DOI:
10.1039/D3QO00408B
(Review Article)
Org. Chem. Front., 2023,
10, 2830-2848
Recent advances in the electrochemical generation of 1,3-dicarbonyl radicals from C–H bonds
Received
20th March 2023
, Accepted 24th April 2023
First published on 25th April 2023
Abstract
Electrochemistry, which employs electrons as reagents and avoids the use of traditional redox reagents, becomes a powerful tool in oxidative dehydrogenation cross-coupling reactions. 1,3-Dicarbonyl derivatives are common and easily available synthetic blocks and are widely used for the construction of active molecules in organic synthesis. 1,3-Dicarbonyl radicals can be generated by direct anodic oxidation or indirect anodic oxidation with the assistance of a redox reagent and can undergo addition to arenes, double bonds or triple bonds to build multifarious organic molecules. In this review, we succinctly summarize the recent advances in the electrochemical generation of 1,3-dicarbonyl radicals from C–H-based 1,3-dicarbonyl derivatives and focus on the mechanistic insights and synthetic applications of these methods and transformations.
1. Introduction
Organic electrosynthesis, as a green synthesis technology, attracts increasing attention from researchers.1 It uses electrons as redox reagents, which eliminates the need for traditional oxidizing and reducing reagents. Various highly active intermediates, such as radicals, cations and anions, can be efficiently produced by electrooxidation at the anode or electroreduction at the cathode.2 Organic electrochemistry can be combined with hot synthetic strategies, such as metal catalysis,3 photochemistry4 and asymmetric catalysis,5 to achieve challenging chemical transformations.
1,3-Dicarbonyl derivatives are a kind of cheap and easily available synthetic unit and are frequently used in organic synthetic chemistry to build bioactive molecules.6 The reductive dehalogenation of prefunctionalized C–X bonds has become an efficient method for generating carbon-centered radicals.7 Direct transformations of simple and easily available C–H bonds are more economical and competitive in organic synthesis.8 1,3-Dicarbonyl radicals can be formed via the single electron oxidation of C–H bonds using stoichiometric metal reagents or oxidants and have been studied extensively.9 In addition, photocatalysis provides an alternative and efficient route to produce 1,3-dicarbonyl radicals from C–H bonds, but the use of oxidants or metal reagents in oxidation-type reactions is unavoidable.10
1,3-Dicarbonyl derivatives, as diverse synthons, have been widely explored in a range of electrochemical transformations.11 Electrochemical self-coupling reactions of 1,3-dicarbonyl derivatives have been discovered for decades.12 Until recently, the electrochemical generation and application of 1,3-dicarbonyl radicals have again attracted the attention of researchers. 1,3-Dicarbonyl radicals can be generated through electrochemical oxidation by direct or indirect electrolysis13 with a mediator, which allows the reaction to proceed under mild conditions at lower potentials. The protons are reduced at the cathode to release hydrogen. These electrochemical reactions are not dependent on sacrificial chemical oxidants, simplifying the experimental conditions and avoiding the generation of chemical waste. 1,3-Dicarbonyl radicals produced by the anodic oxidation of C–H bonds can react with alkenes, alkynes and aromatic-ring systems to furnish a variety of organic structures, in which the 1,3-dicarbonyl moiety in the products can undergo various transformation reactions.
Recently, many breakthroughs have been made in the electrochemical generation of 1,3-dicarbonyl radicals. Herein, we summarize recent advances of the electrochemical generation of 1,3-dicarbonyl radicals and mainly focus on the reaction design, reaction mechanism and developmental prospects (Fig. 1). This review is divided into four sections according to the type of reaction substrates as follows: (i) the addition of 1,3-dicarbonyl radicals to arenes, (ii) the addition of 1,3-dicarbonyl radicals to alkenes, (iii) the addition of 1,3-dicarbonyl radicals to alkynes, and (iv) the radical–radical coupling of 1,3-dicarbonyl radicals.
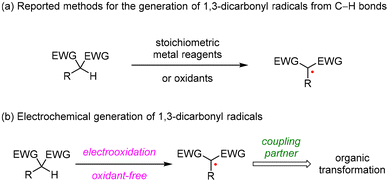 |
| Fig. 1 Generation of 1,3-dicarbonyl radicals from C–H bonds. | |
2. The addition of 1,3-dicarbonyl radicals to arenes
2.1 Intramolecular radical addition cyclization
2-Oxindoles are attractive isatin derivatives that are being extensively explored because they are often found in pharmaceuticals and bioactive natural products.14 The introduction of fluorine-containing groups can effectively improve the physical, chemical and biological properties of organic compounds.15 During the past few years, 3-fluorooxindoles have usually been synthesized through the derivatization of oxindoles, Pd-catalyzed cross-coupling of arylbromides or fluoroarylation of diazoacetamides, in which these reactions often depend on pre-functionalized precursors or violent reaction conditions.16 In 2017, Xu and co-workers reported the Cp2Fe-catalyzed electrochemical intramolecular cyclization of malonate amides for the synthesis of 3-fluorooxindoles through dehydrogenation cross-coupling (Scheme 1).17 This approach is implemented by employing ferrocene (Cp2Fe)18 as a redox catalyst with LiCp as a base in MeOH/THF, which obviates the use of sacrificial oxidizing reagents. A number of functionalized 3-fluorooxindoles are synthesized in a simple undivided cell with good functional group tolerance. The reaction begins with the anodic oxidation of Cp2Fe to form Cp2Fe+ and the cathodic reduction of MeOH to give MeO− and H2. The carbon anion intermediate 1-8, produced by the deprotonation of 1-7 with the assistance of MeO− or LiCp, undergoes a single electron transfer with Cp2Fe+ to furnish a 1,3-dicarbonyl radical intermediate 1-9. Subsequently, 1-9 undergoes radical addition cyclization and rearomatization to provide the final oxindole 1-3.
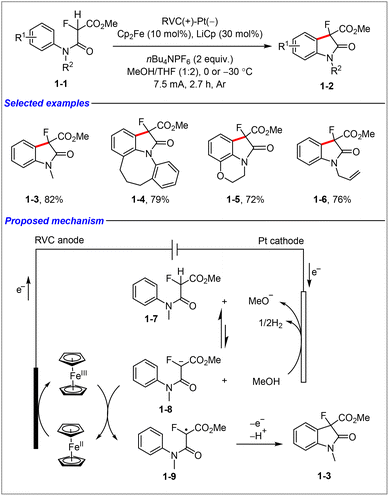 |
| Scheme 1 Electrochemical cyclization of fluorinated malonate amides. | |
On the basis of the above findings, Cp2Fe-catalyzed electrochemical dehydrogenative cyclization of 1,3-dicarbonyl derivatives through releasing hydrogen has been developed by Xu and co-workers (Scheme 2).19 3-Alkyl-substituted oxindoles and 3,4-dihydro-1H-quinolin-2-ones are constructed efficiently under mild electrochemical conditions. The dehydrogenative cross-coupling of a malonic ester and a pyrrole ring is fulfilled to afford the 2,3-dihydro-1H pyrrolizine 2-7, which undergoes saponification and decarboxylation to give the anti-inflammatory drug ketorolac.20
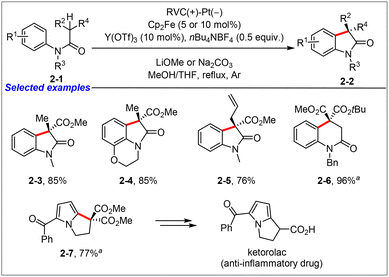 |
| Scheme 2 Electrochemical dehydrogenative cyclization of alkyl-substituted 1,3-dicarbonyl derivatives (a reaction was carried out without Y(OTf)3). | |
Direct electrochemical oxidation of 1,3-dicarbonyl derivatives was described by Mei and co-workers in 2021 (Scheme 3).21 The electrochemical intramolecular cycloaromatization of 1-biphenyl-2-ylethanone bearing an electron-withdrawing group is achieved in the presence of K2CO3 in a methanol solution. The electrochemical C–H/C–H cross-coupling avoids the use of additional catalysts or sacrificial oxidants and provides a simple and efficient way to synthesize a variety of 10-phenanthrenol derivatives. Based on experimental results, a radical mechanism is proposed. At the cathode, methanol is reduced to give a methoxyl anion and hydrogen. Ester-substituted 1-biphenyl-2-ylethanone 3-7 reacts with the methoxyl anion via deprotonation to furnish an anion intermediate 3-8. The latter is directly oxidized at the anode to form a carbon-centered radical intermediate 3-9, which undergoes radical cyclization and rearomatization to afford 10-phenanthrenol 3-10.
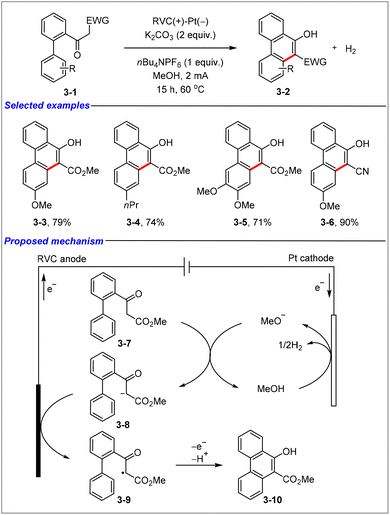 |
| Scheme 3 Direct electrochemical cyclization of 1-biphenyl-2-ylethanones. | |
2.2 Intermolecular radical addition
Electrochemically facilitated multi-component intermolecular addition reactions are also attracting attention. The NaI-promoted electrochemical cross-coupling of indoles and 1,3-dicarbonyl derivatives was reported by Park's group in 2020 (Scheme 4).22 Highly functionalized dihydropyrano[4,3-b]indoles are constructed at a constant voltage of 3.0 V through oxidative [3 + 3] cycloaddition followed by tandem 6π-electrocyclization. After a series of mechanism experiments and density functional theory (DFT) calculations, the authors proposed the following mechanism.
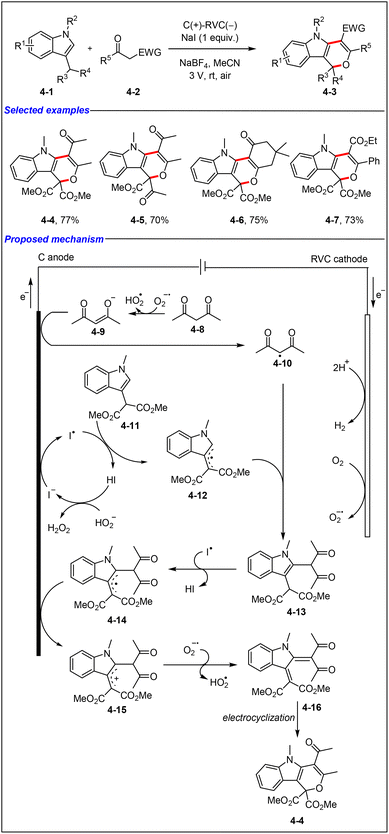 |
| Scheme 4 Electrochemical cross-coupling of indoles and 1,3-dicarbonyl derivatives. | |
Firstly, a superoxide generated by the cathodic reduction of O2 reacts with 1,3-diketone 4-8 to give enolate 4-9, following which 4-9 is oxidized at the anode to furnish diketone radical 4-10. Meanwhile, an iodine radical generated by the anodic oxidation of the iodide abstracts a hydrogen atom from 4-11 to afford carbon-centered radical 4-12. Then radical–radical cross-coupling of 4-10 and 4-12 furnishes intermediate 4-13, which reacts with the iodine radical via a HAT process again to produce radical intermediate 4-14. The anodic oxidation and deprotonation of 4-14 furnishes oxatriene 4-16, which undergoes 6π-electrocyclization to form the final product 4-4.
