↓ bead formation↓ |
Distance between the needle tip and collector |
• Distance↓ then interconnected wet fibre formation↑ |
• Distance↑ fibre diameter↓ |
Ambient condition |
Humidity |
Humidity↑ pore formation in the fibre↑ |
Pressure |
Pressure below atmospheric: electrospinning is not possible |
Although the electrospinning set-up is simple to operate, the science behind fibre generation is highly complex. An understanding of the electrostatics, solution rheology, and solution properties is of utmost importance. The solution properties, process parameters, and ambient conditions discussed above are interdependent and influence each other during the electrospinning process.
3. Insights into the drug-release mechanism
Electrospun polymeric nanofibres are known to enhance the therapeutic efficacy of drug molecules through polymeric encapsulation and tuning of the drug-release rate. For instance, sustained drug release ensures a constant drug plasma level and maximum time in systemic circulation for the efficient absorption of drugs, thus enhancing the therapeutic index and ultimately, patient compliance.8 In literature, electrospun nanofibres have been employed to achieve various kinds of release profiles, including a fast or immediate release, sustained release, prolonged release, delayed-release, on-demand release, the release of multiple phases, and the co-delivery of multiple components based on the disease obligation.40 Such release profiles can be achieved by implementing one or more controlled-release strategies during electrospinning or post electrospinning. Before that, it is important to understand the drug-release mechanism through nanofibres.
There have been numerous attempts to understand the mechanism of drug release through nanofibres. Y. Fu et al. summarized that the release mechanism is an intricate process and a function of multiple factors.41 The factors that significantly contribute to drug release are: (a) the physico-chemical properties of the drug, (b) the structural characteristics of the polymeric matrix, (c) the release environment, and (d) the possible interactions among all these factors. To elaborate in detail, the physico-chemical properties of a drug include the solubility, stability, molecular size, charges, pKa value, and chemical interaction of the drug with the polymer. In the case of polymeric nanofibres, the structural characteristics, such as morphology, specific surface area, porosity, size, fibre entanglements, and orientation, govern the drug diffusion into the release media. Besides, the physiological conditions, such as pH, temperature, ionic strength, and enzymes existing in the media, contribute to the key factors in determining the drug-release kinetics.
For designing any drug-delivery system, it is vital to understand the interdependence of the above-mentioned parameters. Drug release through nanofibres is a diffusion-driven process, mostly following Fickian's law for biodegradable polymers and a non-Fickian analogy for non-degradable or complex polymeric systems.42 Jiaen Wu et al. (2020) reported a three-step release mechanism of ciprofloxacin hydrochloride (quinolone antibiotic drug) from PLGA-based bipolymer blended nanofibres.43 At first, when the nanofibrous mat is kept in the release media, the surface drug molecules diffuse into it. Eventually, the release media occupies the interfibrous pores, and the polymer matrix swells. Due to swelling, the release media gets more access to the inner drug molecules, and further diffusion occurs. The diffusion in the first step is in concordance with Fick's second law. In the second step, due to the fused nanofibres, slow diffusion happens. In the third step, the polymer degradation starts due to enzymatic hydrolysis. The degradation occurs at the surface and bulk, further assisting in the diffusion of the remaining surface and bulk molecules. The pictorial representation of the drug-release mechanism is described in Fig. 2.
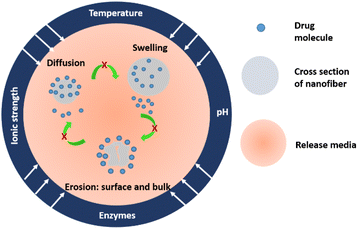 |
| Fig. 2 Mechanism of drug release through nanofibres. | |
The controlled release of a drug is important since it is economical, environmentally friendly, and patient compliable as it avoids drug level fluctuations, toxic accumulation, multiple dosages, and restricts material losses. In order to control the drug release, one needs to investigate and understand the fundamentals behind drug diffusion through nanofibres. In general, drug diffusion, the polymer-matrix swelling, and material degradation are the key mechanisms involved in the drug release from polymeric nanofibres. However, all three mechanisms do not need to exist together.
4. Drug-encapsulation and controlled-release strategies
A fine understanding of the structure–function relationship is a key to design a customized drug-delivery system.41 The drug–polymer arrangement inside the nanofibre can be directly related to the drug-release kinetics. Fig. 3 demonstrates the techniques employed for fabricating drug-loaded nanofibres.
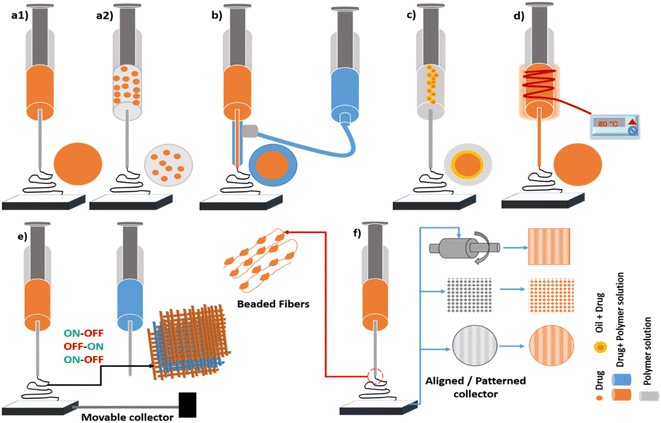 |
| Fig. 3 Drug-release strategies employed during electrospinning: (a1) solution/(a2) blend electrospinning, (b) co-axial electrospinning, (c) emulsion-based electrospinning, (d) melt electrospinning, (e) multi-layered nanofibre arrangement, and (f) beaded-aligned-patterned nanofibres. | |
The details of the pre- and post-electrospinning strategies to encapsulate the drug into the polymeric matrix and the understanding of its release behaviour are disseminated in the following section.
4.1. During electrospinning
4.1.1. Blend or solution electrospinning. Solution electrospinning is a commonly followed technique in which the drug is first dissolved (Fig. 3(a1)) or dispersed (Fig. 3(a2)) into the polymer solution and then electrospun.44 The physico-chemical and mechanical properties of nanofibres are defined by both the drug and polymer. However, meeting the equilibria between the hydrophilic and hydrophobic properties of the drug and polymer is important45 because the hydrophobic drug can accumulate on the surface of nanofibres and lead to an isolated release. The disadvantage of blending can be the burst release of the drug in most cases as drug molecules uniformly distributed on the surface of nanofibres diffuse fast. In the case of anti-inflammatory drugs, as the effect needs to be immediate, a rapid release is desired. The disadvantage of blending can be a loss of the activity of the biomolecules due to their sensitivity to organic solvents.
4.1.2. Co-axial electrospinning. The advent of co-axial electrospinning has contributed to reducing the initial burst release and introducing multiple drug releases. In the core and shell set-up, the core-side polymer generally carries the drug that has to be delivered slowly in a prolonged manner. The shell-side polymer is with or without drugs and specifically alters the drug diffusion (Fig. 3(b)). The shell-side polymer always protects the therapeutic agent inside the core from direct contact with the biological environment to avoid its degradation. Core and shell nanofibres enable the encapsulation of sensitive elements, such as proteins, growth factors, antibiotics, and other biologically active molecules.In recent approaches, Shihao Wen et al. (2019) successfully tuned the release of the dual drugs flurbiprofen and vancomycin for 9 and 17 days, respectively, by using a core–shell nanofibrous assembly.46 The anti-inflammatory, hydrophobic drug flurbiprofen was incorporated into the hydrophilic PEO polymer and placed in the shell side, while the anti-microbial drug vancomycin was incorporated into PEO/silk/collagen blend and occupied the core geometry.
In another study, tri-axial electrospinning was introduced by Liu et al. (2019) to obtain electrospun ferulic acid/gliadin nanofibres.47 Two non-electrospinnable solutions were placed in the middle (solvent) and outer layers (dilute CA), while the core solution was made up of electrospinnable ferulic acid/gliadin. After electrospinning, a thin coat of CA was formed over the ferulic acid/gliadin nanofibres. The thickness of the coating was found to determine the ferulic acid release.
