DOI:
10.1039/D3QM00727H
(Review Article)
Mater. Chem. Front., 2024,
8, 210-227
Magnetic hybrid transition metal halides
Received
29th June 2023
, Accepted 23rd September 2023
First published on 25th September 2023
Abstract
Metal halide semiconductors (MHS) have gained significant attention in recent years due to their exceptional optoelectronic properties. While most MHS systems that are currently under investigation are nonmagnetic, the diverse chemical and structural properties of MHS offer a unique opportunity to incorporate magnetic spins, such as transition-metal ions, into the metal halide lattice. This enables the exploration of novel magnetic phenomena and exchange mechanisms, making MHS a promising platform for multifunctional magnetic materials. In this review, we provide an overview of the historic and recent developments in magnetic transition metal halides (TMHs) with different chemical and structural diversities, highlighting the structure–magnetic property relationships in terms of different structure dimensions and metal ions. We also discuss the potential for multifunctional magnetic TMHs, where magnetism co-exists or is coupled with a secondary property of interest, such as conductivity, ferroelectricity, optical absorption, and luminescence. These coupling effects enable the TMHs to be promising candidates for multifunctional materials.
1. Introduction
Metal halide semiconductors (MHS) that adopt the perovskite crystal structure (AMX3, A = monovalent organic or inorganic cation, M = divalent metal, X = halide) have emerged as a revolutionary solution-processable semiconductor system because of their excellent optical and electronic properties as demonstrated in solar cells and light-emitting devices.1–3 Current studies focus mainly on group IV metal halides (e.g., M = Pb2+, Sn2+, Ge2+), which are, however, non–magnetic systems. Unlike transition-metal oxides whose cooperative magnetism has been intensively studied for decades in various crystal structures (e.g., spinel, garnet, and perovskite),4,5 hybrid transition-metal halides that contain magnetic spins remain relatively unexplored. These materials possess rich chemical and structural diversity and thus offer a great opportunity for the search of new magnetic materials and novel magnetic phenomena.6–8
The framework formed by metal halide octahedra (MX6) is normally stabilized by another inorganic metal cation or a library of organic cations, forming inorganic or organic–inorganic hybrid MHS. MX6 octahedra can be connected by single or multiple octahedral connectivity mode(s) (i.e., corner-, edge-, and face-sharing) in these metal halide materials forming a three-dimensional (3D) structure or condensate into two-dimensional (2D), one-dimensional (1D), or cluster (0D) networks. 3D metal halide perovskites or double-perovskites can be stabilized within the constraints of the Goldschmidt tolerance factor while lower dimensional structures can be formed with larger organic/inorganic cations. This versatile structural platform not only provides tunability for electronic properties, but also modulates magnetic properties through geometry, bond distance, and bond angles.
Research on the magnetic properties of transition metal halides (TMHs) can be traced back to the 1960s by de Jongh and Drumheller, when a number of hybrid 2D TMHs were studied and the structure–magnetic property relationships were revealed.9,10 Their unique 2D lattice with tunable magnetic anisotropy attracts a lot of experimental interest to examine the theory of the 2D magnetic-ordering model, namely, the Heisenberg, Ising, or X–Y model.11 A recent study on the monolayer transition metal halides has shown their potential in modulating spin properties.12–14 Meanwhile, renewed interest in these transition-metal halides has emerged along with the rise of MHS (extensive reviews on the MHS can be seen in ref. 1–3). New crystal structures with interesting magnetic properties including spin frustration,15 magnetic anisotropy,16 hybrid magnonic,17 and chiral ferromagnetic18 were reported.
Compared to traditional magnetic semiconductors or metals, the main advantages of these hybrid TMHs are their structural diversity and solution processability. There is a large opportunity to modulate their structure and magnetic interactions, and solution processability provides convenience for studying the magneto-optical properties and fabricating devices. Moreover, TMHs exhibit excellent semiconductor properties, such as a high absorption coefficient, tunable bandgap, and high carrier mobility. It makes them an ideal platform to explore the coupling effects between the magnetism and optoelectronic properties, giving rise to multi-functional materials, where control of light and charge carriers is coupled with magnetic spins.19 These materials show potential applications in spintronic devices, information storage, and quantum technology. Herein, we summarize the research progress of magnetic hybrid metal halides, highlighting the structure–magnetic property relationships.