In 2022, Sun's group reported the electrochemical [3 + 2] cycloaddition of anilines and 1,3-dicarbonyl derivatives (Scheme 5).23 The reaction is directly executed with 30 mol% TfOH in a solution of nBu4NBF4 in HFIP without the need for metal catalysts or external oxidants, providing a powerful method for the construction of a broad range of multi-substituted indoles. The success of the reaction depends on the anodic oxidation of 5-7 and 5-9 at the same time to form nitrogen-centered radical 5-8 and 1,3-dicarbonyl radical 5-10, in which 5-8 resonates to give carbon-centered radical 5-11. Subsequently, radical–radical cross-coupling of 5-10 and 5-11 produces 5-12. The intermediate 5-12 undergoes tautomerization and intramolecular condensation to afford the final product 5-14. Meanwhile, the cathode reduces protons to H2.
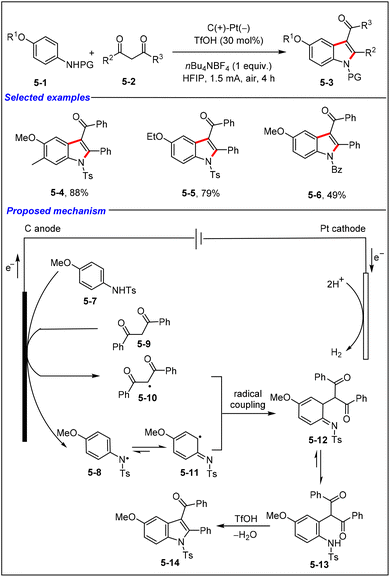 |
| Scheme 5 Electrochemical [3 + 2] cycloaddition of anilines and 1,3-dicarbonyl derivatives. | |
3. Addition of 1,3-dicarbonyl radicals to alkenes
Olefins are one of the most common organic molecules and are widely used in organic synthesis because of their abundant sources, easy availability and diverse reactivity. The difunctionalization of olefins is an effective method for rapidly increasing the complexity of molecules to obtain abundant organic skeletons. 1,3-Dicarbonyl radicals generated by electrochemical oxidation can react with alkenes to build complex molecules under mild conditions. The electrochemical cycloaddition of olefins, 1,3-diketones and molecular oxygen for the synthesis of cyclic peroxides was reported by Yoshida and co-workers in 1989.24 In recent years, more breakthrough research has been made in this field and various new reactions and mechanisms have been investigated.
3.1 Intramolecular radical addition cyclization
In 2021, Xu and co-workers developed Co-catalyzed electrochemical intramolecular allylic C(sp3)–H alkylation of 1,3-dicarbonyl derivatives (Scheme 6).25 This protocol is compatible with a series of functional groups and alkenes, providing a straightforward and atom-economical method to construct high-value alkene-bearing cyclic structures under chemical oxidant-free conditions. Decagram-scale electrosynthesis is conducted to further demonstrate the practicality of this approach. Compared to Cu-promoted allylic C–H functionalization, Co-catalyzed electrochemical conditions are more efficient for the oxidation of sterically hindered 2° carbon-centered radical intermediates and have better selectivity in the H-elimination process. This Co-catalyzed electrochemical strategy provides a new direction for radical transformations of active methylene compounds.
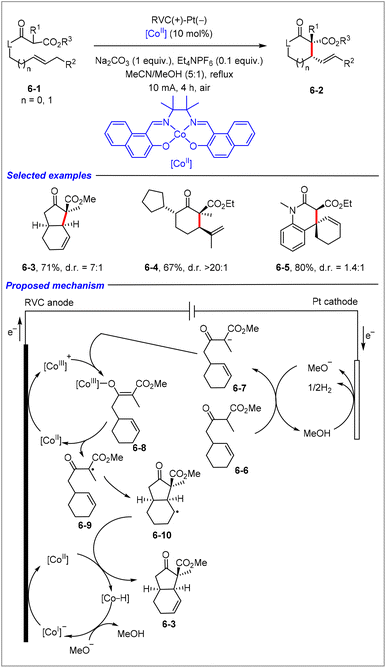 |
| Scheme 6 Electrochemical intramolecular allylic C(sp3)–H alkylation. | |
A possible mechanism for the Co-catalyzed electrochemical allylic C–H alkylation is proposed. The cathodic reduction of MeOH generates MeO− and H2. The deprotonation of 6-6 with MeO− gives carbanion 6-7, which reacts with a [CoIII]+ species generated by the anodic oxidation of the Co catalyst [CoII] to form 1,3-dicarbonyl radical 6-9. The intramolecular radical cyclization of 6-9 affords 6-10, which reacts with [CoII] via H-transfer or β-H elimination to furnish the final product 6-3 and a [Co–H] intermediate. The Co catalyst [CoII] is regenerated by the anodic oxidation of a [CoI]− species formed by the deprotonation of the [Co–H] species with the assistance of MeO−. The success of the reaction depends on the dual role of MeO−, which can effectively promote the oxidation of 6-6 and the regeneration of the Co catalyst [CoII].
Subsequently, organocatalyzed electrochemical intramolecular cyclopropanation of alkenes with active methylene compounds has also been developed (Scheme 7).26 This reaction is performed with 20 mol% of N-arylphenothiazine 7-2 as an organocatalyst in a solution of Et4NBF4 in CF3CH2OH (TFE) and numerous cyclopropane-fused ring systems are prepared with excellent compatibility and scalability under metal-catalyst-free and chemical oxidant-free conditions. Mechanistic studies show that the electrolysis reaction involves a radical–polar crossover process. At the outset, the phenothiazine-based catalyst 7-2 is oxidized at the anode to produce radical cation 7-8, which reacts with substrate 7-9via single-electron transfer to furnish carbon-centered radical intermediate 7-10 with the assistance of CF3CH2O− produced by cathodic reduction and regenerate catalyst 7-2. Then, the intermolecular radical–radical cross-coupling of alkyl radical 7-11 produced by the 6-exo-trig cyclization of 7-10 and 7-8 forms sulfonium 7-12. In the end, a Corey–Chaykovsky-type27 nucleophilic substitution of 7-12 furnishes the cyclopropanation product 7-13 and regenerates 7-2. At the cathode, CF3CH2OH is reduced to produce CF3CH2O− and hydrogen.
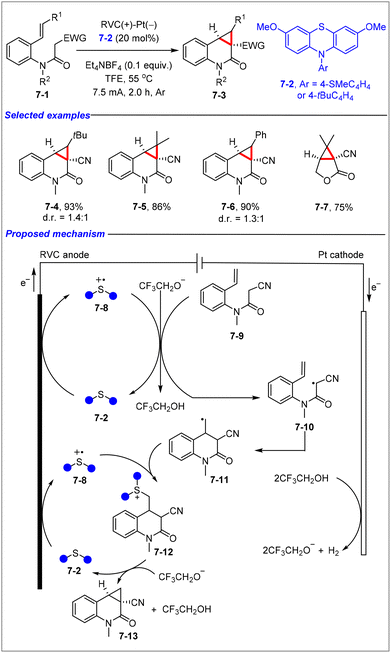 |
| Scheme 7 Electrochemical cyclopropanation of active methylene compounds. | |
The electrochemical oxidative cyclization of tetramethyl butane-1,1,4,4-tetracarboxylate 8-1 using NaI as both a substoichiometric mediator and an electrolyte was achieved by Baran and co-workers in 2020 (Scheme 8).28 A 120-gram-scale reaction is performed at a constant current of 2.5 A with 85% yield in a continuous flow system reactor containing a modular multiplate/frame cell. The formed cyclobutane 8-2 undergoes reduction and nitration to afford cyclobutane-1,1,2,2-tetrayltetrakis(methylene) tetranitrate 8-3, a promising candidate for a melt-castable energetic material. This work distinctly demonstrates the potential of electrochemical reactions in synthetic applications. The authors proposed that this process may involve iodine-promoted nucleophilic cyclization or the addition of the 1,3-dicarbonyl radical to an enolate. The mechanism involving a radical pathway is shown in Scheme 8.
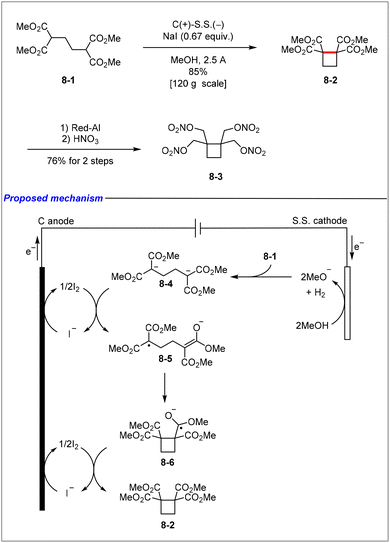 |
| Scheme 8 Electrochemical oxidative cyclization of tetramethyl butane-1,1,4,4-tetracarboxylate (S.S. = stainless steel). | |
3.2 Intermolecular radical addition
The electrochemical intermolecular radical addition of 1,3-dicarbonyl derivatives to alkenes is an effective route to achieve various conversions of alkenes. Back in 2018, the electrochemical dehydrogenative annulation of N-allyl amides with 1,3-dicarbonyl derivatives for the synthesis of highly functionalized pyrrolidines and tetrahydropyridines through a [4 + 1] or [4 + 2] annulation was achieved by Xu and co-workers (Scheme 9).29 This strategy used an unexplored phenothiazine 9-3 as a redox catalyst for the first time in organic electrosynthesis, in which 9-3 (Ep/2 = 0.52 V vs. SCE) was oxidized at the anode to obtain radical cation 9-3˙+. At the same time, H2O was reduced at the cathode to give H2 and HO−, following which HO− reacted with substrates 9-2 (Ep/2 > 2.0 V vs. SCE, R4 = OMe) to furnish the carbon anion intermediates 9-9 (Ep/2 = 0.31 V vs. SCE, R4 = OMe). The effective single-electron transfer from 9-9 to 9-3˙+ produces 1,3-dicarbonyl radicals 9-10 and regenerates catalyst 9-3. Subsequently, 9-10 add to the alkenyl moiety of N-allyl amides 9-1 and these are oxidized by 9-3˙+ to form oxazole cations 9-12. The latter undergo a nucleophilic addition with HO− or H2O followed by cleavage of the C–N bond to furnish intermediates 9-14. Finally, the secondary amines 9-14 undergo dehydrogenative cyclization through C–H/N–H cross-coupling to afford pyrrolidines 9-4 or dehydration annulation to furnish tetrahydropyridines 9-5. It is worth noting that HO− produced by the cathodic reduction of H2O can participate in the formation of 1,3-dicarbonyl radicals 9-10 and the formal migration of an acyl group without the use of extra base.
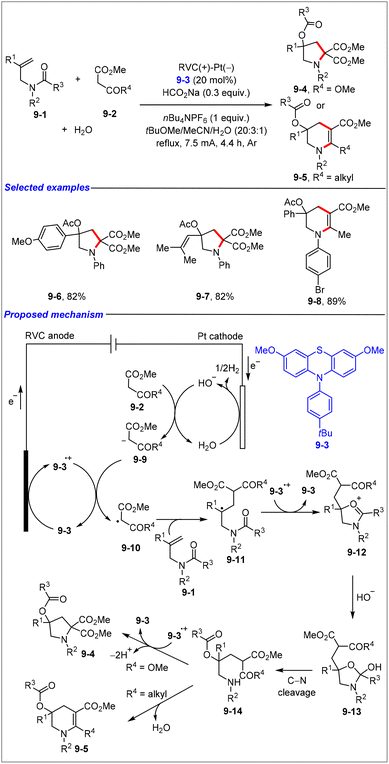 |
| Scheme 9 Electrochemical [4 + 1] or [4 + 2] annulation of N-allyl amides with 1,3-dicarbonyl derivatives. | |
An electrochemical [3 + 2] annulation of alkenes with 1,3-dicarbonyl derivatives was explored by Lei and co-workers in 2019 (Scheme 10).30 This work provides a green and efficient approach for the synthesis of dihydrofurans without metal catalysts and chemical oxidants. The anodic oxidation of acetylacetone anion 10-8 produced by the deprotonation of acetylacetone 10-7 by a base gives 1,3-dicarbonyl radical 10-9. The latter adds to styrene to form benzylic radical 10-10, following a single-electron oxidation at the anode to generate benzylic cation 10-11, which is stabilized by the HFIP cluster to afford the intermediate 10-12. The tautomerization and intramolecular cyclization of 10-12 affords the final annulation product 10-4.