4.1.3. Emulsion-based electrospinning. Emulsion-based electrospinning is an economical, flexible, and one-step process to produce core and shell nanofibres using an oil–water emulsion.44,48,49 Typically, as the name suggests, an emulsion of a drug and polymer is prepared and loaded into the syringe. The therapeutic agent or biomolecule in the aqueous phase is dispersed into the hydrophobic polymeric solution, as shown in Fig. 3(c). The ratio of the hydrophilic and hydrophobic solution affects the distribution of therapeutic agents or biomolecules.45 The bioactivity of the encapsulated therapeutic agent is preserved, as it has minimal contact with the organic solvent. However, during electrospinning, the shear force between the two phases may damage sensitive biomolecules, like nucleic acids.44 However, tuning the operating parameters can help minimize the damage. The technique is used to regulate the release kinetics and stability of therapeutic agents or biomolecules. In one such attempt, P. Coimbra et al. (2019) fabricated gentamicin sulphate (GS)-loaded PLGA nanofibres by emulsion- and suspension-based electrospinning. It was discovered that the emulsion-based GS-nanofibres showed enhanced wettability compared to the suspension-based nanofibres, as a surfactant was added to form the emulsion. Also, the burst release was controlled due to the low wettability.50
4.1.4. Melt electrospinning. In conventional electrospinning, mostly the organic and mixed volatile solvent system is specially selected to dissolve the drug and polymer. However, the drug loading is limited by its solubility in the solvent and also by the ratio of the solvent in the mixed system.51 It has been found that the residual solvents on nanofibres can be harmful for cell proliferation, thus limiting their applicability in biomedical applications.52,53 Discarding the use of solvents can be an effective alternate strategy, which additionally minimizes the cost of solvent, the solvent toxicity, recovery issues, and explosions too.54 In melt electrospinning, an additional electric heater is needed to melt the drugs and polymer and to maintain the uniform temperature during the process. Fig. 3(d) depicts the melt electrospinning set-up.He Lian et al. (2017) studied the release of curcumin (an anti-cancer drug) from PCL nanofibres fabricated by melt electrospinning (temperature = 180 °C) and solvent electrospinning (solvent = dichloromethane
:
ethanol), and compared the possible differences between the two.55 The authors found that in 12 days, nearly 50% of the drug was released from the solvent-electrospun nanofibres, whereas only 10% was released from the melt-electrospun nanofibres. The slow and sustained release through the melt-electrospun nanofibres was attributed to the high drug crystallization and comparatively smooth surface of the nanofibres compared to the solvent-based nanofibres.55 In a similar attempt, Vigh Tamas et al. (2013) demonstrated that due to the absence of solvents, the nanofibres were loosely packed;54 whereas in solvent-based electrospinning, as the nanofibres carry sufficient solvent during deposition, all the subsequent depositions were linked with each other, forming interconnected fibrous structures.56 Further, the fibre diameters of the melt-electrospun nanofibres were in the micron range.51
Compared to solvent-based electrospinning, melt-based electrospinning is economically and environmentally friendly, and hence it is termed a “green process.” In the future, reducing the fibre diameters from microns to the nano level is desired.
4.1.5. Multi-layered arrangements. Diffusional barriers in electrospun mats can be created by fabricating a multilayer assembly, as shown in Fig. 3(e).57 In one such report, a multilayer assembly of inner and outer ethyl cellulose nanofibres over curcumin-loaded gelatin nanofibres aided the sustained release of curcumin for 96 hours.58 The outer hydrophobic nanofibre coating restricted fluid penetration by imparting water-contact resistance to the inner hydrophilic curcumin–gelatin mat, which delivered all the curcumin in only 30 min when tested alone.58 The drug release could be effectively controlled when focusing on the transdermal and transmucosal routes.
4.1.6. Aligning or patterning of nanofibres. Aligning or patterning nanofibres can help control the interfibrous spacing and overall porosity, facilitating penetration and occupancy of the release media inside the nanofibrous matrix. Further, the spacing between the nanofibres changes the surface wettability too.Aligned nanofibres can be produced using a rotating mandrel as a collector (as shown in Fig. 3(f)), or by a unidirectional patterned collector or parallel electrodes.59 The patterned nanofibres can be generated by collecting fibres on patterned templates (mesh, grids, complex architecture), as shown in Fig. 3(f), or by employing patterned electrodes.60 M. Saadatmand et al. prepared different templates for the non-conducting polymer with a pentagon and tetragon geometry.61 When cetirizine (anti-histamine drug)-loaded PCL nanofibres were deposited on these non-conducting templates, patterned cavities with random deposition were formed. The transdermal drug-release studies demonstrated a burst and immediate release profile, since the spacing between the pattern was more, i.e. in the millimetre scale. However, in a study by Adepu et al. (2017), micropatterned drug-loaded nanofibres with 100 μm spacing and a cup-like geometry in a mesh gave a zero-order release for 12 h (65% release),1 showing spacing of the patterns matters.
4.1.7. Beaded nanofibres. Often undesirable and rejected during the fabrication of drug-loaded nanofibres, beads can act as a drug reservoir too. Tingxiao Li et al. (2019) explored and validated the potential of beads on string nanofibres made up of PLGA, as an excipient for micro-level solid drug particles of tetracycline hydrochloride (antibiotic drug).62 As per the study, the bead number and bead diameter were crucial factors in drug release. The bead number defines the encapsulation capability or drug loading capability, whereas the bead diameter or size majorly contributes in determining the drug-release kinetics.62 Bigger sized beads were found to lower the initial burst release, whereas, an increased number of beads increased the total drug release. Thus, a uniform-sized bead distribution is desired to estimate the drug-release mechanism. Regarding the uniform fabrication of beads, Tugba Eren Boncu et al. (2020) illustrated the interdependency of the solution parameters (i.e. a low viscosity, low conductivity, high surface tension, and low polymer concentration) and process parameters (i.e. a lower voltage, high flow rate, and small distance between the collector and needle orifice).63 For a less viscous solution with lower conductivity, uniform circular beads were formed in one of the studies due to an insufficient elongation of the polymeric jet by an electrical force.44,63 However, when the viscosity gradually increased, then spindle-shaped beads were formed (refer to Fig. 3(f)).44The most engaging feature of beads on string nanofibres is the formation of 3D nanofibres with macro-pores, which paves a way for regenerative medicine.62 Tingxiao Li et al. (2020) successfully encapsulated bovine serum albumin with growth factors in the core side into beaded nanofibres of polylactic acid-co-ε-caprolactone (shell side). The as-prepared scaffold showed good attachment and the spread of human mesenchymal stem cells onto the surface of the core and shell nanofibres with the sustained release of growth factors.64 Compared to the bead-free nanofibres, the mechanical strength of beaded fibres is less due to the decreased cohesive force between the nanofibres.63 This can be significantly resolved by using polymer blends.
4.2. Post electrospinning
The post-electrospinning operation involves the immobilization of the drug or biomolecules or enzymes onto the pristine polymeric nanofibres and surface modification of the drug-loaded polymeric nanofibres. Fig. 4 shows the post-electrospinning operations that can be employed to immobilize the active molecules and control their release.
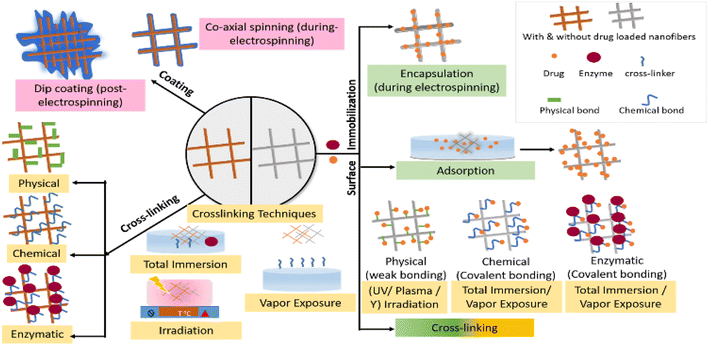 |
| Fig. 4 Post electrospinning strategies involving drug/enzyme immobilization and controlled release, comprising surface immobilization, crosslinking, and coating. | |
4.2.1. Surface immobilization. As electrospinning utilizes an organic solvent system that may be toxic, it can be a challenge to incorporate a few drug molecules, such as solid drug particles, natural or bioactive drugs, and biomolecules such as polypeptides, proteins, vitamins, enzymes, and antibodies. Those biomolecules that may lose their activity during solution preparation can be incorporated into the nanofibres in three different ways: encapsulation, adsorption, and covalent bonding,65,66 refer to Fig. 4 for further insights.
4.2.1.1 Encapsulation. This is the process in which drugs or biomolecules are entrapped in the polymeric matrix. The biomolecules are suspended uniformly into the spinning solution and get immobilized after electrospinning. For instance, Xiaobo Chen et al. (2019) loaded gentamicin (antibiotic drug) into mesoporous silica nanoparticles and suspended them in PCL solution before electrospinning. The entrapped drug could be released steadily, and a ∼20% release was achieved after 24 h compared to ∼65% without immobilization.67
4.2.1.2 Adsorption. Adsorption is the most common way to immobilize drugs and biomolecules. It involves a two-step process. First, the drug/biomolecule and electrospun mat are dipped in a solution for a fixed time. Due to the high surface area and porous structure of the mat, the solution penetrates in to the innermost fibres and then surface adsorption of the drug/biomolecule occurs. In the second step, the mat is rinsed with buffer solution to remove unadsorbed molecules. The weak van der Waals forces or hydrophobic and electrostatic interactions hold the adsorbed molecules.66 Although adsorption is inexpensive, reagent-free, and easily reversible, weak bonding also lets it gets desorbed fast with a change in the temperature, pH, or surface energy. Thus, for rapid delivery, adsorption is the easiest immobilization technique.