2. Structure diversity and synthetic methods of TMHs
In a typical perovskite (AMX3) structure, metal halide octahedra are corner-shared forming a 3D framework, with the A-site cations occupying voids within the cage. Similar to the well-studied hybrid MHS based on group IV metal ions, TMHs display a large chemical and structural diversity. Depending on the manner of the inorganic octahedral linkage, TMHs display 3D, 2D, 1D, and 0D crystal structures (Fig. 1). Typical 3D TMHs are limited to metal fluorides, such as KMIIF3 (M = Mn2+, Fe2+, Co2+, Ni2+, Cu2+),20 with the AMX3 perovskite structures. Other 3D transition-metal halides are rare, not because of the constraint from the Tolerance factor, but due to the limitation of the octahedral factor (cation to anion ratio, μoct > 0.414)21 as transition metals normally have a smaller ionic radius. In the 3D framework, the perovskite structure can be doubled to form a cation-ordered double perovskite structure, A2MIMIIIX6, where monovalent MI or trivalent MIII are transition metal ions. Another category of 3D TMHs is the dilute magnetic perovskites, which are composed of diamagnetic perovskite doped with transition metal ions (Section 5.2), such as MA(Pb:Mn)I3 (MA+ = methylammonium).19
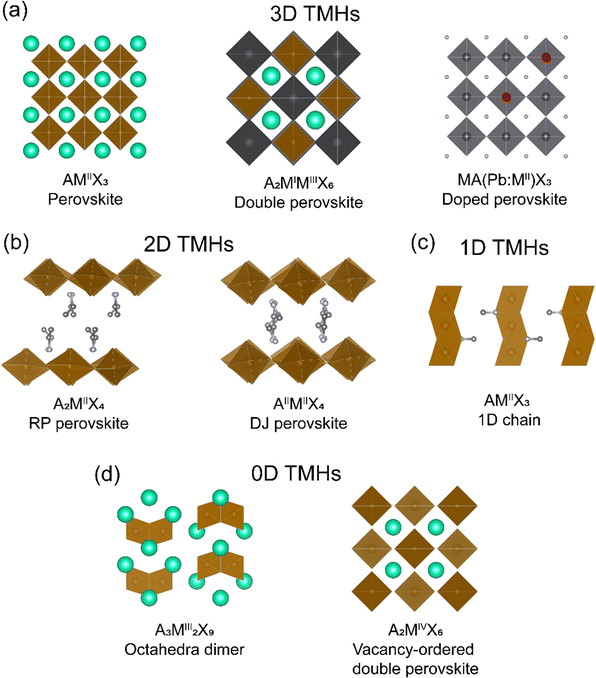 |
| Fig. 1 Different crystal structures of TMHs. (a) 3D TMHs, (b) 2D TMHs, (c) 1D TMHs, and (d) 0D TMHs. | |
2D structures can be derived by sliding the 3D perovskite structures from different crystallographic orientations, including (100), (110), and (111) orientations, with the general formula of AI2MIIX4, AIIMIIX4, and AI4MIIMIII2X12 (where A is a monovalent or divalent organic cation), respectively. The space between the inorganic layers is then filled by the organic cations through electrostatic interactions. The (100) orientated 2D perovskites can be divided into a Ruddlesden–Popper (RP) phase and a Dion–Jacobson (DJ) phase. When incorporated with the monoammonium cation, the structure will be staggered in the RP phase with the formula A2MIIX4, such as EA2CuCl4 (EA = ethylammonium).22 The diammonium cation will lead to the eclipsed DJ phase with the formula of AIIMIIX4, such as EDACuCl4 (EDA = ethylenediammonium).23 (100) orientated structures are the most common among the 2D TMHs. The various structure anisotropy in 2D perovskites will affect the related magnetic properties, giving rise to magnetic anisotropy.
In 1D hybrid TMHs, such as MANiCl3,24 the inorganic 1D chains are formed by face-sharing octahedra, charge compensated by organic spacers. In 0D hybrid TMHs, inorganic metal halide units are isolated, either as individual octahedron or dimers. For example, Cs3Fe2Br9 are composed of face-sharing [Fe2Br9]3− octahedral dimers.25 Another interesting 0D TMHs system is the vacancy-ordered double perovskite with the formula of A2MIVX6, in which the octahedra are isolated. As the magnetic property is closely related to the crystal structure, below we will discuss the magnetic exchange mechanism, magnetic property, and structure–property relationships of hybrid transition-metal halides based on their crystal structures.