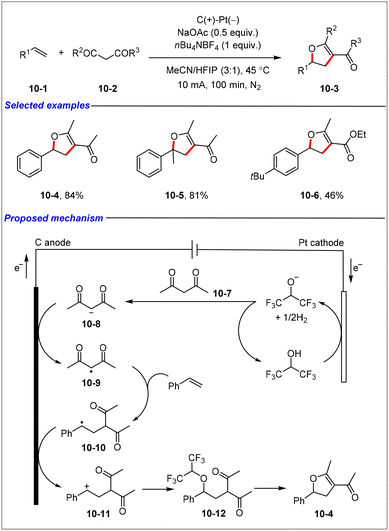 |
| Scheme 10 Electrochemical [3 + 2] annulation of alkenes with 1,3-dicarbonyl derivatives. | |
The manganese-mediated generation of carbon-centered radicals is a direct and powerful strategy for C–C bond formation via the radical addition or cyclization of carbonyl derivatives.31 In 2019, Mn(OTf)2-catalyzed electrochemical chloroalkylation of alkenes using malononitriles or cyanoacetates as carbon-centered radical precursors and NaCl as a chlorine source was described by Lin and co-workers.32 This protocol involves an anodically coupled electrolysis process that achieves the parallel oxidation of [MnII] and [MnII]–Cl at the anode for the generation of [MnIII] and latent chlorine radical [MnIII–Cl]. A similar mechanism process involving anodically coupled electrolysis was described in their previous work on the difunctionalization of alkenes.33 The Mn-catalyzed electrochemical oxyalkylation cyclization of alkenes with acetylacetone and acetylacetates as radical precursors is carried out in these systems, and some 1,2-dihydrofuran-type products are formed (Scheme 11). These products can also be obtained in the absence of NaCl. The oxidation of 11-2 by [MnIII] generated by the anodic oxidation of [MnII] forms radicals 11-7, which add to the alkenes to furnish radicals 11-8. The latter are oxidized by [MnIII] and undergo intramolecular nucleophilic cyclization to form oxyalkylation cyclization products 11-3 and regenerate [MnII]. At the cathode, AcOH is reduced to produce AcO− and hydrogen. The acetate serves as both a ligand to Mn and a proton acceptor to accelerate the oxidation of 11-2. Moreover, the dichlorination products are often afforded as the main products under these conditions when using malonates as radical sources. These findings suggest that the oxidation of radicals 11-8 to cations 11-9 is promoted by the formation of 11-3 through the subsequent nucleophilic cyclization.
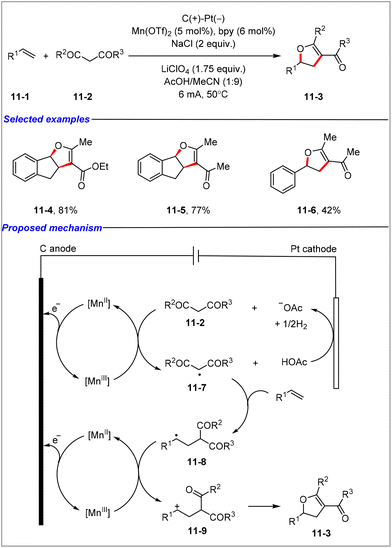 |
| Scheme 11 Mn-catalyzed electrochemical oxyalkylation cyclization of alkenes with 1,3-dicarbonyl derivatives. | |
The direct dehydrogenative cross-coupling of allylic C(sp3)–H bonds and C(sp3)–H bonds through hydrogen evolution is an efficient and robust strategy for synthesizing various structures containing double bonds. In this regard, noble metal-catalyzed and chemical oxidants-assisted allylic C(sp3)–H alkylations have been widely explored in organic synthesis in recent decades.34 The synergy of metal catalysis and electrochemistry has emerged as a very versatile and powerful synthetic tool in organic synthesis, which can achieve a series of challenging chemical transformations including cross-coupling reactions, difunctionalization of alkenes, C–H functionalization and carboxylation without stoichiometric redox reagents.35
By merging cobalt catalysis and electrochemistry, Xu and co-workers reported electrocatalytic allylic C–H alkylation via intermolecular C(sp3)–H/C(sp3)–H cross-coupling with the release of H2 (Scheme 12).36 This reaction is carried out by utilizing a cobalt salen complex as the molecular catalyst and avoids the need for sacrificial oxidants. The application of the cobalt catalyst allows the electrochemical reaction to proceed under more mild conditions, demonstrating excellent functional group compatibility and substrate applicability. This method is further highlighted by its decagram-scale synthesis and various late-stage transformations. The allylic C–H alkylation starts with the oxidation of the [CoII] catalyst (Ep/2 = 0.10 V vs. SCE) at the anode to afford a [CoIII] complex. The latter reacts with 1,3-dicarbonyl derivative 12-8 with the assistance of CO32−via inner sphere electron transfer to give 1,3-dicarbonyl radical 12-10 and regenerates the [CoII] catalyst. The carbon-centered radical 12-10 adds to intermolecular alkene 12-11 to produce a new carbon-centered radical 12-12, from which is selectively abstracted a hydrogen atom at the γ-position rather than at the α-position with its greater steric hindrance to form the final allylic C–H alkylation product 12-4 and the [Co–H] species. The [CoII] catalyst is regenerated by deprotonation and anodic oxidation of the [Co–H] species. In the meantime, protons are reduced to produce hydrogen at the cathode.
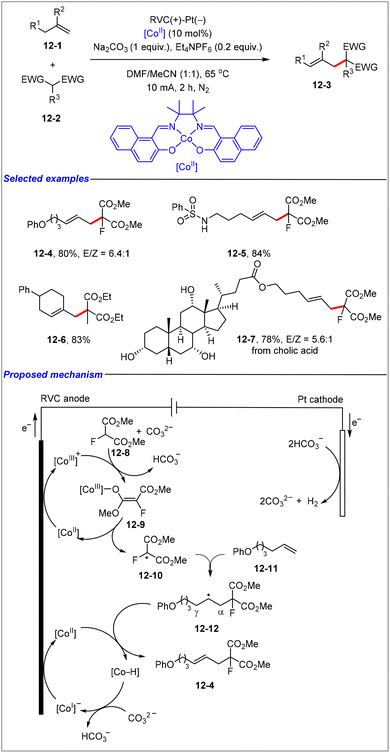 |
| Scheme 12 Electrochemical allylic C–H alkylation. | |
The electrochemical intermolecular cross-coupling of α,β-unsaturated carboxylic acids with 1,3-dicarbonyl derivatives as alkyl radical precursors was studied by Wang and co-workers in 2021 (Scheme 13).37 This protocol employs Cp2Fe as a catalyst to generate 1,3-dicarbonyl radical intermediates from C–H-based 1,3-dicarbonyl derivatives through single-electron transfer without the need for stoichiometric oxidants. Based on mechanism experiments and studies of cyclic voltammograms, the authors propose the following reaction mechanism. MeOH is reduced at the cathode to give H2 and MeO−. The latter removes the proton of dimethyl 2-fluoromalonate 13-7 to give the carbanion intermediate 13-8, which is oxidized by Cp2Fe+ produced through anodic oxidation of Cp2Fe to furnish radical intermediate 13-9 and regenerate Cp2Fe. Then, the intermolecular addition of 13-9 to the conjugate base 13-10 of cinnamic acid affords a new carbon-centered radical 13-11, which undergoes oxidation and elimination of CO2 to afford the product 13-12. The effective single-electron transfer occurs in Cp2Fe (Ep/2 = 0.46 V vs. Ag/AgCl) and carbanion intermediate 13-8 (Ep/2 = 0.45 V vs. Ag/AgCl) rather than in 13-7 (Ep/2 = 1.21 V vs. Ag/AgCl) or 13-10 (Ep/2 = 1.55 V vs. Ag/AgCl) due to the similar oxidation potentials of Cp2Fe and 13-8.
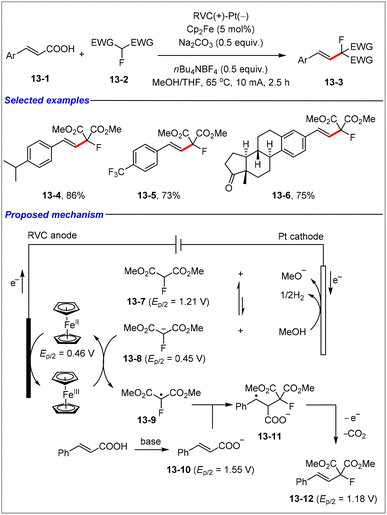 |
| Scheme 13 Electrochemical cross-coupling of α,β-unsaturated carboxylic acids with 1,3-dicarbonyl derivatives. | |
In addition, the monofluorinated 1,3-dicarbonyl radical generated by the electrochemical oxidation of dimethyl 2-fluoromalonate can be used in the electrochemical tandem cyclization of N-arylacrylamides and N-(4-alkoxybenzyl)-acrylamides, which employs the Cp2Fe-catalytic system and obviates the need for prefunctionalized monofluoroalkylation reagents and sacrificial oxidants (Scheme 14).38 A range of monofluorinated 2-oxindoles and 2-azaspiro[4.5]decanes are successfully obtained with satisfactory results under mild conditions. This approach provides a simple and effective route for the synthesis of fluorine-containing heterocyclic molecules.
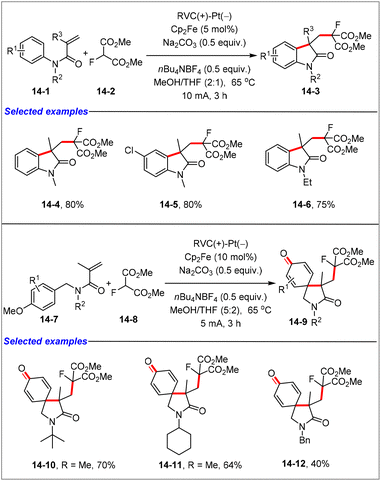 |
| Scheme 14 Electrochemical tandem cyclization of N-arylacrylamides and N-(4-alkoxybenzyl)-acrylamides. | |
During the study of electrochemical cross-coupling of indoles and 1,3-dicarbonyl derivatives, Park and co-workers developed the electrosynthesis of 2,3-dihydrofurans, which is fulfilled in a solution of NaI in MeCN using enamines as radical acceptors (Scheme 15).22 A similar mechanism to the cross-coupling of indoles and 1,3-dicarbonyl derivatives shown in Scheme 4 has been proposed.