4.2.1.3 Covalent bonding. The interactions between the functional groups of biomolecules with the functional groups of polymers can produce stable complexes. Mostly, biopolymers with -amino, -carboxylic, -thiol, -imidazole, -indole, and -hydroxyl groups are involved in covalent bonding.66 The bonding can be done either by direct reaction or by activation through a crosslinker. In one study reporting the immobilization of bromelain enzyme onto cellulose triacetate, the enzyme was immobilized on nanofibres through glutaraldehyde. For comparison, the enzyme was also blended with the polymer in an organic solvent system of acetone and di, methyl formamide, and it was found that 90% enzymatic activity was lost due to exposure to the organic solvent system post-electrospinning.68 Thus, covalent bonding has benefits over surface immobilization; however, use of inappropriate chemical crosslinkers can also reduce the bioactivity.
4.2.2. Crosslinking. Mostly, nanofibres derived from natural-based polymers require crosslinking to enhance their water resistance and thermo-mechanical characteristics. Crosslinking means linking polymer chains, which alters the functionality of the polymer. It can be done in three distinct ways: physical, chemical, and enzymatic crosslinking.69,70 Physical crosslinking involves using a high-energy electron beam, UV/γ irradiation, plasma exposure, or de-hydrothermal treatment.71 For example, de-hydrothermal treatment of drug-loaded PVA nanofibres causes a condensation of water molecules and crystallization of drugs.72 This aids in the slow release of drug molecules. Plasma exposure generates an oxygen radical that reacts with water and removes it from the polymer.73 To determine the most suitable strategy, it is significant to understand the structure and functional groups of the polymer. Chemical and enzymatic crosslinking are similar to covalent bonding in the presence of a crosslinker/enzyme, as explained in Section 4.2.1.
4.2.3. Coating. An applied coating can act as a diffusional barrier to control the initial drug release. Yaoyao Yang et al. (2019) demonstrated that a cellulose acetate coating of 11.6 μm could prolong ibuprofen release from core (ibuprofen–gliadin) and shell (cellulose acetate) nanofibres, from 23.5 to 44 h.74 As mentioned earlier, the thickness of the coating plays an important role.Apart from this, the coating can protect the bioactivity of drugs and also provide sliding/flexibility while passing through the GI tract. Tao Hai et al. (2019) demonstrated that a lipid coating on berberine hydrochloride-loaded ethyl cellulose nanofibres could reduce the burst release of the drug from 65% to 40% in the initial 4 h, while also enhancing the anti-microbial activity of berberine compared to the case without the coated nanofibres.75
As we are aware, most polymers do not possess any specific functional groups, so they must be functionalized for successful specific applications. The current section highlights the techniques to functionalize polymeric nanofibres, such as, by using particular encapsulation techniques to load the active molecule or by employing surface modifications after nanofabrication. These specific techniques, such as blending, coating, grafting, crosslinking, or plasma exposure, need to be properly controlled to prevent the nanofibre morphology being distorted. Moreover, the functionalized nanofibres should not be toxic to the user or the environment.
5. Drug delivery through nanofibres in different routes
In any route of drug administration, the drug has to cross biological membranes before entering the systemic circulation or getting into the direct diseased site. While targeting specific routes, the physiological conditions must be considered, and accordingly, the properties of the nanofibres should be tuned to fit these conditions. Fig. 5 highlights the physiological conditions in the oral, transdermal, and buccal routes. The following section delineates the suitability of nanofibres for a particular route and the further customization required to achieve controlled release.
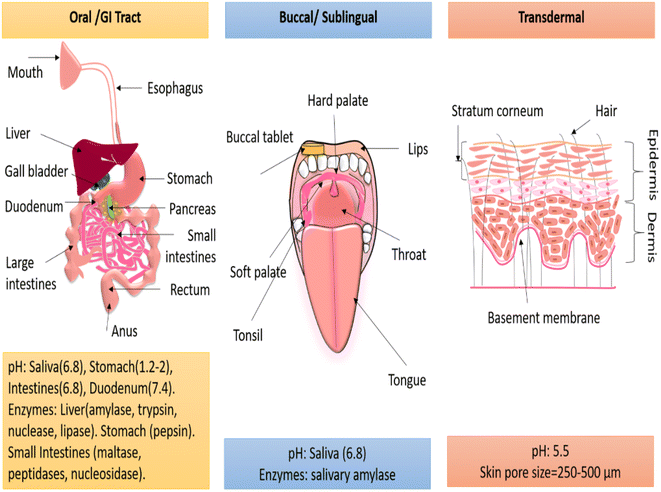 |
| Fig. 5 Physiological conditions in the oral or GI tract, buccal/sublingual, and transdermal routes. | |
5.1. Buccal or sublingual route
In the buccal or sublingual region, the moist environment, flexible tissues, and slippery texture cause a lower retention of conventional formulations.76 However, drug-loaded nanofibres, by virtue of their high specific surface area and mucoadhesive properties, can effortlessly deliver drugs in the buccal or sublingual regions.77 The drug absorbed by the highly vascularized buccal mucosa and sublingual region can directly enter into the systemic circulation, eliminating the need for passage through the GI tract and therefore bypassing the influence of enzymes, gastric acids, and the first-pass effect.40,77 This route is especially suitable for delivering drugs with poor solubility and low bioavailability. Jinghan Li et al. (2020) delivered the carvedilol (beta-blocker used to treat heart failure and hypertension) drug, which is known to have poor aqueous solubility and 25% bioavailability, by the sublingual route immediately from polyvinyl pyrrolidone PVP/PEG-400 nanofibres.40 The amorphous nature and hydrophilicity of the nanofibres could ensure the release of 80% carvedilol in 30 min from nanofibres, while only 10% of the drug was released from the physical mixture alone.40
Emese Sipos et al. (2019) fabricated the oral-dissolving nanofibrous web-releasing aceclofenac (an anti-inflammatory drug with a low biological half-life) within a minute from trolamine/PVP nanofibres.77 The improved wettability and high dissolution of trolamine–PVP aided immediate drug release.77 Thus, buccal or sublingual drug delivery provides a plausible way to deliver poor-water-soluble drugs, non-steroidal anti-inflammatory drugs, antibodies, and peptide-based formulations, either for systemic drug delivery or for treating oral diseases. Further, buccal or sublingual delivery can be used for old-aged people and children who face difficulties swallowing.