The solution-processable synthetic methods of TMHs show obvious advantages compared to traditional semiconductors, i.e., convenient implementation and free of harsh reaction conditions. To grow the single crystals of TMHs, the two most commonly used techniques are the cooling method and the antisolvent method. In the cooling method, the metal salts and organic ammonium salts are dissolved under heating, to form a supersaturated solution. With the temperature slowly cooling to room temperature, crystals gradually precipitated. By slowly diffusing antisolvent into the precursor solution, the antisolvent method enables the preparation of TMHs single crystals at room temperature. To prepare polycrystalline thin films, spin coating is the most used technique, which allows for fast and convenient operations but is only suitable for substrates with small areas. Polycrystalline thin films are the main format for the study of magneto-optical properties and the fabrication of related devices.26,27
3. Magnetic exchange mechanism of TMHs
Any transition metal ions with unpaired electrons will exhibit paramagnetism, in which the spins tend to align in the direction of the external magnetic field. Below the critical temperature, the spin fluctuation induced by thermal energy is removed, and long-range magnetic order will emerge in the magnetically correlated systems, which includes ferromagnetism and antiferromagnetism. In ferromagnetic (FM) materials, the spin will align parallelly below the ordering temperature, known as the Curie temperature (Tc). In antiferromagnetic materials, the spins will align antiparallelly below the Néel temperature (TN). In the structures of TMHs, the transition metal ions are bridged through the halide ions in single or multiple M–X–M linkages depending on the connectivity of inorganic building blocks. Therefore, the magnetic order in TMHs is generally realized through the M–X–M superexchange path, which can be understood by the Goodenough–Kanamori rules.28
For those symmetric MX6 octahedra (e.g., Mn2+, Fe2+) without Jahn–Teller distortion (Fig. 2a), the nearest-neighbor metal ions exchange via the same d orbital (e.g., dx2−y2), and there will be two situations.29,30 When the M–X–M bond angle is 180°, the linear M–X–M superexchange path will result in the AFM order according to the Pauli exclusion principle. When the M–X–M bond angle approaches 90°, both the px and py orbitals in the halide ions participate in the superexchange path. The spin alignment on the two orthogonal 2p orbitals ensures the maximum spin multiplicity, therefore leading to a ferromagnetic exchange between the nearest-neighbor metal ions. For the Jahn–Teller distorted MX6 octahedra (Cr2+, Cu2+), the neighboring octahedra are arranged in the antiferrodistortive structure.31–33 For the d4 Cr2+ ions, two bonds will be elongated along the z direction in the octahedra, lowering the energy level of dz2. In the distorted layered octahedral plane, the elongated Cr–X bond will be located orthogonally in the nearest octahedra (Fig. 2b). Therefore, the two dz2 orbitals on the neighboring octahedra will be orthogonal, leading to a zero-integral of the orbitals and FM superexchange interaction.34–36 In the Jahn–Teller distorted Cu2+ octahedra, the one unpaired electron is located on the dx2−y2 orbital, resulting in the dx2−y2–dz2 FM superexchange path.37–41 The exchange energy between magnetic spins can be described by the Heisenberg–Dirac–Van Vleck (HDVV) Hamiltonian42 (1).
| 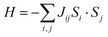 | (1) |
Si is the spin operator, and
Jij is the exchange constant between the magnetic ions at sites
i and
j. The value of exchange constant
Jij suggests the coupling strength between the magnetic ions, which is positive for FM exchange and negative for AFM exchange.
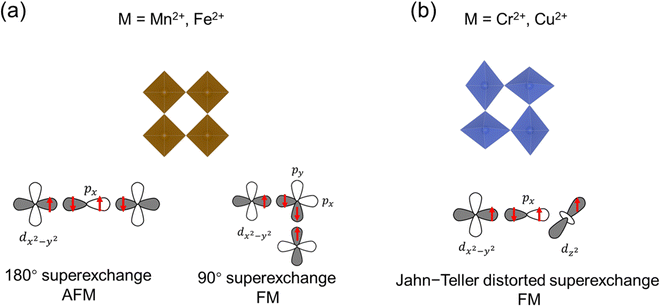 |
| Fig. 2 Illustration of the superexchange mechanism in Mn2+/Fe2+ systems (a) and Jahn–Teller distorted Cr2+/Cu2+ systems (b). | |
To characterize the magnetic properties of TMHs, temperature-dependent magnetization measurement is usually conducted, where the material is cooled with (field cooled, FC) or without (zero field cooled, ZFC) an external magnetic field. The bifurcation between ZFC and FC is induced by the thermal barrier in domain reorientation. Based on the mean field theory, the magnetic susceptibility (χ) of TMHs obeys the Curie–Weiss law (2) above the ordering temperature.
| 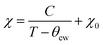 | (2) |
C is the Curie constant,
χ0 is the diamagnetic component, and
θcw is the Curie–Weiss temperature that indicates the magnetic correlation. Ferromagnetic materials will have a positive
θcw value, while antiferromagnets have a negative
θcw value. As the paramagnet transforms to a ferromagnet below
Tc, the spins align parallelly spontaneously, inducing a significant increase in the magnetic moment. For an antiferromagnet, the magnetization will show a maximum at Néel temperature. For detailed methodologies to analyze the experimental magnetic data refer to the protocol from Seshadri's group.