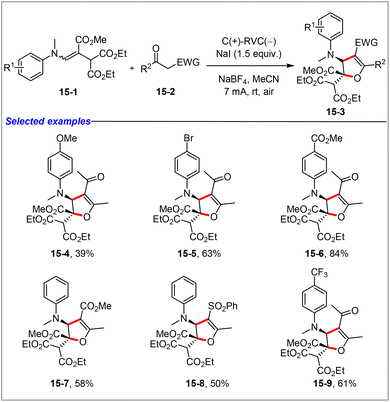 |
| Scheme 15 Electrosynthesis of 2,3-dihydrofurans. | |
Stereoselective radical transformations have been a challenging but hot field in organic synthesis.39 Catalytic asymmetric electrosynthesis combines the advantages of electrosynthesis and asymmetric catalysis, which provides a powerful alternative for achieving stereoselective radical transformation to synthesize chiral compounds.40 In 2018, Meggers and co-workers described the electrochemical oxidative cross-coupling of 2-acyl imidazoles with silyl enol ethers through asymmetric Lewis acid catalysis (Scheme 16).41 A series of high-value 1,4-dicarbonyl compounds containing tertiary- and quaternary-carbon stereocenters are synthesized in moderate to good yields with excellent chemo- and enantio-selectivity. This approach employs a chiral-at-metal rhodium catalyst to activate substrates 16-1 to furnish rhodium-bound intermediates 16-7, which lose a proton to afford enolate intermediates 16-8. The anodic single-electron oxidation of 16-8 furnishes carbon-centered radicals 16-9, which are added to silyl enol ethers 16-2 to form radicals 16-10. Then a second anodic oxidation of 16-10 affords intermediates 16-11. Finally, 16-11 undergo desilylation with MeOH and exchange of substrate/product to furnish products 16-3 and regenerate 16-7. Meanwhile, the cathode reduces protons to form H2. The chiral-at-metal rhodium catalyst not only promotes substrate oxidation by activating the substrate, but also provides high enantioselectivity by chiral induction in this transformation. The oxidation of 16-8 to 16-9 can also be implemented under mild indirect electrolysis conditions with Cp2Fe as the redox mediator. In this respect, Meggers and co-workers reported a Cp2Fe-catalyzed electrochemical enantioselective nucleophilic α-C(sp3)–H alkenylation of 2-acyl imidazoles with alkenyl trifluoroborates by combining electrochemistry with asymmetric catalysis.42 These findings demonstrate the great potential applications of asymmetric organic synthesis through merging chiral catalysts and electrosynthesis.
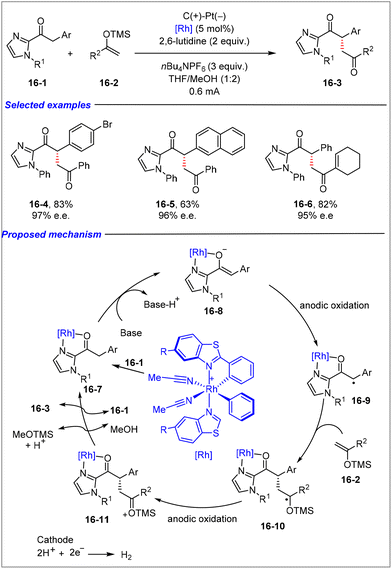 |
| Scheme 16 Asymmetric electrochemical cross-coupling of 2-acyl imidazoles with silyl enol ethers. | |
Tetrasubstituted furans are important structural skeletons present in many natural products and biologically active molecules. The electrochemical C–H activation for the synthesis of tetrasubstituted furans via the intermolecular cyclization of allenes and 1,3-dicarbonyl derivatives was developed by Tang and co-workers (Scheme 17).43 This approach employs cheap Cp2Fe as a redox catalyst and avoids the need for stoichiometric oxidants and noble metal catalysts. Many tetrasubstituted furans are obtained with satisfactory yields under simple electrochemical conditions, and one of these products exhibited good antitumor activity against the T-24 tumor cell line. A radical pathway is proposed by the authors. Firstly, the 1,3-dicarbonyl radical 17-8 produced by Cp2Fe-catalyzed indirect electrolysis adds to allene 17-9 to give radical intermediate 17-10, which loses an electron at the anode to form benzylic carbocation 17-11. Subsequently, the intramolecular nucleophilic cyclization and isomerization of 17-11 yield intermediate 17-12, which is anodized and loses a proton to generate radical 17-13. Finally, 17-13 undergoes anodic oxidation and nucleophilic addition with 17-6 to furnish the product 17-4. Meanwhile, protons are reduced at the cathode to form hydrogen. The intermediate 17-12 is obtained in 63% isolated yield by electrolyzing 17-6 and 17-9 for 1 h, and it can also react with 17-6 to produce the target product 17-4 under these conditions. These findings suggest that 17-12 might be an effective intermediate for this reaction and support the mechanism process proposed by the authors.
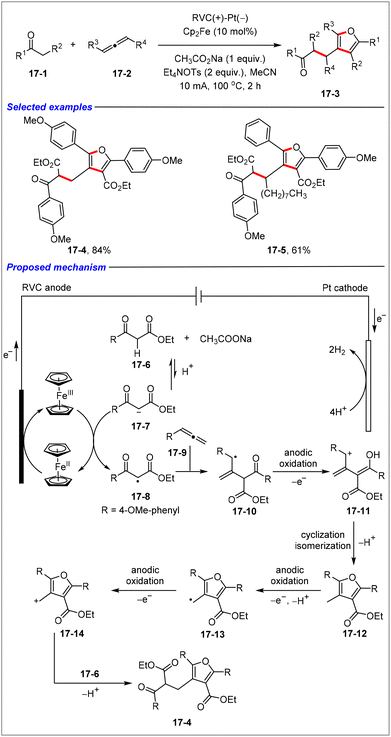 |
| Scheme 17 Electrochemical cyclization of allenes and 1,3-dicarbonyl derivatives to tetrasubstituted furans. | |
4. Addition of 1,3-dicarbonyl radicals to alkynes
1,3-Dicarbonyl radicals generated by electrochemical oxidation can react with intermolecular alkynes to give highly functionalized alkenes, cyclic compounds and ketones, and have attracted widespread attention. In 2020, Pan and co-workers reported an electrochemical intermolecular [4 + 2] annulation of alkynes with 1,3-dicarbonyl derivatives for the synthesis of functionalized 1-naphthols (Scheme 18).44 This method is conducted at a constant potential of 1.15 V vs. Ag/AgCl with Cp2Fe as a redox catalyst and NaOEt as a base in THF/EtOH at 100 °C. The obtained 1-naphthol derivatives demonstrate good in vitro antitumor activity. A possible mechanism for the electrochemical [4 + 2] annulation is proposed. The cathodic reduction of EtOH produces EtO− and H2. The anodic oxidation of Cp2Fe produces Cp2Fe+, which oxidizes 18-9 produced by deprotonation of 18-8 with the assistance of a base to form 1,3-dicarbonyl radical 18-10 and regenerate Cp2Fe. Intermolecular radical addition of 18-10 to alkyne 18-11 followed by a cyclization of 18-12 produces radical intermediate 18-13, which undergoes anodic oxidation and rearomatization to afford final product 18-4.
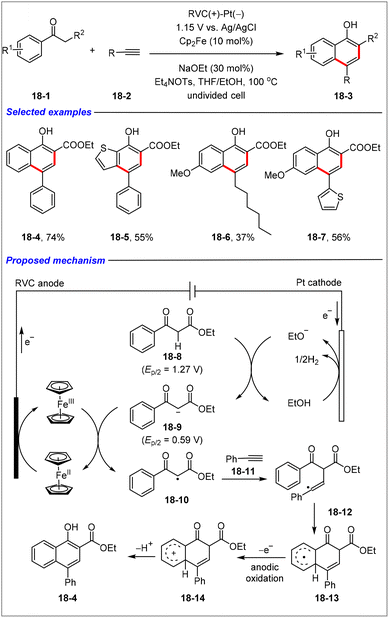 |
| Scheme 18 Electrochemical [4 + 2] annulation of alkynes with 1,3-dicarbonyl derivatives. | |
Spiro[4.5]decanes are widely found in natural products, pharmaceutical molecules and organic functional materials, and are often used as synthetic intermediates in organic synthesis.45 The reported synthesis methods often rely on metal reagents and photocatalysis through the reductive debromination of 2-bromomalonates.46 In 2022, Wang and Hou explored the electrochemical dearomatizing spirocyclization of alkynes with dimethyl 2-benzylmalonates for the synthesis of spiro[4.5]deca-trienones (Scheme 19).47 A variety of spiro compounds are efficiently constructed in satisfactory yields with Cp2Fe as an electrocatalyst, which obviates the use of noble metal reagents, sacrificial oxidants and 2-bromomalonates. The reaction begins with the anodic oxidation of Cp2Fe to Cp2Fe+ and the cathodic reduction of MeOH to MeO− and H2. MeO− reacts with 1,3-dicarbonyl derivative 19-2 to give carbanion intermediate 19-7. Then the single-electron transfer from 19-7 to Cp2Fe+ furnishes a carbon-centered radical 19-8, which undergoes radical addition cyclization with the alkyne to form radical 19-9. The latter is oxidized by Cp2Fe+ and trapped by MeOH to afford intermediate 19-11, which is isolated and identified. Finally, 19-11 undergoes the Sc(OTf)3-mediated hydrolysis to furnish the product 19-3.
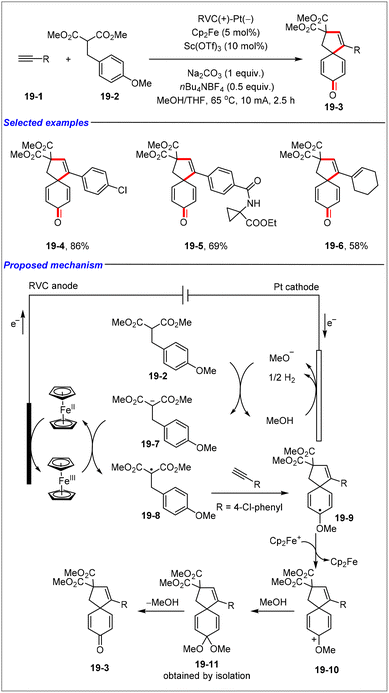 |
| Scheme 19 Electrochemical dearomatizing spirocyclization of alkynes with dimethyl 2-benzylmalonates. | |
By using allyl-substituted 1,3-dicarbonyl derivatives as the radical precursors and readily available alkynes as the radical acceptors, Lei and co-workers developed NaI- or Cp2Fe-catalyzed electrochemical oxidative [3 + 2] annulation with high regioselectivity (Scheme 20).48 Numerous poly-substituted cyclopentenes or cyclopentanes are prepared with high efficiency under mild reaction conditions. On the basis of experimental studies, a possible mechanism involving an iodine radical and a carbon-centered radical for the electrochemical [3 + 2] annulation is proposed. At the anode, I− or Cp2Fe is first oxidized and loses an electron to form an iodine radical or Cp2Fe+, which reacts with the conjugate base of dimethyl 2-allylmalonate 20-1via a single-electron transfer process to furnish a carbon-centered radical 20-13. Then, an intermolecular radical addition of 20-13 to alkynes takes place followed by a cyclization of 20-14 to furnish new carbon-centered radicals 20-15. On the one hand, the radical–radical cross-coupling of 20-15 and the iodine radical affords the products 20-3 (path a). On the other hand, hydrogen atom transfer from the solvent or 1,4-cyclohexadiene (1,4-CHD) to 20-15 forms products 20-8 under Cp2Fe-catalyzed electrochemical conditions (path b).
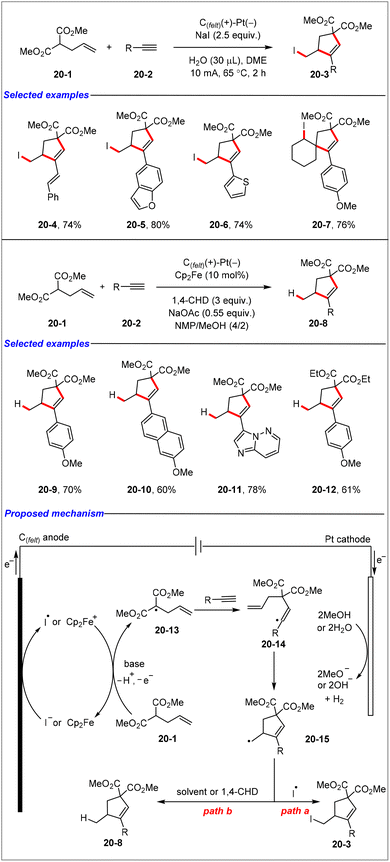 |
| Scheme 20 Electrochemical [3 + 2] annulation of alkynes with allyl substituted 1,3-dicarbonyl derivatives. | |
The direct electrochemical difunctionalization of terminal alkynes with 1,3-dicarbonyl esters or ketones to synthesize 1,4-dicarbonyl compounds was reported by Lei and co-workers in 2021 (Scheme 21).49 The reaction is achieved at 60 °C in a solution of K2CO3 in AcOH/DCE. The radical addition of 21-8 produced by the anodic oxidation of 21-1 to terminal alkynes 21-2 furnished alkenyl radical intermediates 21-9, which are further oxidized by the anode and trapped by H2O produced by the reaction of K2CO3 and AcOH to afford products 21-3.