5.2. Oral/GI tract route
The oral route is the most preferred route for drug administration but has a highly complex physiology. In the GI tract, the drug must penetrate through the epithelium and the innermost lining of the entire lumen. The epithelial cell barrier selectively permeates the drug to the underlying tissue compartments via three mechanisms: transcellular diffusion, para-cellular diffusion, and receptor or carrier-mediated diffusion.78 Furthermore, after an oral administration, the drug has to survive through variable pH conditions and enzymatic degradation, as shown in Fig. 5. Polymeric encapsulation, a high surface-to-volume ratio, and a nano topography aid systematized drug diffusion along with their biostability in gastric acids and enzymes. For instance, Mohammadreza Rostami et al. (2019) developed a resveratrol (anti-cancer phytochemical)-loaded chitosan/gellan polymeric nanofibrous system to cure GI or colon cancer. The afore-mentioned phytochemical had low bioavailability and a low biological half-life. The biopolymer chitosan was blended with gellan gum to enhance the mucoadhesive and non-digestible properties. The drug release in the stomach and intestine pH conditions (1.2 and 6.8, 7.4) was subsided due to polymeric encapsulation. The cell viability was found to be a function of the drug concentration.79
Further, to achieve slow drug release in an acidic environment, Serdar Tort et al. (2020) introduced self-inflating nanofibres by generating an effervescence effect in acidic pH conditions.80 A solution of NaHCO3-PEO was casted as a thin film, and a disc was placed in between pramipexole (a drug for Parkinson's disease)-loaded eudragit-based nanofibres. The disc was inserted when half of the solution was electrospun and the other half was deposited as a nanofibre on the disc to form a pouch. This pouch, when tested for drug release in realistic pH conditions, showed a slower release rate. Due to the floating effect, only the downside drug-loaded nanofibres were in contact with the release media, which means the exposed nanofibre surface was restricted.80
In terms of the material point of view, the oral system demands biocompatible polymers. However, most biopolymers, like polypeptides and polynucleotides, are susceptible to enzymatic hydrolysis, and hence an intelligent strategy must be employed to achieve controlled release.6 For studying the oral drug delivery of amphotericin B (an antifungal and anti-leishmanial drug) through gelatin nanofibres at realistic pH conditions, Gaydhane et al. (2020) employed three novel strategies. First, the drug-loaded nanofibres were crosslinked with glutaraldehyde vapours (25% aqueous) for 6 min, then a rolled mat was obtained and compressed using a hydraulic press to obtain a nanofibrous tablet, and later the tablet was again crosslinked and coated with alginate. These indigenous strategies could result in zero-order drug release in 96 h by controlling the drug diffusion. The enzymatic degradation was evaluated under acidic pH in the presence of pepsin, and only 6% degradation was observed in the first 4 h. Also, acid-cured gelatin was protonated in acidic pH conditions, hence the water dissolution could not happen.81,82 In a similar approach, Marilena Vlachou et al. (2019) developed a furosemide (a diuretic drug to treat kidney failure)-loaded eudragit polymer-based nanofibrous tablet using hydraulic press with a 10 mm die set.83 The compression of the nanofibres restricted fluid penetration and hence achieved a slower release.83,84
Thus, the drug-loaded nanofibres could be customized to cure systemic diseases, colon cancer, or gastric infection.78,85
5.3. Transdermal route
This route utilizes the permeability of the stratum corneum, an upper layer of skin epidermis with porosity ranging from 250–500 μm and a thickness of 20–25 μm.86,87 As the membrane is semipermeable and has endogenous lipid and sebum present, it generally influxes hydrophobic drugs.86 Nanofibres, due to their high porosity, high specific surface area, and moisture permeability, have proven to be able to deliver hydrophilic drugs too in a controlled manner by implementing smart strategies.88,89 Gomaa El Fawal et al. (2020) used ethosome, a phospholipid nano-vesicle, as a carrier for fluorescein iso-thiocyanate (allergen drug) and a transdermal drug-transport enhancer.90 The drug-loaded ethosome and PVA solution was electrosprayed on PVA/HEC nanofibres. The drug release through the rat's dorsal skin showed an increased drug release (45% in 10 h) compared to without ethosome strategy (25% in 10 h). Apart from ethosome, the authors selected HEC polymer to enhance the adhesive properties of PVA. Similarly, A. Gencturk et al. (2017) blended hydroxypropyl cellulose to impart hydrophilicity to polyurethane-loaded donepezil hydrochloride (a drug for Alzheimer's dementia).91
The most explored application of transdermal delivery is wound healing and skin regeneration.92–94 The use of nanofibres, apart from drug release, can anchor the ruptured cells and direct cell differentiation. By maintaining optimum moisture in the wounded bed, the use of nanofibres has been found to reduce scar formation.30,95 Recently, Pranbesh Sasmal et al. (2019) reported a first-ever approach in developing a tranexamic acid (antifibrinolytic drug)-loaded chitosan/PVA nanofibrous membrane to treat haemorrhage.96 The drug release was found to be ∼90% within 10 h due to the additional hydrophilicity of PVA. The captivating part of this study was that the whole blood clotting was achieved in only 170 s96
Thus, nanofibrous transdermal drug delivery offers an easy, non-invasive, self-administrative, and patient-friendly route.87,90 It is a simple practice for drug administration via the skin for systemic and local effects. Keeping in mind the local toxicity, the dosage forms can also be reduced.
5.4. Injectable nanofibres
Injectable nanofibres are suitable for the localized delivery of personalized therapeutics, such as reparative medicine, bone regeneration, cancer treatment, and tissue engineering scaffolds.97 Hydrogels serve as a solution for carrying the suspended drug-loaded nanofibres.98 Drugs like doxorubicin (anti-cancer drug) and desferrioxamine (iron-chelating agent) have been reportedly delivered at tumour sites via silk nanofibres loaded into the hydrogel.99,100 Soon after injection, the hydrogel solidifies and releases the drug as tailored by the pH condition. Wei Liu et al. (2014) elaborated that for traditional bone grafting, an open surgical procedure is usually followed, which is painful, forms scar post-surgery, and takes a long recovery time.101 In such a case, the use of an injectable hydrogel incorporating nanofibres can represent a novel biomimetic bone substitute.
A fascinating feature of injectable nanofibre loaded hydrogels is that they combine the properties of both hydrogels and nanofibres, such as super hydrophilicity, high water-holding capacity, good biocompatibility, and enhanced mechanical strength and structural stability.73,102,103 Whereas, plain hydrogels possess poor mechanical strength due to their 90% aqueous portion. Dan Kai et al. (2012) illustrated that when the PCL nanofibre loading was increased to 25% in the gelatin hydrogel, the composite displayed a higher modulus and compressive strength along with improved cell proliferation.98
Nanofibres can be loaded into the hydrogel in various steps. Juliana Ribeiro et al. (2020) first fabricated ciprofloxacin-β-cyclodextrin nanofibres and then cryo-cut the mat and freeze-dried it in polydioxanone to obtain short nanofibres. Later, these short nanofibres were dispersed into a gelatin methacrylol hydrogel. The injectable hydrogel delivered the loaded antibiotic at a sustained rate for treating periodontal disease and pulpal pathology.97
This section discusses the design considerations with respect to the physiological conditions in the oral, buccal, transdermal, topical, and parenteral routes. Their smaller size, easy drug encapsulation, ability to mimic the extra cellular matrix, and biodegradability make polymeric nanofibres as an interesting carriers for different administration routes. However, the drug interaction with the biological barriers, and its absorption, solubility, and bioavailability determine its fate for clinical acceptance.
6. Future challenges and state of the art
Although numerous strategies are being executed and have been formulated to attain customized drug delivery, the focus is on achieving inexpensive, biocompatible, patient-friendly, and environmentally friendly drug-delivery systems. The following are the neoteric aims being explored in this direction.
6.1. Green electrospinning
When it comes to large-scale production, the material and production cost, and societal and environmental concerns need to be considered.104–106 Basically, a “green” approach is anticipated. Earlier, melt electrospinning was described as a “green process”. Other alternatives with probable pros and cons are discussed below.
6.1.1. Green solvent. In electrospinning, a suitable solvent system is required to dissolve API and polymers. Often these volatile solvents are toxic, hazardous to the environment, and difficult to recover. During electrospinning, solvent vaporization takes place, and the vapours accumulate in the closed compartment. These vapours can corrode the metallic set-up, entrap into the pores of drug-loaded nanofibres, and can harm the operator after opening the doors.106 Considering these issues, water-based solvent systems have the potential to offer the least environmental hazards most economically.107 The noted green solvents, apart from DI water, are acidic PBS, skimmed milk, and limonene, which is an extract obtained from orange peels.107 Water-soluble polymers, such as PVA, PEO, PVP, gelatin, polyamic acid, hydroxypropyl cellulose, kefir, dextran, and many more, can be considered for electrospinning.72,107 These polymers with fast water-dissolution properties could be ultimately utilized in the immediate or fast release of drugs, preferably in the buccal or sublingual routes.106A few water-soluble polymers, such as polyelectrolytes, are not spinnable. In such cases, additives and salts can help. Songnan Li et al. (2020) fabricated nanofibres from an aqueous dispersion of modified starch and octenylsuccinated, and introduced pullulan polysaccharide to improve the adhesive/blending properties of the solution.108 Furthermore, the water-soluble polymers face poor mechanical and thermal characteristics, which need to be improved. For tissue engineering of scaffolds and wound dressings, the mechanical strength is a decisive parameter. Daqian Gao et al. (2020) implemented a “green crosslinking” approach to improve the water resistivity and mechanical strength of PVA nanofibres loaded with epidermal growth factor derivatives as a wound-dressing biomaterial.109 Before the loading of the growth factor, the PVA nanofibrous mat was crosslinked in ethanol solution for 24 h and later heated at 120 °C for 2 h.109 Another approach is the blending of water-soluble polymers with synthetic polymers. Dalila Miele et al. (2020) blended collagen and PCL using an acidic aqueous solvent system as a green approach for improving the mechanical strength.110
6.1.2. Green polymer. The future of sustainable nanotechnology is feasible only with the best use of green materials. Here, biopolymers can serve as an affordable, easily available, non-toxic, biocompatible, biodegradable, and environment-friendly polymer source. To mention a few, these encompass alginate, chitosan, hyaluronic acid, kefiran, pullulan, tree gum, gellan gum, zein, soy protein, and wheat protein, which are derived from natural sources.105 In recent times, polysaccharides and protein-based polymers have received abundant attention. However, these biopolymers have poor conductivity and mechanical properties, and hence they are mostly blended with synthetic polymers. In blended nanofibres, a combination of the properties exhibited by the individual polymers is observed. Wherein, the addition of synthetic polymers can improve the water stability and thermo-mechanical characteristics of a final nanofibrous matrix, biopolymers provide biocompatibility and controllable biodegradability. In a study carried out by Meera Moydeen Hameed et al. (2020), core–shell nanofibres were fabricated with cephalexin (antibiotic), and corn oil as the core and PVA were blended in 90
:
10 ratios with four kinds of biopolymers, namely chitosan, carboxymethyl cellulose, carboxymethyl starch, and hydroxypropyl cellulose, respectively. After thermal crosslinking at 120 °C for 6 h, crystallization occurred. The in vitro release studies demonstrated that the nature of the biopolymer blend and its inherent interaction with the second polymer could significantly control the drug-release kinetics.72
6.1.3. Crosslinker-free approach. In drug delivery and tissue engineering, biopolymers are exploited as drug excipients or scaffolds, owing to their cytocompatibility and non-toxic degradation in the system.111,112 However, FDA-approved biopolymers, such as gelatin, chitosan, and alginate, often face limitations due to the use of chemical crosslinkers, such as glutaraldehyde, which is toxic. Although a few works have reported minimizing the use of crosslinkers either by lowering their concentration or time of exposure, a crosslinker-free approach would always be welcomed.57,81 We can exclusively highlight the crosslinker-free drug-release studies carried out by Bhutani et al. with gelatin-based hydrogels, which could be implemented with nanofibre-based drug-delivery systems too.113–116 In one such approach, a deseeded cardamom husk, a natural shell with crude fibres, with a proper incision at the top, was encased with a drug-gelatin hydrogel. The open end was plastered with sodium alginate viscous solution and dried. This construct was found to control the initial burst release and could achieve the zero-order release of the hydrophilic drug naproxen and hydrophobic drug piperine when loaded independently.114 Further, the cardamom husk was stable in physiological conditions. Thus, a crosslinker-free, natural capsule of cardamom husk was innovatively used to load a realistic drug dosage into the gelatin-based hydrogel to enhance the stability of the drug–polymer matrix in the harsh acidic environment of the stomach.114 Next, what about introducing a false crosslinker? The same group developed gelatin-naproxen tablets using a mould and then coated the surface with piperine drug molecules through a solvent–evaporation technique. Piperine, known for enhancing the bioavailability of most drug molecules, is hydrophobic. It formed covalent bonding with gelatin and then was further coated with sodium alginate film to impart the water resistance. This compelling assortment remarkably helped to omit the use of glutaraldehyde in return, enhancing the water-dissolution resistance of gelatin in acidic pH 1.2 (stomach) and achieving the zero-order release of naproxen.116These examples can be ultimately utilized for developing a gelatin nanofibre-based drug delivery. The use of natural resources, like cardamom husk, is a brilliant alternative for oral drug delivery.