43
4. Magnetic properties of different TMHs
The magnetic properties of TMHs are strongly related to the crystal structures. In 2D structures, the interlayer structure anisotropy gives rise to magnetic anisotropy. With the structure dimension lowered to 1D and 0D, the magnetic coupling between metal ions will be weakened. Below, we will discuss the magnetic properties of TMHs in terms of different dimensions and different transition metal ions.
4.1 3D magnetic TMHs
Typical 3D magnetic TMHs are in the 3D perovskite structure, such as the series of KMF3 (M = Mn2+, Fe2+, Co2+, Ni2+, Cu2+)17 and KMnCl3,44 which are strong antiferromagnets with high Néel temperatures from 88 to 275 K (Table 1). In the cubic structure of KMF3 (M = Mn2+, Fe2+, Co2+, Ni2+), TN is higher with higher d electron metal ions, which might be induced by the enhanced orbital overlap. Another 3D magnetic MHS that has been found recently is the CsEuX3 (X = Cl−, Br−).45 These compounds order antiferromagnetically with a Néel temperature of ∼2 K, originating from the nearly 180° bond angle of Eu–X–Eu. The contracted 4f-orbitals of the lanthanide centers result in weaker magnetic exchange interactions compared to 3d metal ions.46 Interestingly, nanocrystals and thermally evaporated thin films of CsEuCl3 were reported to be ferromagnetic with Curie temperatures of 2.5–3.0 K, which indicates that the type and strength of magnetic coupling can be tuned from antiferromagnetic to ferromagnetic through quantum confinement or substrate film interactions.
Table 1 Néel temperatures of several 3D TMHs
Compounds |
T
N (K) |
KMnCl3 |
98 |
KMnF3 |
88 |
KFeF3 |
113 |
KCoF3 |
114 |
KNiF3 |
275 |
KCuF3 |
243 |
Cs2AgFeCl6 |
18 |
Cs2NaFeCl6 |
3 |
CsEuX3 (X = Cl, Br) |
2 |
We have studied the magnetic properties of 3D Cs2AgFeCl6 and Cs2NaFeCl6 double perovskites, revealing their geometrically frustrated AFM systems.15 The Néel temperatures of these double perovskites are much lower than that of the typical 3D perovskite KFeF3, as the magnetic ions (Fe3+) are not directly connected, but are interconnected through a diamagnetic ion (Na+ or Ag+), resulting in weaker superexchange interactions. A recent study on perovskites with trivalent lanthanide, the Cs2NaLnI6 (Ln = Ce3+, Nd3+, Gd3+, Tb3+, Dy3+) series,47 reveals the weakened coupling between magnetic ions due to the long Ln–Ln distance and contracted f orbitals.
4.2 2D magnetic TMHs
4.2.1 2D Hybrid manganese (d5) halides.
The study of 2D layered Mn2+ halides started in 1970. Epstein et al. revealed that Rb2MnCl4 and Cs2MnCl4 order antiferromagnetically with two-dimensional spin correlations.48 A later study of MA2MnCl4 by van Amstel et al. found magnetic anisotropy in this 2D antiferromagnet.29 This indicates that the octahedral tilting and the anisotropic layered structure can lead to the spin canting phenomenon in 2D Mn2+ halides, in which spins are tilted by a small angle about their axis rather than being co-parallel.
Recently, the García group systematically studied the spin canting phenomenon in a series of 2D Mn2+ halides.16 PEA2MnCl4 (PEA = (C6H5CH2CH2NH3)+) and EA2MnCl4 crystallize in staggered RP phases (Fig. 3(a) and (b)), and EDAMnCl4 belongs to the DJ phase (Fig. 3(c)). In PEA2MnCl4, the out-of-plane temperature dependent magnetization curve, M(T), shows a typical AFM order. The steep increase of magnetic moment in the in-plane direction indicates a weak FM component in the [MnCl6] octahedral plane (Fig. 3(d)), which suggests that the out-of-plane direction is the easy axis of the AFM order. This is consistent with the isothermal magnetization curve in these two directions (Fig. 3(e)). The weak FM component in the in-plane direction is attributed to the Dzyaloshinskii–Moriya interaction, which refers to the antisymmetric magnetic exchange in a system that lacks inversion symmetry. In EA2MnCl4, the magnetic order along the out-of-plane direction is much more complex, exhibiting a series of metamagnetism transitions, i.e., AFM → AFM with spin-canting → AFM with FM contributions (Fig. 3(f) and (g)). When looking at the DJ phase of EDAMnCl4, the magnetic properties are different from the RP structures. Both the in-plane and out-of-plane M(T) curves show a typical AFM order (Fig. 3(h)) and are absent from spin canting, indicating a much smaller magnetic anisotropy in DJ structures compared to RP structures.