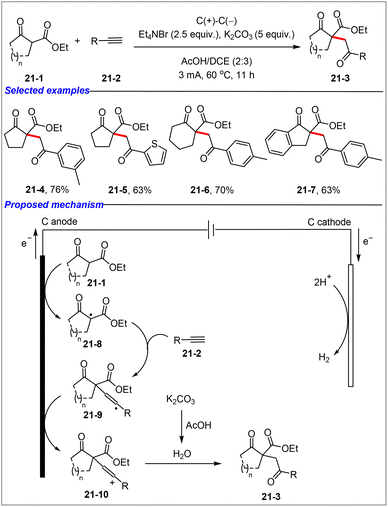 |
| Scheme 21 Electrochemical synthesis of 1,4-dicarbonyl derivatives. | |
In the 2022, Wang and co-workers developed a regio- and stereo-selective electrochemical selenoalkylation of alkynes with 1,3-dicarbonyl compounds and diselenides (Scheme 22).50 The electrosynthesis approach shows good compatibility with alkynes, 1,3-dicarbonyl compounds and diselenides and a lot of highly functionalized alkenes are synthesized with satisfactory efficiency under mild conditions. The authors proposed a possible mechanism for the selenoalkylation of alkynes as follows: 1,3-dicarbonyl radicals 22-9 generated by Cp2Fe-catalyzed anodic oxidation add to alkynes to form alkenyl radical intermediates 22-10. The latter are trapped by diselenides to afford the thermodynamically stable (E)-alkene products 22-4.
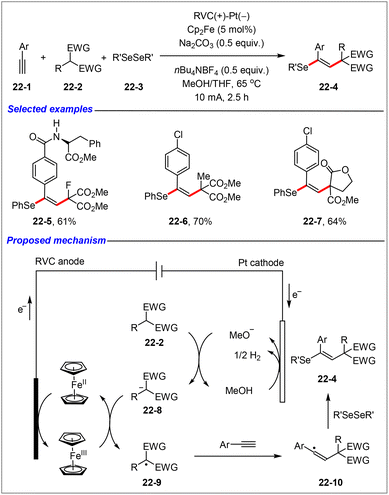 |
| Scheme 22 Electrochemical selenoalkylation of alkynes with 1,3-dicarbonyl derivatives. | |
5. Radical–radical coupling of 1,3-dicarbonyl radicals
5.1 Cross-coupling of 1,3-dicarbonyl radicals
Radical–radical coupling is a straightforward method for the preparation of functionalized molecules through the release of hydrogen. α-Functionalized carbonyl derivatives are a class of important bioactive molecules in organic chemistry. In this respect, He and co-workers developed an electrochemical α-thiolation and α-azidation of 1,3-dicarbonyl derivatives with RSH and TMSN3 (Scheme 23).51 This protocol employs cheap and readily available NH4I as a halogen mediator to access several valuable carbonyl compounds. Based on research results, a radical mechanism for the electrochemical α-thiolation is proposed. The anode oxidizes I− and RSH in parallel to furnish an iodine radical and sulfur radical 23-13. The generated iodine radical reacts with the substrate 23-14 to furnish 1,3-dicarbonyl radical 23-15. The radical–radical cross-coupling of 23-13 and 23-15 affords the target product 23-16. Meanwhile, the cathode reduces protons to form hydrogen.
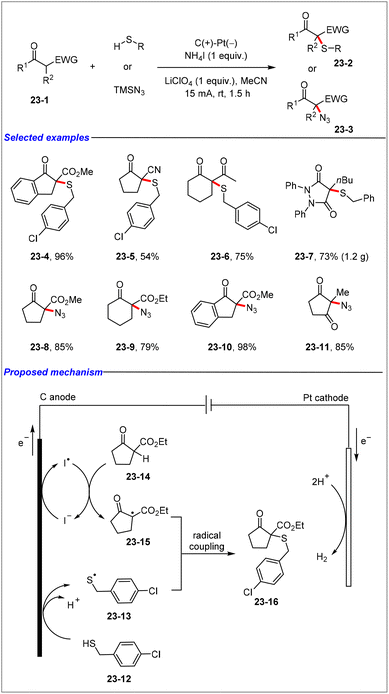 |
| Scheme 23 Electrochemical α-thiolation and azidation of 1,3-dicarbonyl derivatives. | |
The electrochemical intramolecular C–H/N–H dehydrogenative cross-coupling of dimalonate-substituted 2-phenylindoles to afford various isoindolo[2,1-a]indoles was described by Huang and co-workers (Scheme 24).52 The addition of NaI is the key to the success of the reaction and an iodide-promoted intramolecular radical–radical coupling process is proposed. A similar C–H/N–H cross-coupling reaction is also found in the electrochemical dehydrogenative annulation of N-allyl amides with 1,3-dicarbonyl derivatives by Xu and co-workers.29 Under the conditions of non-electrochemical manganese catalysis, the C–H/C–H coupling of dimalonate-substituted 2-phenylindoles can also be achieved in high yields.
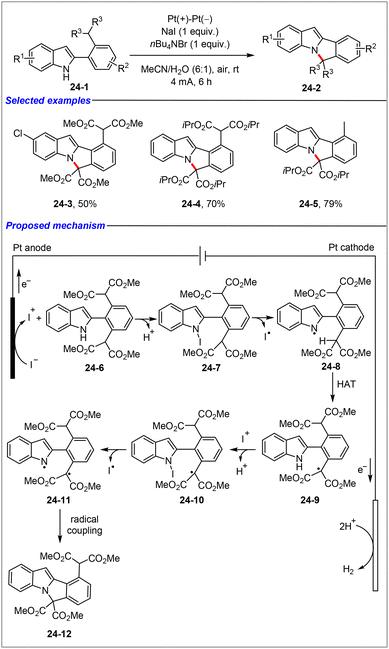 |
| Scheme 24 Electrochemical C–H/N–H cross-coupling of dimalonate-substituted 2-phenylindoles. | |
5.2 Self-coupling of 1,3-dicarbonyl radicals
The self-coupling reactions of 1,3-dicarbonyl radicals have been known for decades.12 In 2021, the electrochemical annulation of 1,3-dicarbonyl derivatives and primary amines for the synthesis of polysubstituted pyrroles was developed by Pan and co-workers (Scheme 25).53 The authors found that enamines are also suitable substrates for the electrochemical transformation under similar conditions, suggesting that the reaction may involve an enamine intermediate. The condensation of 25-7 and 25-8 under heated and acidic conditions furnishes imine 25-9, which tautomerizes to the enamine 25-10. The anodic oxidation of 25-10 forms nitrogen-centered radical intermediate 25-11, which tautomerizes to a more stable 1,3-dicarbonyl radical 25-12. Subsequently, the self-coupling of 25-12 affords dimer 25-13, which undergoes intramolecular cyclization to generate the final product 25-4 with the departure of benzylamine. At the cathode, TfOH is reduced to give H2 and TfO−, in which TfO− can promote the anodic oxidation of 25-10.
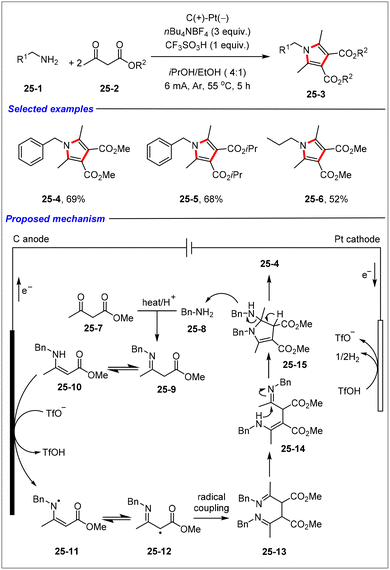 |
| Scheme 25 Electrochemical cyclization of 1,3-dicarbonyl derivatives and primary amines. | |
Recently, TEMPO-catalyzed electrochemical dimerization of three different types of 3-substituted-2-oxindoles at a control potential of only 0.8 V vs. Ag/Ag+ was developed by Bisai and Paul (Scheme 26).54 The oxidation potentials of 3-substituted-2-oxindoles determine that the reaction process involves either a two-electron- or one-electron-transfer pathway. A radical–radical self-coupling process is proposed for the construction of dimerized 3-alkyl-2-oxindoles. The direct electrolysis for the dimerization of 3-substituted 2-oxindoles with NaH as a base was also developed by their group in 2020.55 This protocol involves a proton-coupled electron-transfer process and avoids the need for catalysts, chemical oxidants and metal reagents, which can be used as a key step to achieve the total synthesis of (±)-folicanthine and formal total syntheses of (±)-chimonanthine and (±)-calycanthine.56
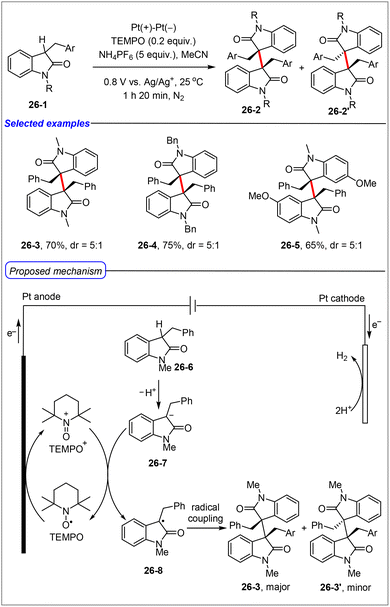 |
| Scheme 26 Electrochemical dimerization of 3-alkyl-2-oxindoles. | |
6. Conclusions
Electrosynthesis, as an environmentally friendly synthesis technology, is an efficient and sustainable method for the generation of 1,3-dicarbonyl radicals from C–H-based 1,3-dicarbonyl derivatives. 1,3-Dicarbonyl radicals serve as useful intermediates in various chemical conversions. In this review, we have briefly generalized the recent advances in the electrochemical generation of 1,3-dicarbonyl radicals from C–H bonds and focused on the mechanistic insights and synthetic applications of these transformations.
Although great efforts have been made in electrosynthesis, many challenges still remain to be further explored, such as developing new organic catalysts and novel chemical transformations. Moreover, 1,3-dicarbonyl derivatives, which act as readily available ligands and radical precursors, have great potential in asymmetric organic synthesis. Meanwhile, the radical transformation of unactivated C–H bonds is a very important and challenging subject, and current electrochemical reports in this field are rare. 1,3-Dicarbonyl derivatives are common synthetic intermediates and are widely used to synthesize natural products or drug molecules, but the related electrochemical approaches for the synthesis of active molecules still need to be further developed. In addition, complex electrolytic systems or devices and expensive electrodes are required to achieve the electrochemical transformations. The electrosynthesis methods can be fulfilled under simple and mild conditions by combining with other methods and techniques, such as the use of functionalized electrodes, alternating current, flow chemistry, artificial intelligence, etc. We believe that more reports in these directions will be published in the near future.
Conflicts of interest
There are no conflicts to declare.
Acknowledgements
We gratefully acknowledge the National Natural Science Foundation of China (22101201, 22071171) and the Natural Science Foundation of Zhejiang Province (LQ22B020005 and LZ22B020003) for their financial support of this work.