6.2. Herbal- or phytochemical-based drug delivery
Due to an upsurge in the complexities associated with synthetic drugs, such as anti-microbial resistance, lethal side effects, inappropriate systemic clearance, and cytotoxicity, it is high time to develop phytochemical-based drug delivery.117–119 As evidenced by various studies, herbal drugs are easily adaptable to the human body and offer minimum side effects. The numerous components in herbal extracts can act synergistically and offer a complete solution to a disease, unlike synthetic drugs.120 The polymeric encapsulation of herbal drugs into nanofibres in the form of extracts, active components, and essential oils have been investigated for varied applications, such as wound-healing mats, drug-delivery systems, and implants.26,121 In recent times, phytochemical-loaded nanofibrous patches have been explored and tested for effectual chemotherapy after cancer surgery to prevent relapse. Rasouli et al. (2020) encapsulated the plant anti-oxidants curcumin and chrysin simultaneously into a PLGA/PEG blend. The obtained nanofibres exhibited enhanced anti-cell proliferative activity against breast cancer cells compared to only curcumin-loaded nanofibres.122 Thus, phytochemical-based drug delivery can offer the safest alternative to chemotherapy and radiotherapy, which traditionally can cause adverse side effects and affect the life quality of cancer patients.
6.3. Combinatorial drug release
Electrospinning is a programmable technique that enables the delivery of two or more drugs simultaneously or separately either via blend, emulsion-based, co-axial electrospinning, or a multi-layered construct.123 Combinatorial drug delivery is an advantageous formulation capable of reducing the associated cost of monotherapy, the dosage forms, toxicity, side effects, and anti-drug resistance.124 Especially in chemotherapy, the use of a single drug is typically avoided as high doses can be toxic, and the heterogeneity of cancer cells may develop drug resistance.125 Considering this, Huijun Li et al. (2017) developed a core and shell nanofibrous patch loaded with synthetic and natural drugs to demonstrate the significance of dual drug delivery for treating cancerous cells.126 First, the hydrophobic drug curcumin was loaded into regenerated silk fibroin (RSF) solution and precipitated in ethanol to obtain nanospheres through self-assembly. Next, the hydrophilic drug doxorubicin hydrochloride was blended with aqueous RSF to form the shell side of the nanofibre. To tune the wettability, the water annealing of the nanofibrous mat was performed at 45 °C and 60 °C, respectively. The annealed sample at 60 °C gave a simultaneous and sustained release of drugs to treat breast cancer or skin cancer. Here, it is noteworthy to employ natural and synthetic drugs for combinatorial therapy, whose synergistic action can enhance the therapeutic efficacy as well as safety and biocompatibility.
6.4. Polymer-free drug release
The polymer forms the core of the drug-delivery systems. However, in electrospinning, when the polymer and drugs are not compatible, either physically or chemically, the polymer-free approach can be a new alternative. In a few attempts made with electrospun nanofibres for drug delivery, β-cyclodextrin, a cyclic derivative of partially hydrolyzed starch obtained by enzymatic hydrolysis, has been used as a drug excipient.127 This oligosaccharide has a cone-shaped molecular structure in which the inner side is hydrophilic, and the outer side is hydrophobic.128 This structure makes it easier to form an inclusion complex with numerous hydrophobic drug molecules.129 Hydrophobic drug delivery is a challenge for pharmacists since the drug is poorly absorbed in the body due to its solubility issues. Forming an inclusion complex with cyclodextrin not only improves its solubility and bioavailability but also masks the unpleasant smell of API and reduces tissue irritation.54 One more advantage of using cyclodextrin is, more drugs (w/w %) can be loaded compared with the polymer-based system and also an aqueous solvent system is utilized.130 Vigh et al. (2013) fabricated spironolactone (diuretic drug)-loaded hydroxypropyl-β-cyclodextrin nanofibres and achieved an immediate release within a minute.54 In another example, Zehra Irem Yildiz et al. (2017) further achieved the fast dissolution of poorly soluble sulfisoxazole (anti-microbial drug) through cyclodextrin-based nanofibres.129
As a polymer-free system, it may undergo first-pass metabolism and a decayed bioactivity; hence, cyclodextrin-based nanofibres can be used in buccal or sublingual administration.
7. Future directions
The past two decades have witnessed extensive qualitative research in the fabrication and development of electrospun nanofibres as drug-delivery vehicles. It has been explored and validated by some brilliant strategies to encapsulate and deliver a vast range of APIs, encompassing herbal actives, proteins, vitamins, enzymes, DNA, RBCs, genes, etc., with the desired kinetics. The past section highlighted some recent and emerging trends comprising green, herbal, combinatorial, and polymer-free drug-delivery approaches. Still, many hurdles remain before we can foresee nanofibrous drug delivery in actual use. At present, many researchers have reported proof-of-concept system. However, with the few exceptions of use in wound-healing nanofibrous mats, such as Rivelin®, ReDura™, NeoDura™, SurgiClot®, and Heal Smart™, most of the findings have not yet reached the clinical trials.
For oral or buccal drug delivery through nanofibres, it is highly needed to address some existing issues, including drug release in a therapeutic window, loading a realistic drug dosage, applying realistic in vitro conditions by considering enzymatic degradation of the carrier polymer or the drug, and maintaining a constant release profile. Moreover, the cytocompatibility studies performed to date have been limited to qualitative investigations, such as cell proliferation, adhesion, and differentiation. Hence, quantitative analysis focusing on the amount of drug uptake by cells, drug intercalation, and interaction should be performed. While fabricating oral and buccal nanofibrous patches or tablets, it is recommended to encapsulate or coat these with sweetening agents, such as honey or sugar, to make them more palatable. Coming to the transdermal route, the drug release through nanofibres have been found to be inefficient due to the low diffusion of drug molecules through the skin pores and an improper contact of the patch and skin. Introducing an adhesive gel may be a solution to enhance drug diffusion and maintain local skin contact. In a similar way, as highlighted earlier, the parenteral administration of drug-loaded nanofibres via injectable hydrogels has a great future in treating benign tumours, internal wounds, ulcers, and ocular disorders. Hence, there is scope to exhilarate this domain and identify the hurdles for forming a competent targeted drug-delivery system. Apart from this, the scalability or mass production of drug-loaded electrospun nanofibres needs to be resolved. Modern technology exercising multiple jets and multi-nozzles arrangements come in handy in this regard to facilitate mass production. There are a few prototypes that have been reported to achieve mass production involving needleless electrospinning, near field electrospinning, free-surface electrospinning, and bubble electrospinning approaches. It may be often doubted, but numerous companies are working in the field and supplying nanofibre-based products. A proper tie-up between academia and the industrial sector would surely help in this regard.