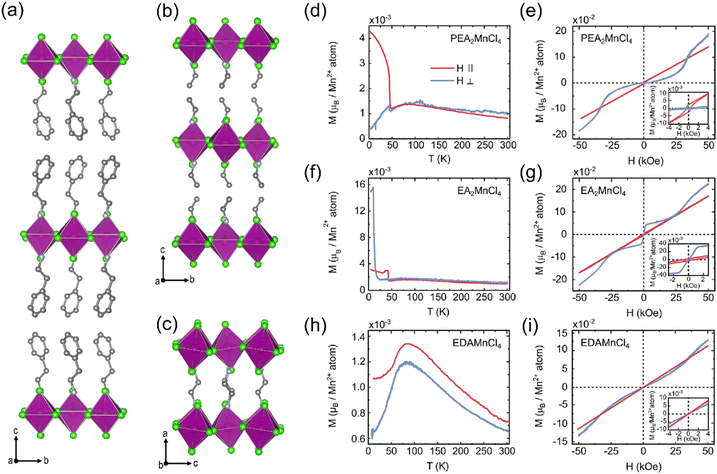 |
| Fig. 3 Crystal structure of PEA2MnCl4 (a), EA2MnCl4 (b), and EDAMnCl4 (c). Temperature (T) dependent magnetization (M) at 500 Oe parallel (‖, red lines) and perpendicular (⊥, blue lines) to the MnCl6 octahedral layers for PEA2MnCl4 (d), EA2MnCl4 (f), and EDAMnCl4 (h). Field (H) dependent magnetization (M) at 5 K parallel and perpendicular to the MnCl6 octahedral layers for PEA2MnCl4 (e), EA2MnCl4 (g), and EDAMnCl4 (i). The insets show the zoom-in of the M(H) curves at low magnetic fields. Reproduced from ref. 16 with permission from Wiley-VCH, Copyright 2022. | |
The origin of the spin-canting in Mn2+ halides is the antisymmetric Dzyaloshinskii–Moriya interaction, which is related to both the octahedral tilting and the interlayer anisotropy. In the RP structure, the layer of [MnCl6] octahedra is staggered with the adjacent layer, which results in anisotropy in the out-of-plane direction. While in DJ structures, the inorganic layers are vertically aligned. Both the structure symmetry and shorter interlayer distance are responsible for the absence of spin canting in the DJ structure. Other layered Mn2+ halides with RP structures also show spin canting behavior with different canting angles, as summarized in Table 2. This structure–property relationship provides us with a strategy to modulate the magnetic anisotropy in layered manganese halides by changing the organic cations.
Table 2 Magnetic properties of different layered manganese halides
Compound |
Néel temperature TN (K) |
J/KB (K) |
Curie–Weiss temperature θCW (K) |
Spin canting degree (°) |
Ref. |
PA = C6H5NH3+, PMA = C6H5CH2NH3+, PPA = C6H5CH2CH2CH2NH3+. ‖ (parallel) and ⊥ (perpendicular) is the direction of magnetic field to the MnCl6 octahedral layers. |
PEA2MnCl4 |
45 |
−9.9 |
(‖) −173 |
0.06 |
16, 49, 50
|
(⊥) −449 |
EA2MnCl4 |
43 |
−8.8 |
(‖) −195 |
0.03 |
16
|
(⊥) −256 |
EDAMnCl4 |
80 |
−10.2 |
(‖) −190 |
|
16
|
(⊥) −180 |
PA2MnCl4 |
42.6 |
|
−126 |
0.036 |
51
|
PMA2MnCl4 |
44.6 |
|
−135 |
|
51
|
PPA2MnCl4 |
41.8 |
|
−148 |
0.029 |
51
|
MA2MnCl4 |
44 |
|
−23 |
|
29,52
|
4.2.2 2D Hybrid iron (d6) halides.