References
-
(a) M. Yan, Y. Kawamata and P. S. Baran, Synthetic Organic Electrochemical Methods since 2000: On the Verge of a Renaissance, Chem. Rev., 2017, 117, 13230–13319 CrossRef CAS PubMed
;
(b) A. Wiebe, T. Gieshoff, S. Möhle, E. Rodrigo, M. Zirbes and S. R. Waldvogel, Electrifying Organic Synthesis, Angew. Chem., Int. Ed., 2018, 57, 5594–5619 CrossRef CAS PubMed
;
(c) Y. Jiang, K. Xu and C. Zeng, Use of Electrochemistry in the Synthesis of Heterocyclic Structures, Chem. Rev., 2018, 118, 4485–4540 CrossRef CAS PubMed
;
(d) P. Xiong and H.-C. Xu, Chemistry with Electrochemically Generated N-Centered Radicals, Acc. Chem. Res., 2019, 52, 3339–3350 CrossRef CAS PubMed
;
(e) S.-H. Shi, Y. Liang and N. Jiao, Electrochemical Oxidation Induced Selective C–C Bond Cleavage, Chem. Rev., 2021, 121, 485–505 CrossRef CAS PubMed
;
(f) L. F. T. Novaes, J. Liu, Y. Shen, L. Lu, J. M. Meinhardt and S. Lin, Electrocatalysis as an Enabling Technology for Organic Synthesis, Chem. Soc. Rev., 2021, 50, 7941–8002 RSC
;
(g) M.-J. Luo, Q. Xiao and J.-H. Li, Electro-/Photocatalytic Alkene-Derived Radical Cation Chemistry: Recent Advances in Synthetic Applications, Chem. Soc. Rev., 2022, 51, 7206–7237 RSC
.
-
(a) Y. Yuan and A. Lei, Electrochemical Oxidative Cross-Coupling with Hydrogen Evolution Reactions, Acc. Chem. Res., 2019, 52, 3309–3324 CrossRef CAS PubMed
;
(b) S. D. Minteer and P. S. Baran, Electrifying Synthesis: Recent Advances in the Methods, Materials, and Techniques for Organic Electrosynthesis, Acc. Chem. Res., 2020, 53, 545–546 CrossRef CAS PubMed
;
(c) Y. Yu, J.-S. Zhong, K. Xu, Y. Yuan and K.-Y. Ye, Recent Advances in the Electrochemical Synthesis and Functionalization of Indole Derivatives, Adv. Synth. Catal., 2020, 362, 2102–2119 CrossRef
;
(d) Z. Zou, W. Zhang, Y. Wang and Y. Pan, Recent Advances in Electrochemically Driven Radical Fluorination and Fluoroalkylation, Org. Chem. Front., 2021, 8, 2786–2798 RSC
;
(e) P. R. D. Murray, J. H. Cox, N. D. Chiappini, C. B. Roos, E. A. McLoughlin, B. G. Hejna, S. T. Nguyen, H. H. Ripberger, J. M. Ganley, E. Tsui, N. Y. Shin, B. Koronkiewicz, G. Qiu and R. R. Knowles, Photochemical and Electrochemical Applications of Proton-Coupled Electron Transfer in Organic Synthesis, Chem. Rev., 2022, 122, 2017–2291 CrossRef CAS PubMed
;
(f) N. E. S. Tay, D. Lehnherr and T. Rovis, Photons or Electrons? A Critical Comparison of Electrochemistry and Photoredox Catalysis for Organic Synthesis, Chem. Rev., 2022, 122, 2487–2649 CrossRef CAS PubMed
;
(g) Z.-W. Hou, H.-C. Xu and L. Wang, Electrochemical Dehydrogenative N–H/N–H Coupling Reactions, Curr. Opin. Electrochem., 2022, 34, 100988 CrossRef CAS
;
(h) Z. Wang, Y. Sun, L.-Y. Shen, W.-C. Yang, F. Meng and P. Li, Photochemical and Electrochemical Strategies in C–F Bond Activation and Functionalization, Org. Chem. Front., 2022, 9, 853–873 RSC
.
-
(a) K.-J. Jiao, Y.-K. Xing, Q.-L. Yang, H. Qiu and T.-S. Mei, Site-Selective C–H Functionalization via Synergistic Use of Electrochemistry and Transition Metal Catalysis, Acc. Chem. Res., 2020, 53, 300–310 CrossRef CAS PubMed
;
(b) L. Ackermann, Metalla-Electrocatalyzed C–H Activation by Earth-Abundant 3d Metals and Beyond, Acc. Chem. Res., 2020, 53, 84–104 CrossRef CAS PubMed
;
(c) J.-S. Zhong, Y. Yu, Z. Shi and K.-Y. Ye, An Electrochemical Perspective on the Roles of Ligands in the Merger of Transition-Metal Catalysis and Electrochemistry, Org. Chem. Front., 2021, 8, 1315–1328 RSC
.
-
(a) J. P. Barham and B. König, Synthetic Photoelectrochemistry, Angew. Chem., Int. Ed., 2020, 59, 11732–11174 CrossRef CAS PubMed
;
(b) Y. Yu, P. Guo, J.-S. Zhong, Y. Yuan and K.-Y. Ye, Merging Photochemistry with Electrochemistry in Organic Synthesis, Org. Chem. Front., 2020, 7, 131–135 RSC
;
(c) L. Capaldo, L. L. Quadri and D. Ravelli, Merging Photocatalysis with Electrochemistry: The Dawn of a new Alliance in Organic Synthesis, Angew. Chem., Int. Ed., 2019, 58, 17508–17510 CrossRef CAS PubMed
;
(d) S. Wu, J. Kaur, T. A. Karl, X. Tian and J. P. Barham, Synthetic Molecular Photoelectrochemistry: New Frontiers in Synthetic Applications, Mechanistic Insights and Scalability, Angew. Chem., Int. Ed., 2022, 61, e202107811 CAS
;
(e) H. Huang, K. A. Steiniger and T. H. Lambert, Electrophotocatalysis: Combining Light and Electricity to Catalyze Reactions, J. Am. Chem. Soc., 2022, 144, 12567–12583 CrossRef CAS PubMed
;
(f) J. Liu, L. Lu, D. Wood and S. Lin, New Redox Strategies in Organic Synthesis by Means of Electrochemistry and Photochemistry, ACS Cent. Sci., 2020, 6, 1317–1340 CrossRef CAS PubMed
;
(g) Z. Wang, L. Wang, Z. Wang, P. Zhang and Y. Li, A Practical Synthesis of α-Bromo/iodo/chloroketones from Olefins under Visible-light Irradiation Conditions, Chin. Chem. Lett., 2021, 32, 429–432 CrossRef CAS
;
(h) Q. Wan, Z.-W. Hou, X.-R. Zhao, X. Xie and L. Wang, Organoelectrophotocatalytic C–H Silylation of Heteroarenes, Org. Lett., 2023, 25, 1008–1013 CrossRef CAS PubMed
.
-
(a) Q. Lin, L. Li and S. Luo, Asymmetric Electrochemical Catalysis, Chem. – Eur. J., 2019, 25, 10033–10044 CrossRef CAS PubMed
;
(b) Q. Lin and S. Luo, Tailoring Radicals by Asymmetric Electrochemical Catalysis, Org. Chem. Front., 2020, 7, 2997–3000 RSC
;
(c) K. Yamamoto, M. Kuriyama and O. Onomura, Asymmetric Electrosynthesis: Recent Advances in Catalytic Transformations, Curr. Opin. Electrochem., 2021, 28, 100714 CrossRef CAS
;
(d) K.-J. Jiao, Z.-H. Wang, C. Ma, H.-L. Liu, B. Cheng and T.-S. Mei, The Applications of Electrochemical Synthesis in Asymmetric Catalysis, Chem. Catal., 2022, 2, 3019–3047 CrossRef CAS
.
-
(a) W. Zi, Z. Zuo and D. Ma, Intramolecular Dearomative Oxidative Coupling of Indoles: A Unified Strategy for the Total Synthesis of Indoline Alkaloids, Acc. Chem. Res., 2015, 48, 702–711 CrossRef CAS PubMed
;
(b) C. Zhu, Z. Liu, G. Chen, K. Zhang and H. Ding, Total Synthesis of Indole Alkaloid Alsmaphorazine D, Angew. Chem., Int. Ed., 2015, 54, 879–882 CrossRef CAS PubMed
;
(c) H. Yokoe, C. Mitsuhashi, Y. Matsuoka, T. Yoshimura, M. Yoshida and K. Shishido, Enantiocontrolled Total Syntheses of Breviones A, B, and C, J. Am. Chem. Soc., 2011, 133, 8854–8857 CrossRef CAS PubMed
.
-
(a) H. Jiang, Y. Cheng, Y. Zhang and S. Yu, De Novo Synthesis of Polysubstituted Naphthols and Furans Using Photoredox Neutral Coupling of Alkynes with 2-Bromo-1,3-dicarbonyl Compounds, Org. Lett., 2013, 15, 4884–4887 CrossRef CAS PubMed
;
(b) L. Wang, W. Huang, R. Li, D. Gehrig, P. W. Blom, K. Landfester and K. A. I. Zhang, Structural Design Principleof Small-Molecule Organic Semiconductors for Metal-Free, Visible-Light-Promoted Photocatalysis, Angew. Chem., Int. Ed., 2016, 55, 9783–9787 CrossRef CAS PubMed
;
(c) D. Fernandez Reina, A. Ruffoni, Y. S. S. Al-Faiyz, J. J. Douglas, N. S. Sheikh and D. Leonori, Visible-Light-Mediated Reactions of Electrophilic Radicals with Vinyl and Allyl Trifluoroborates, ACS Catal., 2017, 7, 4126–4130 CrossRef CAS
;
(d) J. Huo, X. Geng, W. Li, P. Zhang and L. Wang, A Traceless Heterocyclic Amine Mediator in Regioselectivity–Switchable Formal [1 + 2 + 2] Cycloaddition Reaction to 1,3,4- and 1,4,5-Trisubstituted Pyrazoles, Org. Lett., 2023, 25, 512–516 CrossRef CAS PubMed
.
-
(a) J. A. Labinger, Platinum-Catalyzed C–H Functionalization, Chem. Rev., 2017, 117, 8483–8496 CrossRef CAS PubMed
;
(b) Y. Qin, L. Zhu and S. Luo, Organocatalysis in Inert C–H Bond Functionalization, Chem. Rev., 2017, 117, 9433–9520 CrossRef CAS PubMed
;
(c) H. Wang, X. Gao, Z. Lv, T. Abdelilah and A. Lei, Recent Advances in Oxidative R1-H/R2-H Cross-Coupling with Hydrogen Evolution via Photo-/Electrochemistry, Chem. Rev., 2019, 119, 6769–6787 CrossRef CAS PubMed
;
(d) N. Holmberg-Douglas and D. A. Nicewicz, Photoredox-Catalyzed C–H Functionalization Reactions, Chem. Rev., 2022, 122, 1925–2016 CrossRef CAS PubMed
;
(e) P. Bellotti, H.-M. Huang, T. Faber and F. Glorius, Photocatalytic Late-Stage C–H Functionalization, Chem. Rev., 2003, 123, 4237–4352 CrossRef PubMed
;
(f) Y. Lv, Z.-W. Hou, P. Li and L. Wang, Paired Electrochemical C–H Bromination of (Hetero)Arenes with 2-Bromoethan-1-ol, Org. Chem. Front., 2023, 10, 990–995 RSC
.
-
(a) B. B. Snider, Manganese(III)-Based Oxidative Free-Radical Cyclizations, Chem. Rev., 1996, 96, 339–363 CrossRef CAS PubMed
;
(b) V. Nair and A. Deepthi, Cerium(IV) Ammonium Nitrates-A Versatile Single-Electron Oxidant, Chem. Rev., 2007, 107, 1862–1891 CrossRef CAS PubMed
;
(c) M.-Q. Tian, C. Wang, X.-H. Hu and T.-P. Loh, Divergent C–H Oxidative Radical Functionalization of Olefins to Install Tertiary Alkyl Motifs Enabled by Copper Catalysis, Org. Lett., 2019, 21, 1607–1611 CrossRef CAS PubMed
;
(d) M.-Q. Tian, Z.-Y. Shen, X. Zhao, P. J. Walsh and X.-H. Hu, Iron-Catalyzed Tertiary Alkylation of Terminal Alkynes with 1,3-Diesters via a Functionalized Alkyl Radical, Angew. Chem., Int. Ed., 2021, 60, 9706–9711 CrossRef CAS PubMed
.