8. Conclusions
Developing an economical and environmentally friendly, sustainable drug-delivery system with a high therapeutic index and patient compliance is the ultimate goal of pharmaceutical and biotechnological industries. Electrospinning offers the easy incorporation of API into the polymeric nanofibres, either by direct blending, emulsifying, co-spinning, or immobilizing into the nanofibres. The polymeric encapsulation of API can ensure a uniform spatial distribution and conservation of the therapeutic activity. Implementing various strategies to control the drug diffusion during and post electrospinning is possible to tune the drug-release mechanism. Further, considering the physiology and biological barriers imposed by different routes, this review provides a number of ingenious strategies to tune the physico-chemical properties and biocompatibility of drug-loaded nanofibres. Thus, with its morphological, compositional, and structural benefits, electrospun nanofibres offer programmable release as per the disease obligation.
This review highlighted the capabilities of electrospun nanofibres as a promising drug-delivery vehicle endowed with various personalized adaptations befitting the pre, post, and during the electrospinning process to achieve controlled release. It later considered oral, buccal, transdermal, and parenteral routes of drug administration and suggested the prerequisite measures to achieve desired drug release through nanofibres. It particularly discussed the newer trends in fabricating oral or buccal nanofibrous tablets, capsules, transdermal patches, and injectable hydrogels with a focus on achieving realistic drug dosage, maintaining real physiological conditions, and investigating drug-release mechanisms.
Finally, the impending challenges and the latest emerging solutions in customized drug delivery focusing on herbal-phytochemical, combinatorial, and polymer-free drug-release approaches were discussed. This review concluded with green electrospinning as an imminent novel drug-delivery system. The ultimate prominence of sourcing green polymers, green solvents, herbal drugs, and a crosslinker-free approach has been emphasized for achieving a compliant, safer, and quality assured healthcare system.
Author contributions
MKG: conception or design, data collection, literature survey, writing and drafting, communication. CSS: conception or design, editing, critical revision, final approval. SM: conception or design, editing, critical revision, final approval.
Conflicts of interest
There are no conflicts to declare.
Acknowledgements
The authors acknowledge the DST-Nano mission grant (SR/NM/NS-1006/2016) for financial support.
Notes and references
- S. Adepu, M. K. Gaydhane, M. Kakunuri, C. S. Sharma, M. Khandelwal and S. J. Eichhorn, Appl. Surf. Sci., 2017, 426, 755–762 CrossRef CAS
. - S. Gupta, R. Kesarla and A. Omri, ISRN Pharmacol., 2013, 2013, 1–16 CrossRef PubMed
. - W. Anonymous, 2020.
- Z. Zhang, F. Gao, S. Jiang, L. Ma and Y. Li, Curr. Drug Metab., 2012, 13, 1110–1118 CrossRef CAS PubMed
. - Anonymous, Gastric drug absorption, https://derangedphysiology.com/main/cicm-primary-exam/required-reading/pharmacokinetics/Chapter134/gastric-drug-absorption, accessed 31 October 2020.
- E. J. Torres-Martinez, J. M. Cornejo Bravo, A. Serrano Medina, G. L. Pérez González and L. J. Villarreal Gómez, Curr. Drug Delivery, 2018, 15, 1360–1374 CrossRef CAS PubMed
. - R. Sridhar, J. R. Venugopal, S. Sundarrajan, R. Ravichandran, B. Ramalingam and S. Ramakrishna, J. Drug Delivery Sci. Technol., 2011, 21, 451–468 CrossRef CAS
. - K. Vinothini and M. Rajan, Mechanism for the Nano-Based Drug Delivery System, Elsevier Inc., 2018 Search PubMed
. - D. Psimadas, P. Georgoulias, V. Valotassiou and G. Loudos, J. Pharm. Sci., 2012, 101, 2271–2280 CrossRef CAS PubMed
. - N. Vij, Expert Opin. Drug Delivery, 2011, 8, 1105–1109 CrossRef CAS PubMed
. - A. P. Subramanian, S. K. Jaganathan, A. Manikandan, K. N. Pandiaraj, N. Gomathi and E. Supriyanto, RSC Adv., 2016, 6, 48294–48314 RSC
. - G. T. Kulkarni, J. Chronother. Drug Delivery, 2011, 2(3), 113–119 Search PubMed
. - X. Shan, C. Liu, F. Li, C. Ouyang, Q. Gao and K. Zheng, Des. Monomers Polym., 2015, 18, 678–689 CrossRef CAS
. - M. Ghezzi, S. Pescina, C. Padula, P. Santi, E. del Favero, L. Cantù and S. Nicoli, J. Controlled Release, 2021, 332, 312–336 CrossRef CAS PubMed
. - T. M. Allen and P. R. Cullis, Adv. Drug Delivery Rev., 2013, 65, 36–48 CrossRef CAS PubMed
. - A. Akbarzadeh, R. Rezaei-Sadabady, S. Davaran, S. W. Joo, N. Zarghami, Y. Hanifehpour, M. Samiei, M. Kouhi and K. Nejati-Koshki, Nanoscale Res. Lett., 2013, 8(1), 1–9 CrossRef PubMed
. - J. Li and D. J. Mooney, Nat. Rev. Mater., 2016, 1(12), 1–12 Search PubMed
. - I. Shahzadi, M. Islam, H. Saeed, A. Haider, A. Shahzadi, J. Haider, N. Ahmed, A. Ul-Hamid, W. Nabgan, M. Ikram and H. A. Rathore, Int. J. Biol. Macromol., 2022, 220, 1277–1286 CrossRef CAS PubMed
. - H. Zare, S. Ahmadi, A. Ghasemi, M. Ghanbari, N. Rabiee, M. Bagherzadeh, M. Karimi, T. J. Webster, M. R. Hamblin and E. Mostafavi, Int. J. Nanomed., 2021, 16, 1681–1706 CrossRef PubMed
. - A. Valizadeh, H. Mikaeili, M. Samiei, S. M. Farkhani, N. Zarghami, M. Kouhi, A. Akbarzadeh and S. Davaran, Nanoscale Res. Lett., 2012, 7, 1–14 CrossRef PubMed
. - S. Nimesh, Gene therapy: Potential applications of nanotechnology, Elsevier, 2013, pp. 13–42 Search PubMed
. - A. Z. Wilczewska, K. Niemirowicz, K. H. Markiewicz and H. Car, Pharmacol. Rep., 2012, 64, 1020–1037 CrossRef CAS PubMed
. - M. Ikram, K. Chaudhary, A. Shahzadi, A. Haider, I. Shahzadi, A. Ul-Hamid, N. Abid, J. Haider, W. Nabgan and A. R. Butt, Mater. Today Nano, 2022, 20, 100271 CrossRef CAS
. - Z. Liu, S. Ramakrishna and X. Liu, APL Bioeng., 2020, 4(3), 030901 CrossRef CAS PubMed
. - S. Ramakrishna, K. Fujihara, W. E. Teo, Z. Ma and T. C. Lim, Electrospinning and nanofibers, World Scientific Publishing Co. Pte. Ltd, Singapore, 2005 Search PubMed
. - R. Sridhar, R. Lakshminarayanan, K. Madhaiyan, V. A. Barathi, K. Hsiu, C. Lim and S. Ramakrishna, Chem. Soc. Rev., 2015, 44, 790–814 RSC
. - N. Goonoo, A. Bhaw-Luximon and D. Jhurry, J. Biomed. Nanotechnol., 2014, 10, 2173–2199 CrossRef CAS PubMed