Layered Fe2+ halides show similar magnetic properties as 2D Mn2+ halides, where AFM interaction plays a dominant role. Kurmoo's group studied several RP-type layered Fe2+ compounds, MA2FeCl4, EA2FeCl4 and PEA2FeCl4 (Table 3).53–55 MA2FeCl4 only exhibits antiferromagnetism with hidden canting and a low metamagnetic critical field of 200 Oe. By changing the organic cation to C2H5NH3+, the extra CH2 introduces more degrees of freedom in EA2FeCl4 compared to MA2FeCl4 (Fig. 4a), giving rise to a series of temperature-dependent phase transitions and ferroelectric and ferroelastic properties. EA2FeCl4 also shows canted antiferromagnetism along the c axis with a spin canting angle of 0.64° (Fig. 4(b) and (c)). PEA2FeCl4 is more stable than the former two compounds, benefiting from the hydrophobic 2-phenylethyl ammonium cation, (C6H5C2H4NH3)+. It exhibits canted antiferromagnetism along the a axis with an obvious hysteresis loop (Fig. 4(d) and (e)). Due to the ferroelastic structure in PEA2FeCl4, the temperature and mechanical stress induced structural transition between single- and multi-ferroelastic domain states can effectively modulate the magnetic properties. Uniaxial stress can even switch the axes in the crystal. The hysteresis loop along the a axis in the virgin sample will vanish after applying stress along the b axis (Fig. 4(e)).
Table 3 Magnetic properties of different layered iron halides
Compound |
Néel temperature TN (K) |
Curie–Weiss temperature θCW (K) |
Spin canting degree (°) |
Ref. |
PEA2FeCl4 |
98 |
−298 |
0.53 |
55
|
EA2FeCl4 |
99 |
H ‖ a or b, −300 K |
|
H ‖ c, −530 K |
0.64 |
54
|
MA2FeCl4 |
95 |
H ‖ a or b, −250 K |
|
H ‖ c, −330 K |
1.4 |
53
|
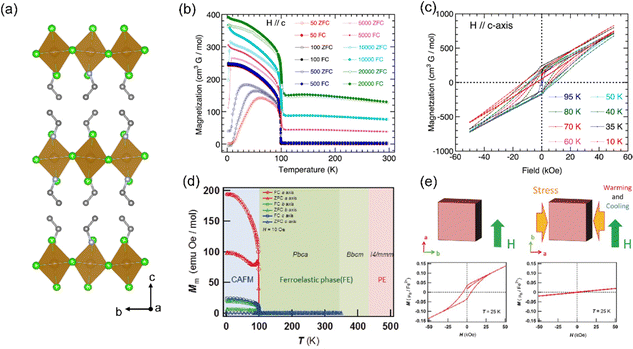 |
| Fig. 4 (a) Crystal structure of EA2FeCl4. (b) Temperature dependence of the zero-field-cool and field-cool magnetizations of EA2FeCl4 in different applied fields H ‖ c-axis. (c) Isothermal magnetization at different temperatures for EA2FeCl4 after zero-field-cooling with H ‖ c-axis. (b) and (c) are reproduced from ref. 54 with permission from American Chemical Society, Copyright 2015. (d) Overlay of the crystal phase regions with the temperature dependence of the magnetization of PEA2FeCl4 (H = 10 Oe). (e) Isothermal magnetizations of a PEA2FeCl4 single crystal for the same orientation at 25 K; (left) the virgin sample and (right) the sample after applying stress along the b-axis. (d) and (e) are reproduced from ref. 55 with permission from Wiley-VCH, Copyright 2017. | |
This study reveals that the antisymmetric Dzyaloshinskii–Moriya interaction is coupled to ferroelasticity in PEA2FeCl4. Furthermore, the designed combination of functional organic molecules and transition metal ions can make molecule-based multiferroics, which are expected to realize the coupling of ferroelectric, ferroelastic, and AFM/FM properties within one material.
4.2.3 2D Hybrid copper (d9) halides.
Unlike the Mn2+ and Fe2+ MHS, the Jahn–Teller distortion of [CuX6] octahedra gives rise to intralayer ferromagnetism in 2D hybrid Cu2+ halide perovskites with a Curie temperature in the range of 4–16 K. The intralayer FM exchange can be modulated with the halide composition, with Br showing larger FM interactions than Cl due to higher covalency (Fig. 5a). Organic cations show a weak impact on the intralayer FM interaction (Fig. 5a). However, the interlayer magnetic exchange interaction is quite different from the intralayer FM interaction, which generally gives a weak AFM in the out-of-plane direction.9,10,33 Interestingly, the interlayer magnetic interaction can be modulated by changing the interlayer distance and halide-halide distance.33,56 A systematic study of interlayer and intralayer interactions in the alkylammonium Cu2+ metal halides (CnH2n+1NH3)2CuX4 (X = Cl, Br, n = 1–10) was done by de Jongh et al., and is now summarized in Fig. 5(a) and (b).57–60 Due to the much longer interlayer distance compared to the Cu–X bonds, the interlayer exchange constant J′ is significantly smaller than the intralayer exchange constant J (Fig. 5b). The Br compounds show a larger absolute value of J′ compared to Cl compounds because of the larger orbital radius of Br ions, which enhances the interlayer interaction.38 In the staggered RP structures of (CnH2n+1NH3)2CuX4, the interlayer interaction is reduced due to the offset between the inorganic layers and the larger interlayer distance. The interlayer distance is increased with longer organic cations, leading to a reduced J′ with a larger n value. The n = 1 (X = Cl) and n = 2 (X = Br) are two exceptions to this trend. (CH3NH3)2CuCl4 even shows a positive value of J′, indicating an FM interaction in the out-of-plane direction.61 The exact mechanism remains to be further understood.