-
(a) P. Qian, B. Du, R. Song, X. Wu, H. Mei, J. Han and Y. Pan,
N-Iodosuccinimide-Initiated Spirocyclopropanation of Styrenes with 1,3-Dicarbonyl Compound for the Synthesis of Spirocyclopropanes, J. Org. Chem., 2016, 81, 6546–6553 CrossRef CAS PubMed
;
(b) T. Li, K. Liang, Y. Zhang, D. Hu, Z. Ma and C. Xia, Three-Component Minisci Reaction with 1,3-Dicarbonyl Compounds Induced by Visible Light, Org. Lett., 2020, 22, 2386–2390 CrossRef CAS PubMed
;
(c) J.-D. Guo, X.-L. Yang, B. Chen, C.-H. Tung and L.-Z. Wu, Photoredox/Cobalt-Catalyzed C(sp3)–H Bond Functionalization toward Phenanthrene Skeletons with Hydrogen Evolution, Org. Lett., 2020, 22, 9627–9632 CrossRef CAS PubMed
;
(d) J. Zhang, S. R. Paladugu, R. M. Gillard, A. Sarkar and D. L. Boger, Tris(4-bromophenyl)aminium Hexachloroantimonate-Mediated Intermolecular C(sp2)–C(sp3) Free Radical Coupling of Vindoline with β-Ketoesters and Related Compounds, J. Am. Chem. Soc., 2022, 144, 495–502 CrossRef CAS PubMed
.
-
(a) G. Yuan, Z. Zhu, X. Gao and H. Jiang, Electrochemically Promoted Synthesis of Polysubstituted Oxazoles from β-Diketone Derivatives and Benzylamines under Mild Conditions, RSC Adv., 2014, 4, 24300–24303 RSC
;
(b) L.-S. Kang, M.-H. Luo, C. M. Lam, L.-M. Hu, R. D. Little and C.-C. Zeng, Electrochemical C–H Functionalization and Subsequent C–S and C–N Bond Formation: Paired Electrosynthesis of 3-Amino-2-thiocyanato-α,β-unsaturated Carbonyl Derivatives Mediated by Bromide Ions, Green Chem., 2016, 18, 3767–3774 RSC
;
(c) J. Strehl and G. Hilt, Electrochemical, Manganese-Assisted Carbon–Carbon Bond Formation between β-Keto Esters and Silyl Enol Ethers, Org. Lett., 2019, 21, 5259–5263 CrossRef CAS PubMed
;
(d) Y. Wang, B. Tian, M. Ding and Z. Shi, Electrochemical
Cross-Dehydrogenative Coupling between Phenols and β-Dicarbonyl Compounds: Facile Construction of Benzofurans, Chem. – Eur. J., 2020, 26, 4297–4303 CrossRef CAS PubMed
;
(e) L. Li, Y. Li, N. Fu, L. Zhang and S. Luo, Catalytic Asymmetric Electrochemical α-Arylation of Cyclic β-Ketocarbonyls with Anodic Benzyne Intermediates, Angew. Chem., Int. Ed., 2020, 59, 14347–14351 CrossRef CAS PubMed
;
(f) Y.-Z. Yang, Y.-C. Wu, R.-J. Song and J.-H. Li, Electrochemical Dehydrogenative Cross-Coupling of Xanthenes with Ketones, Chem. Commun., 2020, 56, 7585–7588 RSC
.
-
(a) T. Okubo and S. Tsutsumi, The Anodic Oxidation of Esters, Bull. Chem. Soc. Jpn., 1964, 37, 1794–1797 CrossRef CAS
;
(b) M. T. Ismail, Electrosynthesis of Organic Compounds. XL Synthesis of Some Arylmalononitrile Derivatives, J. Appl. Electrochem., 1987, 17, 881–883 CrossRef CAS
;
(c) M. I. Elinson, S. K. Fedukovich, B. I. Ugrak and G. I. Nikishin, Electrochemical Oxidation of Tetramethyl Esters of α,α,ω,ω-Alcaneteracarboxylic Acids, Russ. Chem. Bull., 1992, 41, 1827–1833 CrossRef
.
-
(a) R. Francke and R. D. Little, Redox Catalysis in Organic Electrosynthesis: Basic Principles and Recent Developments, Chem. Soc. Rev., 2014, 43, 2492–2521 RSC
;
(b) C. Ma, P. Fang, Z.-R. Liu, S.-S. Xu, K. Xu, X. Cheng, A. Lei, H.-C. Xu, C. Zeng and T.-S. Mei, Recent Advances in Organic Electrosynthesis Employing Transition Metal Complexes as Electrocatalysts, Sci. Bull., 2021, 66, 2412–2429 CrossRef CAS PubMed
;
(c) X. Cheng, A. Lei, T.-S. Mei, H.-C. Xu, K. Xu and C. Zeng, Recent Applications of Homogeneous Catalysis in Electrochemical Organic Synthesis, CCS Chem., 2022, 4, 1120–1152 CrossRef CAS
.
-
(a) C. Marti and E. M. Carreira, Construction of Spiro[pyrrolidine-3,3′-oxindoles]-Recent Applications to the Synthesis of Oxindole Alkaloids, Eur. J. Org. Chem., 2003, 2209–2219 CrossRef CAS
;
(b) C. V. Galliford and K. A. Scheidt, Pyrrolidinyl-Spirooxindole Natural Products as Inspirations for the Development of Potential Therapeutic Agents, Angew. Chem., Int. Ed., 2007, 46, 8748–8758 CrossRef CAS PubMed
;
(c) J. J. Badillo, N. V. Hanhan and A. K. Franz, Enantioselective Synthesis of Substituted Oxindoles and Spirooxindoles with Applications in Drug Discovery, Curr. Opin. Drug Discovery Dev., 2010, 13, 758–776 CAS
.
-
(a) D. O'Hagan, Understanding Organofluorine Chemistry. An Introduction to the C–F Bond, Chem. Soc. Rev., 2008, 37, 308–319 RSC
;
(b) R. Berger, G. Resnati, P. Metrangolo, E. Weber and J. Hulliger, Organic Fluorine Compounds: A Great Opportunity for Enhanced Materials Properties, Chem. Soc. Rev., 2011, 40, 3496–3508 RSC
;
(c) C. Ni and J. Hu, The Unique Fluorine Effects in Organic Reactions: Recent Facts and Insights into Fluoroalkylations, Chem. Soc. Rev., 2016, 45, 5441–5454 RSC
.
-
(a) Y. Hamashima, T. Suzuki, H. Takano, Y. Shimura and M. Sodeoka, Catalytic Enantioselective Fluorination of Oxindoles, J. Am. Chem. Soc., 2005, 127, 10164–10165 CrossRef CAS PubMed
;
(b) T. Ishimaru, N. Shibata, T. Horikawa, N. Yasuda, S. Nakamura, T. Toru and M. Shiro, Cinchona Alkaloid Catalyzed Enantioselective Fluorination of Allyl Silanes, Silyl Enol Ethers, and Oxindoles, Angew. Chem., Int. Ed., 2008, 47, 4157–4161 CrossRef CAS PubMed
;
(c) L. L. Wu, L. Falivene, E. Drinkel, S. Grant, A. Linden, L. Cavallo and R. Dorta, Synthesis of 3-Fluoro-3-Aryl Oxindoles: Direct Enantioselective α-Arylation of Amides, Angew. Chem., Int. Ed., 2012, 51, 2870–2873 CrossRef CAS PubMed
.
- Z.-J. Wu and H.-C. Xu, Synthesis of C3-Fluorinated Oxindoles through Reagent-Free Cross-Dehydrogenative Coupling, Angew. Chem., Int. Ed., 2017, 56, 4734–4738 CrossRef CAS PubMed
.
-
(a) U. Jahn, M. Muller and S. Aussieker, The Combination of Anionic and Radical Reactions to Oxidative Tandem Processes Exemplified by the Synthesis of Functionalized Pyrrolidines, J. Am. Chem. Soc., 2000, 122, 5212–5213 CrossRef CAS
;
(b) U. Jahn, P. Hartmann and E. Kaasalainen, Efficient Oxidative Radical Cyclizations of Ester Enolates with Carbocation Desilylation as Termination: Synthesis of Cyclopentanoid Monoterpenes and Analogues, Org. Lett., 2004, 6, 257–260 CrossRef CAS PubMed
;
(c) F. Kafka, M. Holan, D. Hidasova, R. Pohl, I. Cisarova, B. Klepetarova and U. Jahn, Oxidative Catalysis Using the Stoichiometric Oxidant as a Reagent: An Efficient Strategy for Single-Electron-Transfer-Induced Tandem Anion-Radical Reactions, Angew. Chem., Int. Ed., 2014, 53, 9944–9948 CrossRef CAS PubMed
.
- Z.-J. Wu, S.-R. Li, H. Long and H.-C. Xu, Electrochemical Dehydrogenative Cyclization of 1,3-Dicarbonyl Compounds, Chem. Commun., 2018, 54, 4601–4604 RSC
.
- D. R. Artis, I.-S. Cho and J. M. Muchowski, Radical-Based Syntheses of 5-Benzoyl-1,2-dihydro-3H-pyrrolo[1,2-a]pyrrole-1-carboxylic Acid (Ketorolac), Can. J. Chem., 1992, 70, 1838–1842 CrossRef CAS
.
- R. Mei, C. Yang, F. Xiong, M. Mao, H. Li, J. Sun, L. Zou, W. Ma and L. Ackermann, Access to 10-Phenanthrenols via Electrochemical C–H/C–H Arylation, Adv. Synth. Catal., 2021, 363, 1120–1125 CrossRef CAS
.
- S. Choi, J. Park, E. Yu, J. Sim and C.-M. Park, Electrosynthesis of Dihydropyrano[4,3-b]indoles Based on a Double Oxidative [3 + 3] Cycloaddition, Angew. Chem., Int. Ed., 2020, 59, 11886–11891 CrossRef CAS PubMed
.
- X. Chang, X. Chen and P. Sun, Electrochemical [3 + 2] Cycloaddition of Anilines and 1,3-Dicarbonyl Compounds: Construction of Multisubstituted Indoles, Adv. Synth. Catal., 2022, 364, 2865–2871 CrossRef CAS
.
- J.-i. Yoshida, S. Nakatani, K. Sakaguchi and S. Isoe, Formal [2 + 2 + 2] Cycloaddition of Molecular Oxygen, 1,3-Diketone, and Olefin. Synthesis and Reactions of Cyclic Peroxides, J. Org. Chem., 1989, 54, 3383–3389 CrossRef CAS
.
- C.-Y. Cai, Z.-J. Wu, J.-Y. Liu, M. Chen, J. Song and H.-C. Xu, Tailored Cobalt-salen Complexes Enable Electrocatalytic Intramolecular Allylic C–H Functionalizations, Nat. Commun., 2021, 12, 3745 CrossRef CAS PubMed
.
- L.-H. Jie, B. Guo, J. Song and H.-C. Xu, Organoelectrocatalysis Enables Direct Cyclopropanation of Methylene Compounds, J. Am. Chem. Soc., 2022, 144, 2343–2350 CrossRef CAS PubMed
.
- E. J. Corey and M. Chaykovsky, Dimethyloxosulfonium Methylide ((CH3)2SOCH2) and Dimethylsulfonium Methylide ((CH3)2SCH2). Formation and Application to Organic Synthesis, J. Am. Chem. Soc., 1965, 87, 1353–1364 CrossRef CAS
.
- L. Chen, L. M. Barton, J. C. Vantourout, Y. Xu, C. Chu, E. C. Johnson, J. J. Sabatini and P. S. Baran, Electrochemical Cyclobutane Synthesis in Flow: Scale-Up of a Promising Melt-Castable Energetic Intermediate, Org. Process Res. Dev., 2020, 25, 2639–2645 CrossRef
.