. - M. Moreno Raja, P. Q. Lim, Y. S. Wong, G. M. Xiong, Y. Zhang, S. Venkatraman and Y. Huang, Polymeric Nanomaterials, Elsevier Inc., 2019 Search PubMed
. - D. Sundaramurthi, U. M. Krishnan and S. Sethuraman, Polym. Rev., 2014, 54, 348–376 CrossRef CAS
. - P. Zahedi, I. Rezaeian, S. O. Ranaei-Siadat, S. H. Jafari and P. Supaphol, Polym. Adv. Technol., 2010, 21, 77–95 CrossRef CAS
. - J. Xue, J. Xie, W. Liu and Y. Xia, Acc. Chem. Res., 2017, 50, 1976–1987 CrossRef CAS PubMed
. - M. Rahimi, A. Jafari, S. J. S. Tabaei, M. Rahimi, S. Taranejoo and M. Ghanimatdan, World J. Cancer Res., 2020, 7, 1–11 Search PubMed
. - X. Mo, B. Sun, T. Wu and D. Li, Electrospun Nanofibers for Tissue Engineering, Elsevier Inc., 2019 Search PubMed
. - R. Goyal, L. K. Macri, H. M. Kaplan and J. Kohn, J. Controlled Release, 2016, 240, 77–92 CrossRef CAS PubMed
. - T. Garg and A. K. Goyal, Expert Opin. Drug Delivery, 2014, 11, 767–789 CrossRef CAS PubMed
. - J. Doshi and D. H. Reneker, Industry Applications Society Annual Meeting, 1993., Conference Record of the 1993 IEEE, 1993, 3, 1698–1703 Search PubMed
. - D. H. Reneker, A. L. Yarin, E. Zussman and H. Xu, Adv. Appl. Mech., 2007, 41, 43–195 Search PubMed
. - D. Li and Y. Xia, Adv. Mater., 2004, 16, 1151–1170 CrossRef CAS
. - Y. Si, X. Tang, J. Yu and B. Ding, Electrospun Nanofibers: Solving Global Issues, Springer US, New York, 2014 Search PubMed
. - J. Li, H. Pan, Q. Ye, C. Shi, X. Zhang and W. Pan, J. Drug Delivery Sci. Technol., 2020, 58, 101726 CrossRef CAS
. - Y. Fu and W. J. Kao, Packag. Technol., 2009, 7, 429–444 Search PubMed
. - F. L. He, X. Deng, Y. Q. Zhou, T. di Zhang, Y. L. Liu, Y. J. Ye and D. C. Yin, Polym. Adv. Technol., 2019, 30, 425–434 CrossRef CAS
. - J. Wu, Z. Zhang, J. Gu, W. Zhou, X. Liang, G. Zhou, C. C. Han, S. Xu and Y. Liu, J. Controlled Release, 2020, 320, 337–346 CrossRef CAS PubMed
. - R. S. Bhattarai, R. D. Bachu, S. H. S. Boddu and S. Bhaduri, Pharmaceutics, 2018, 11(1), 5 CrossRef PubMed
. - M. Vlachou, A. Siamidi and S. Kyriakou, Electrospinning and Electrospraying - Techniques and Applications, 2019, pp. 1–22 Search PubMed
. - S. Wen, Y. Hu, Y. Zhang, S. Huang, Y. Zuo and Y. Min, Mater. Sci. Eng., C, 2019, 100, 514–522 CrossRef CAS PubMed
. - X. Liu, Y. Yang, D. G. Yu, M. J. Zhu, M. Zhao and G. R. Williams, Chem. Eng. J., 2019, 356, 886–894 CrossRef CAS
. - W. Boontung, S. Moonmangmee, A. Chaiyasat and P. Chaiyasat, Adv. Mater. Res., 2012, 506, 303–306 CAS
. - R. Bisotto-de-Oliveira, B. Jorge, I. Roggia, J. Sant'Ana and C. Pereira, J. Res. Updates Polym. Sci., 2014, 3, 40–47 CrossRef
. - P. Coimbra, J. P. Freitas, T. Gonçalves, M. H. Gil and M. Figueiredo, Mater. Sci. Eng., C, 2019, 94, 86–93 CrossRef CAS PubMed
. - H. Lian and Z. Meng, Mater. Sci. Eng., C, 2017, 74, 117–123 CrossRef CAS PubMed
. - K. Cao, Y. Liu, A. A. Olkhov, V. Siracusa and A. L. Iordanskii, Drug Delivery Transl. Res., 2018, 8, 291–302 CrossRef CAS PubMed
. - M. L. Muerza-Cascante, D. Haylock, D. W. Hutmacher and P. D. Dalton, Tissue Eng., Part B, 2015, 21, 187–202 CrossRef CAS PubMed
. - T. Vigh, T. Horváthová, A. Balogh, P. L. Sóti, G. Drávavölgyi, Z. K. Nagy and G. Marosi, Eur. J. Pharm. Sci., 2013, 49, 595–602 CrossRef CAS PubMed
. - H. Lian and Z. Meng, Bioact. Mater., 2017, 2, 96–100 CrossRef PubMed
. - N. M. Neves, R. Campos, A. Pedro, J. Cunha, F. Macedo and R. L. Reis, Int. J. Nanomed., 2007, 2, 433–448 CAS
. - A. Laha, C. S. Sharma and S. Majumdar, Mater. Sci. Eng., C, 2017, 76, 782–786 CrossRef CAS PubMed
. - P. Wang, Y. Li, C. Zhang, F. Feng and H. Zhang, Food Chem., 2020, 308, 125599 CrossRef CAS PubMed
. - D. Li, Y. Wang and Y. Xia, Adv. Mater., 2004, 16, 361–366 CrossRef CAS
. - D. Li, G. Ouyang, J. T. McCann and Y. Xia, Nano Lett., 2005, 5, 913–916 CrossRef CAS PubMed
. - M. M. Saadatmand, M. E. Yazdanshenas, R. Khajavi, F. Mighani and T. Toliyat, Int. J. Nano Dimens., 2019, 10, 78–88 CAS
. - T. Li, L. Liu, L. Wang and X. Ding, J. Biomater. Sci., Polym. Ed., 2019, 30, 1454–1469 CrossRef CAS PubMed
. - T. Eren Boncu, N. Ozdemir and A. Uskudar Guclu, Drug Dev. Ind. Pharm., 2020, 46, 109–121 CrossRef CAS PubMed
. - T. Li, L. Wang, Y. Huang, B. Xin and S. Liu, J. Biomater. Sci., Polym. Ed., 2020, 31, 1223–1236 CrossRef CAS PubMed
. - M. Stack, D. Parikh, H. Wang, L. Wang, M. Xu, J. Zou, J. Cheng and H. Wang, Electrospun Nanofibers for Drug Delivery, Elsevier Inc., 2019 Search PubMed
. - S. Smith, K. Goodge, M. Delaney, A. Struzyk, N. Tansey and M. Frey, Nanomaterials, 2020, 10, 1–39 CrossRef CAS PubMed
. - X. Chen, C. Xu and H. He, Biochem. Biophys. Res. Commun., 2019, 516, 1085–1089 CrossRef CAS PubMed
. - M. de Melo Brites, A. A. Cerón, S. M. Costa, R. C. Oliveira, H. G. Ferraz, L. H. Catalani and S. A. Costa, Enzyme Microb. Technol., 2020, 132, 109384 CrossRef CAS PubMed
. - Y. Pilehvar-soltanahmadi, A. Akbarzadeh, N. Moazzez-lalaklo and N. Zarghami, Artif. Cells, Nanomed., Biotechnol., 2016, 44(6), 1350–1364 CrossRef CAS PubMed
. - A. P. Kishan, R. M. Nezarati, C. M. Radzicki, A. L. Renfro, J. L. Robinson, M. E. Whitely and E. M. Cosgriff-Hernandez, J. Mater. Chem. B, 2015, 3, 7930–7938 RSC
. - C. E. Campiglio, N. C. Negrini, S. Farè and L. Draghi, Materials, 2019, 12(15), 2476 CrossRef CAS PubMed
. - M. M. Abdul Hameed, S. A. P. Mohamed Khan, B. M. Thamer, A. Al-Enizi, A. Aldalbahi, H. El-Hamshary and M. H. El-Newehy, J. Macromol. Sci., Part A: Pure Appl. Chem., 2020, 1–15 Search PubMed
. - L. A. Bosworth, L. A. Turner and S. H. Cartmell, Nanomedicine, 2013, 9, 322–335 CrossRef CAS PubMed
. - Y. Yang, W. Li, D. G. Yu, G. Wang, G. R. Williams and Z. Zhang, Carbohydr. Polym., 2019, 203, 228–237 CrossRef CAS PubMed
. - T. Hai, X. Wan, D. G. Yu, K. Wang, Y. Yang and Z. P. Liu, Mater. Des., 2019, 162, 70–79 CrossRef CAS
. - J. G. Edmans, K. H. Clitherow, C. Murdoch, P. v. Hatton, S. G. Spain and H. E. Colley, Pharmaceutics, 2020, 12, 1–21 CrossRef PubMed
. - E. Sipos, N. Kósa, A. Kazsoki, Z. I. Szabó and R. Zelkó, Pharmaceutics, 2019, 11, 1–11 CrossRef PubMed
. - S. Hua, Front. Pharmacol., 2020, 11, 1–22 CrossRef PubMed
. - M. Rostami, M. Ghorbani, M. Aman mohammadi, M. Delavar, M. Tabibiazar and S. Ramezani, Int. J. Biol. Macromol., 2019, 135, 698–705 CrossRef CAS PubMed