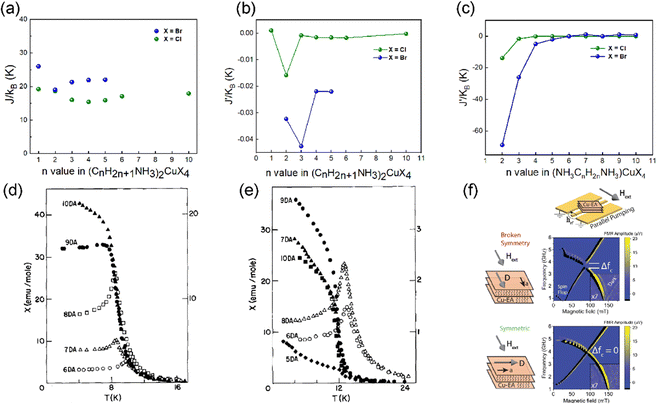 |
| Fig. 5 (a) The plot of J versus the n value in the RP structures of (CnH2n+1NH3)2CuX4 (X = Cl, Br). (b) The plot of J′ versus the n value in the RP structures of (CnH2n+1NH3)2CuX4 (X = Cl, Br). (c) The plot of J′ versus the n value in the DJ structures of (NH3(CH2)nNH3)CuBr4 (X = Cl, Br). J′ is the interlayer interaction. J is the intralayer interaction. (d) Powder magnetic susceptibility versus temperature data for (NH3(CH2)nNH3)CuCl4 with n = 6–10. (e) Powder magnetic susceptibility versus temperature data for (NH3(CH2)nNH3)CuBr4 with n = 5–10.62 Solid data points are referenced to the left scale and open data points to the right scale. (d) and (e) are reproduced from ref. 62 with permission from Elsevier, Copyright 1984. (f) Antiferromagnetic resonance in (CH3CH2NH3)2CuCl4 single crystals with a strong magnon–magnon coupling. (f) is reproduced from ref. 17 with permission from Nature Springer, Copyright 2023. | |
When incorporating the divalent alkyldiammonium cations, the structure of layered Cu2+ halides transforms into the eclipsed DJ phase. The magnetic properties of (NH3(CH2)nNH3)CuX4 (X = Cl, Br) were studied by Rubenacker et al., and are summarized in Fig. 5(c).62–67 As the inorganic layers are arranged vertically in the out-of-plane direction, the X–X halide distance is shorter and the absolute value of J′ is larger compared to the RP structure (Fig. 5c). Similar to the RP structure, the interlayer exchange interactions decay rapidly with the increasing length of organic spacers. In (NH3(CH2)2NH3)CuCl4, the interlayer interaction is even stronger than the intralayer interaction, resulting in an overall AFM order.68 The interlayer interaction J′ is also decreased as the interlayer distance increases, leading to an overall FM order in n = 10 (Fig. 5(d)). More interestingly, in the series of (NH3(CH2)nNH3)CuBr4, an unexpected alternation of J′ is observed. J′ is positive when n = 7, 9, and 10 and negative when n = 6 and 8 (Fig. 5(e)), which might be related to the structural geometry of the Cu–X⋯X–Cu linkage under layered structures.62 These results indicate that the magnetic order and anisotropic exchange interactions in layered Cu2+ halides can be effectively modulated by changing the halides and organic spacers. To raise the Curie temperatures of copper halides, which is crucial for the development of spintronics, it's necessary to enhance the intralayer FM coupling and weaken the interlayer AFM coupling. Therefore, Br/I halides and long organic spacers are promising for making 2D copper halides with high Curie temperatures.
We have further studied the Dzyaloshinskii–Moriya interaction in layered AFM (CH3CH2NH3)2CuCl4 through ferromagnetic resonance measurements.17 This interaction can control the magnetic dynamics particularly in AFM materials, such as collective spin excitations (magnons). The mixed magnon in this AFM material has a simple planar anisotropy. In principle, due to the opposite parity, two orthogonal magnon modes exist independently and cannot interact with each other, allowing for a degeneracy to occur. However, due to the presence of Dzyaloshinskii–Moriya interaction, the symmetry of even parity high-frequency optical magnon (under a twofold rotation) and odd parity low-frequency acoustic magnon is broken (Fig. 5(f)). These two magnon modes couple, lifting the degeneracy and forming new hybrid states. This coupling between the optical and acoustic magnons allows for the spin information transfer between these two quasi-particles.