- Z.-J. Wu, S.-R. Li and H.-C. Xu, Synthesis of N-Heterocycles by Dehydrogenative Annulation of N-Allyl Amides with 1,3-Dicarbonyl Compounds, Angew. Chem., Int. Ed., 2018, 57, 14070–14074 CrossRef CAS PubMed
.
- M. Xiong, X. Liang, X. Liang, Y. Pan and A. Lei, Hexafluoro-2-Propanol-Promoted Electro-Oxidative [3 + 2] Annulation of 1,3-Dicarbonyl Compounds and Alkenes, ChemElectroChem, 2019, 6, 3383–3386 CrossRef CAS
.
-
(a) B. B. Snider, Manganese(III)-Based Oxidative Free-Radical Cyclizations, Chem. Rev., 1996, 96, 339–363 CrossRef CAS PubMed
;
(b) B. B. Snider, Mechanisms of Mn(OAc)3-B ased Oxidative Free-Radical Additions and Cyclizations, Tetrahedron, 2009, 65, 10738–10744 CrossRef CAS PubMed
;
(c) M. Mondala and U. Bora, Recent Advances in Manganese(III) Acetate Mediated
Organic Synthesis, RSC Adv., 2013, 3, 18716–18754 RSC
.
- N. Fu, Y. Shen, A. R. Allen, L. Song, A. Ozaki and S. Lin, Mn-Catalyzed Electrochemical Chloroalkylation of Alkenes, ACS Catal., 2019, 9, 746–754 CrossRef CAS PubMed
.
-
(a) G. S. Sauer and S. Lin, An Electrocatalytic Approach to the Radical Difunctionalization of Alkenes, ACS Catal., 2018, 8, 5175–5187 CrossRef CAS
;
(b) K. Ye, G. Pombar, N. Fu, G. S. Sauer, I. Keresztes and S. Lin, Anodically Coupled Electrolysis for the Heterodifunctionalization of Alkenes, J. Am. Chem. Soc., 2018, 140, 2438–2441 CrossRef CAS PubMed
.
-
(a) O. Pàmies, J. Margalef, S. Cañellas, J. James, E. Judge, P. J. Guiry, C. Moberg, J.-E. Bäckvall, A. Pfaltz, M. A. Pericàs and M. Diéguez, Recent Advances in Enantioselective Pd-Catalyzed Allylic Substitution: From Design to Applications, Chem. Rev., 2021, 121, 4373–4505 CrossRef PubMed
;
(b) Q. Cheng, H.-F. Tu, C. Zheng, J.-P. Qu, G. Helmchen and S.-L. You, Iridium-Catalyzed Asymmetric Allylic Substitution Reactions, Chem. Rev., 2019, 119, 1855–1969 CrossRef CAS PubMed
.
-
(a) C. Ma, P. Fang and T.-S. Mei, Recent Advances in C–H Functionalization Using Electrochemical Transition Metal Catalysis, ACS Catal., 2018, 8, 7179–7189 CrossRef CAS
;
(b) C. A. Malapit, M. B. Prater, J. R. Cabrera-Pardo, M. Li, T. D. Pham, T. P. McFadden, S. Blank and S. D. Minteer, Advances on the Merger of Electrochemistry and Transition Metal Catalysis for Organic Synthesis, Chem. Rev., 2022, 122, 3180–3218 CrossRef CAS PubMed
.
- M. Chen, Z.-J. Wu, J. Song and H.-C. Xu, Electrocatalytic Allylic C–H Alkylation Enabled by a Dual-Function Cobalt Catalyst, Angew. Chem., Int. Ed., 2022, 61, e202115954 CAS
.
- Z.-W. Hou, T. Jiang, T.-X. Wu and L. Wang, Electrochemical Intermolecular Monofluoroalkylation of α,β-Unsaturated Carboxylic Acids and Heteroaromatics with 2-Fluoromalonate Esters, Org. Lett., 2021, 23, 8585–8589 CrossRef CAS PubMed
.
- Y. Lv, Z.-W. Hou, Y. Wang, P. Li and L. Wang, Electrochemical Monofluoroalkylation Cyclization of N-Arylacrylamides to Construct Monofluorinated 2-Oxindoles, Org. Biomol. Chem., 2023, 21, 1014–1020 RSC
.
- D. A. Nagib, Asymmetric Catalysis in Radical Chemistry, Chem. Rev., 2022, 122, 15989–15992 CrossRef CAS PubMed
.
-
(a) Q. Zhang, X. Chang, L. Peng and C. Guo, Asymmetric Lewis Acid Catalyzed Electrochemical Alkylation, Angew. Chem., Int. Ed., 2019, 58, 6999–7003 CrossRef CAS PubMed
;
(b) P.-S. Gao, X.-J. Weng, Z.-H. Wang, C. Zheng, B. Sun, Z.-H. Chen, S.-L. You and T.-S. Mei, CuII/TEMPO-Catalyzed Enantioselective C(sp3)–H Alkynylation of Tertiary Cyclic Amines through Shono-Type Oxidation, Angew. Chem., Int. Ed., 2020, 59, 15254–15259 CrossRef CAS PubMed
;
(c) C.-J. Long, H. Cao, B.-K. Zhao, Y.-F. Tan, Y.-H. He, C.-S. Huang and Z. Guan, Merging the Non-Natural Catalytic Activity of Lipase and Electrosynthesis: Asymmetric Oxidative Cross-Coupling of Secondary Amines with Ketones, Angew. Chem., Int. Ed., 2022, 61, e2022036 CrossRef PubMed
.
- X. Huang, Q. Zhang, J. Lin, K. Harms and E. Meggers, Electricity-Driven Asymmetric Lewis Acid Catalysis, Nat. Catal., 2018, 2, 34–40 CrossRef
.
- P. Xiong, M. Hemming, S. I. Ivlev and E. Meggers, Electrochemical Enantioselective Nucleophilic α-C(sp3)–H Alkenylation of 2-Acyl Imidazoles, J. Am. Chem. Soc., 2022, 144, 6964–6971 CrossRef CAS PubMed
.
- M.-X. He, Y. Yao, C.-Z. Ai, Z.-Y. Mo, Y.-Z. Wu, Q. Zhou, Y.-M. Pan and H.-T. Tang, Electrochemically-Mediated C–H Functionalization of Allenes and 1,3-Dicarbonyl Compounds to Construct Tetrasubstituted Furans, Org. Chem. Front., 2022, 9, 781–787 RSC
.
- M.-X. He, Z.-Y. Mo, Z.-Q. Wang, S.-Y. Cheng, R.-R. Xie, H.-T. Tang and Y.-M. Pan, Electrochemical Synthesis of 1-Naphthols by Intermolecular Annulation of Alkynes with 1,3-Dicarbonyl Compounds, Org. Lett., 2020, 22, 724–728 CrossRef CAS PubMed
.
-
(a) H. Sorek, A. Rudi, I. Goldberg, M. Aknin and Y. Kashman, Saldedines A and B, Dibromo Proaporphine Alkaloids from a Madagascan Tunicate, J. Nat. Prod., 2009, 72, 784–786 CrossRef CAS PubMed
;
(b) K. C. Nicolaou, D. J. Edmonds, A. Li and G. S. Tria, Asymmetric Total Syntheses of Platensimycin, Angew. Chem., Int. Ed., 2007, 46, 3942–3945 CrossRef CAS PubMed
;
(c) S. P. Roche and J. A. Porco, Dearomatization Strategies in the Synthesis of Complex Natural Products, Angew. Chem., Int. Ed., 2011, 50, 4068–4093 CrossRef CAS PubMed
.
-
(a) A. Citterio, R. Sebastiano, A. Maronati, R. Santi and F. Bergamini, 1,5 vs. 1,6 Intramolecular Homolytic Aromatic Substitution by Vinyl Radicals, J. Chem. Soc., Chem. Commun., 1994, 1517–1518 RSC
;
(b) W. Dong, Y. Yuan, X. Gao, M. Keranmu, W. Li, X. Xie and Z. Zhang, Visible-Light-Induced Intermolecular Dearomative Cyclization of 2-Bromo-1,3-dicarbonyl Compounds and Alkynes: Synthesis of Spiro[4.5]deca-1,6,9-trien-8-ones, Org. Lett., 2018, 20, 5762–5765 CrossRef CAS PubMed
;
(c) W. Dong, Y. Yuan, X. Xie and Z. Zhang, Visible-Light-Driven Dearomatization Reaction toward the Formation of Spiro[4.5]deca-1,6,9-trien-8-ones, Org. Lett., 2020, 22, 528–532 CrossRef CAS PubMed
.
- L. Li, Z.-W. Hou, P. Li and L. Wang, Electrochemical Dearomatizing Spirocyclization of Alkynes with Dimethyl 2-Benzylmalonates to Spiro[4.5]deca-trienones, J. Org. Chem., 2022, 87, 8697–8708 CrossRef CAS PubMed
.
- Z. Guan, S. Zhu, Y. Ye, X. Li, Y. Liu, P. Wang, H. Zhang, Z. Huang and A. Lei, Synthesis of Cyclopentene Derivatives via Electrochemically Induced Intermolecular Selective (3 + 2) Annulation, Angew. Chem., Int. Ed., 2022, 61, e202207059 CAS
.
- J. Hu, L. Zeng, J. Hu, Z. Xu, R. Ma, X. Liu, Y. Jiao, H. He, S. Chen, Z. Xu, H. Wang and A. Lei, Electrochemical Difunctionalization of Terminal Alkynes: Access to 1,4-Dicarbonyl Compounds, Org. Lett., 2021, 24, 289–292 CrossRef PubMed
.
- Z.-W. Hou, L. Li and L. Wang, Regio- and Stereo-Selective Electrochemical Selenoalkylation of Alkynes with 1,3-Dicarbonyl Compounds and Diselenides, Org. Chem. Front., 2022, 9, 2815–2820 RSC
.
- L. Zhu, Y. Guo, B. Zu, J. Ke and C. He, Electrochemical α-Thiolation and Azidation of 1,3-Dicarbonyls, Chem. Commun., 2022, 58, 2758–2761 RSC
.
- P. Lu, W. Zhuang, L. Lu, A. Liu, Y. Chen, C. Wu, X. Zhang and Q. Huang, Chemodivergent Synthesis of Indeno[1,2-b]indoles and Isoindolo[2,1-a]indoles via Mn(III)-Mediated or Electrochemical Intramolecular Radical Cross-Dehydrogenative Coupling, J. Org. Chem., 2022, 87, 10967–10981 CrossRef CAS PubMed
.
- M. Xiong, X. Liang, Y. Zhou and Y. Pan, Synthesis of Polysubstituted Pyrroles through Electro-Oxidative Annulation of 1,3-Dicarbonyl Compounds and Primary Amines, J. Org. Chem., 2021, 86, 4986–4993 CrossRef CAS PubMed
.
- S. Sharma, S. Shaheeda, K. Shaw, A. Bisai and A. Paul, Two-Electron- and One-Electron-Transfer Pathways for TEMPO-Catalyzed Greener Electrochemical Dimerization of 3-Substituted-2-Oxindoles, ACS Catal., 2023, 13, 2118–2134 CrossRef CAS
.
- S. Sharma, A. Roy, K. Shaw, A. Bisai and A. Paul, Electrochemical Synthesis of Dimeric 2-Oxindole Sharing Vicinal Quaternary Centers Employing Proton-Coupled Electron Transfer, J. Org. Chem., 2020, 85, 14926–14936 CrossRef CAS PubMed
.
- K. Shaw, S. Sharma, A. Khatua, A. Paul and A. Bisai, Oxidative Electro-Organic Synthesis of Dimeric Hexahydropyrrolo-[2,3-b]indole Alkaloids Involving PCET: Total Synthesis of (±)-Folicanthine, Org. Biomol. Chem., 2021, 19, 9390–9395 RSC
.
|
This journal is © the Partner Organisations 2023 |