. - S. Tort, D. Han and A. J. Steckl, Int. J. Pharm., 2020, 579, 119164 CrossRef CAS PubMed
. - A. Laha, S. Majumdar and C. S. Sharma, MRS Adv., 2016, 1, 2107–2113 CrossRef CAS
. - M. Gaydhane, P. Choubey, C. S. Sharma and S. Majumdar, Mater. Today Commun., 2020, 24, 100953 CrossRef CAS
. - M. Vlachou, S. Kikionis, A. Siamidi, S. Kyriakou, A. Tsotinis, E. Ioannou and V. Roussis, Pharmaceutics, 2019, 11, 1–13 CrossRef PubMed
. - A. Laha, M. K. Gaydhane, C. S. Sharma and S. Majumdar, Nano-Struct. Nano-Objects, 2019, 19, 100367 CrossRef CAS
. - Y. Zhou, Q. Zhao and M. Wang, MRS Commun., 2019, 9, 1098–1104 CrossRef CAS
. - H. Ishii, H. Todo, A. Terao, T. Hasegawa, M. Akimoto, K. Oshima and K. Sugibayashi, Drug Dev. Ind. Pharm., 2009, 35, 1356–1363 CrossRef CAS PubMed
. - M. Rahmani, S. Arbabi Bidgoli and S. M. Rezayat, Nanomed. J., 2017, 4, 61–70 CAS
. - S. Sa’adon, S. I. Abd Razak, A. E. Ismail and K. Fakhruddin, Procedia Comput. Sci., 2019, 158, 436–442 CrossRef
. - Z. Cui, Z. Zheng, L. Lin, J. Si, Q. Wang, X. Peng and W. Chen, Adv. Polym. Technol., 2018, 37, 1917–1928 CrossRef CAS
. - G. el Fawal, H. Hong, X. Song, J. Wu, M. Sun, L. Zhang, C. He, X. Mo and H. Wang, Appl. Biochem. Biotechnol., 2020, 191, 1624–1637 CrossRef CAS PubMed
. - A. Gencturk, E. Kahraman, S. Güngör, G. Özhan, Y. Özsoy and A. S. Sarac, Artif. Cells, Nanomed., Biotechnol., 2017, 45, 655–664 CrossRef CAS PubMed
. - H. Iqbal, B. A. Khan, Z. U. Khan, A. Razzaq, N. U. Khan, B. Menaa and F. Menaa, Int. J. Biol. Macromol., 2020, 144, 921–931 CrossRef CAS PubMed
. - M. K. Gaydhane, J. S. Kanuganti and C. S. Sharma, J. Mater. Res., 2020, 600–609 CrossRef CAS
. - S. S. Said, A. K. Aloufy, O. M. El-Halfawy, N. A. Boraei and L. K. El-Khordagui, Eur. J. Pharm. Biopharm., 2011, 79, 108–118 CrossRef CAS PubMed
. - P. Zahedi, Z. Karami, I. Rezaeian, S. Jafari, P. Mahdaviani, A. H. Abdolghaffari and M. Abdollahi, J. Appl. Polym. Sci., 2011, 124, 4174–4183 CrossRef
. - P. Sasmal and P. Datta, J. Drug Delivery Sci. Technol., 2019, 52, 559–567 CrossRef CAS
. - J. S. Ribeiro, A. Daghrery, N. Dubey, C. Li, L. Mei, J. C. Fenno, A. Schwendeman, Z. Aytac and M. C. Bottino, Biomacromolecules, 2020, 21, 3945–3956 CrossRef CAS PubMed
. - D. Kai, M. P. Prabhakaran, B. Stahl, M. Eblenkamp, E. Wintermantel and S. Ramakrishna, Nanotechnology, 2012, 23(9), 095705 CrossRef PubMed
. - E. L. Bakota, Y. Wang, F. R. Danesh and J. D. Hartgerink, Biomacromolecules, 2011, 12, 1651–1657 CrossRef CAS PubMed
. - H. Wu, S. Liu, L. Xiao, X. Dong, Q. Lu and D. L. Kaplan, ACS Appl. Mater. Interfaces, 2016, 8, 17118–17126 CrossRef CAS PubMed
. - W. Liu, J. Zhan, Y. Su, T. Wu, S. Ramakrishna, S. Liao and X. Mo, J. Biomater. Sci., Polym. Ed., 2014, 25, 168–180 CrossRef CAS PubMed
. - Q. Fu, C. Duan, Z. Yan, Y. Li, Y. Si, L. Liu, J. Yu and B. Ding, Macromol. Rapid Commun., 2018, 39, 1–19 CrossRef PubMed
. - X. Li, B. Cho, R. Martin, M. Seu, C. Zhang, Z. Zhou, J. S. Choi, X. Jiang, L. Chen, G. Walia, J. Yan, M. Callanan, H. Liu, K. Colbert, J. Morrissette-McAlmon, W. Grayson, S. Reddy, J. M. Sacks and H. Q. Mao, Sci. Transl. Med., 2019, 11, 1–12 CAS
. - J. Sun, K. Bubel, F. Chen, T. Kissel, S. Agarwal and A. Greiner, Macromol. Rapid Commun., 2010, 31, 2077–2083 CrossRef CAS PubMed
. - R. Jain, S. Shetty and K. S. Yadav, J. Drug Delivery Sci. Technol., 2020, 57, 101604 CrossRef CAS
. - Anonymous, Benign and green electrospinning, http://electrospintech.com/greenelectrospinning.html, accessed 16 November 2020.
- R. Krishnan, S. Sundarrajan and S. Ramakrishna, Macromol. Mater. Eng., 2013, 298(10), 1034–1058 CrossRef CAS
. - S. Li, L. Kong and G. R. Ziegler, Carbohydr. Polym., 2021, 258, 116933 CrossRef CAS PubMed
. - D. Gao, Y. Mi and Z. Gao, Mater. Lett., 2020, 276, 128237 CrossRef CAS
. - D. Miele, L. Catenacci, S. Rossi, G. Sandri, M. Sorrenti, A. Terzi, C. Giannini, F. Riva, F. Ferrari, C. Caramella and M. C. Bonferoni, Materials, 2020, 13, 1–24 CrossRef PubMed
. - G. Thakur, F. C. Rodrigues and K. Singh, Adv. Exp. Med. Biol., 2018, 1078, 213–231 CrossRef CAS PubMed
. - G. Thakur, A. Mitra, D. Rousseau, A. Basak, S. Sarkar and K. Pal, J. Mater. Sci.: Mater. Med., 2011, 22, 115–123 CrossRef CAS PubMed
. - U. Bhutani, A. Laha, K. Mitra and S. Majumdar, Mater. Lett., 2016, 164, 76–79 CrossRef CAS
. - U. Bhutani and S. Majumdar, Mater. Discovery, 2017, 8, 1–8 CrossRef
. - A. Laha, U. Bhutani, K. Mitra and S. Majumdar, Mater. Manuf. Processes, 2016, 31, 223–230 CrossRef CAS
. - U. Bhutani, A. Ronghe and S. Majumdar, ACS Appl. Bio Mater., 2018, 1, 1244–1253 CrossRef CAS PubMed
. - N. Letha, J. Joseph, G. Sundar, A. U. Pillai, A. John and A. Abraham, J. Bioact. Compat. Polym., 2020, 35, 451–466 CrossRef CAS
. - S. Suganya, T. S. Ram, B. S. Lakshmi and V. R. Giridev, J. Appl. Polym. Sci., 2011, 121, 2893–2899 CrossRef CAS
. - S. Suganya, J. Venugopal, S. Ramakrishna, B. S. Lakshmi and V. R. Dev, J. Biomater. Tissue Eng., 2014, 4, 9–19 CrossRef CAS
. - S. H. Ansari, F. Islam and M. Sameem, J. Adv. Pharm. Technol. Res., 2012, 3, 142–146 CrossRef CAS PubMed
. - V. Andreu, G. Mendoza, M. Arruebo and S. Irusta, Materials, 2015, 8, 5154–5193 CrossRef PubMed
. - S. Rasouli, M. Montazeri, S. Mashayekhi, S. Sadeghi-Soureh, M. Dadashpour, H. Mousazadeh, A. Nobakht, N. Zarghami and Y. Pilehvar-Soltanahmadi, J. Drug Delivery Sci. Technol., 2020, 55, 101402 CrossRef CAS
. - X. Feng, J. Li, X. Zhang, T. Liu, J. Ding and X. Chen, J. Controlled Release, 2019, 302, 19–41 CrossRef CAS PubMed
. - M. Spitzer, N. Robbins and G. D. Wright, Virulence, 2017, 8, 169–185 CrossRef CAS PubMed
. - R. Rasouli, A. Barhoum, M. Bechelany and A. Dufresne, Macromol. Biosci., 2019, 19, 1–27 CrossRef PubMed
. - H. Li, J. Zhu, S. Chen, L. Jia and Y. Ma, RSC Adv., 2017, 7, 56550–56558 RSC
. - B. Gidwani and A. Vyas, Colloids Surf., B, 2014, 114, 130–137 CrossRef CAS PubMed
. - A. Celebioglu, F. Kayaci-Senirmak, S. Ipek, E. Durgun and T. Uyar, Food Funct., 2016, 7, 3141–3153 RSC
. - Z. I. Yildiz, A. Celebioglu and T. Uyar, Int. J. Pharm., 2017, 531, 550–558 CrossRef CAS PubMed
. - A. Celebioglu and T. Uyar, Mol. Pharm., 2019, 16, 4387–4398 CrossRef CAS PubMed
.
|
This journal is © The Royal Society of Chemistry 2023 |