Recently, Zhang et al. reported two new layered Cu2+ halides with aromatic organic spacers, (PED)CuCl4 (PED = N-phenylethylenediammonium) and (BED)2CuCl6 and (BED = N-benzylethylenediammonium), where the former one features a (110) structure and the latter a (100) structure (Fig. 6(a) and (b)).69 The Curie temperatures of these two compounds are 12 K (Fig. 6(a) and (d)). The value of saturated magnetization in (PED)CuCl4 is much smaller than that in (BED)2CuCl6 (Fig. 6(e) and (f)), which is ascribed to a more distorted octahedra in (PED)CuCl4 ((110) structure) than that in (BED)2CuCl6. More distorted octahedra and corrugated [CuCl6] layers suppress the superexchange interaction between Cu2+ ions in (PED)CuCl4, leading to weaker ferromagnetism. Similar to (PEA)2FeCl4, (PEA)2CuCl4 also exhibits multiferroic behavior involving the coexistence of ferroelectricity induced by the organic spacers and ferromagnetism induced by the corner-shared [CuCl6] octahedral layer, giving rise to large magnetoelectric coupling.70
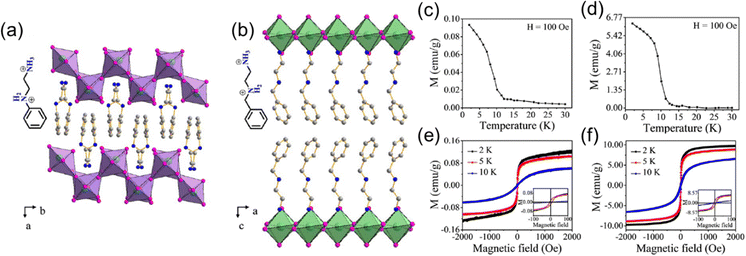 |
| Fig. 6 Crystal structures of (PED)CuCl4 (a), and (BED)2CuCl6 (b). (c) and (d) Temperature-dependent magnetization of (PED)CuCl4, (BED)2CuCl6 under the field of 100 Oe. (e) and (f) M–H curves of (PED)CuCl4 and (BED)2CuCl6 at 2 K, 5 K, and 10 K. Reproduced from ref. 69 with permission from Wiley-VCH, Copyright 2019. | |
4.2.4 2D Hybrid chromium (d4) halides.
2D layered Cr2+ halides show a similar crystal structure to layered hybrid Cu2+ halides with a significant Jahn–Teller distortion. The high-spin configuration (d4) of the Cr2+ ion results in strong FM coupling and relatively high Curie temperature compared to the copper compounds.71–73 A series of layered Cr2+ halides (CnH2n+1NH3)2CrCl4 was studied by Day et al. in 1986, showing an FM order with a Curie temperature in the range of 37–52 K (Table 4).74–76 (C6H5CH2NH3)2CrBr4 shows a relatively high Curie temperature of 52 K, owing to the larger interlayer distance.77 Recently, Xiong's group reported multifunctional Cr2+ halides (DFCBA)2CrCl4 (DFCBA = 3,3-difluorocyclobutylammonium), which show the co-existence of ferroelectricity (transition temp = 387 K) and ferromagnetism (Tc = 32.6 K) (Fig. 7(a) and (b)).78 At 2 K, [DFCBA]2CrCl4 shows a hysteresis loop with a coercive field (HC) of 85 Oe (Fig. 7(c)). The relatively high Curie temperature of Cr2+ halide perovskites combined with ferroelectricity is of great potential in multifunctional smart devices.
Table 4 Magnetic properties of layered chromium halides
Compound |
T
c/K |
(J/KB)/K |
θ
CW (K) |
d/Å |
Ref. |
(CH3NH3)2CrCl4 |
42 |
13.0 |
59 |
9.44 |
71
|
(C2H5NH3)2CrCl4 |
41 |
10.1 |
58 |
10.71 |
71
|
(C3H7NH3)2CrCl4 |
39.5 |
9.3 |
57 |
12.35 |
74
|
(C5H11NH3)2CrCl4 |
|
9.3 |
57 |
17.81 |
74
|
(C6H5CH2NH3)2CrCl4 |
37 |
10.6 |
58 |
15.71 |
77
|
(C6H5CH2NH3)2CrBr3.3Cl0.7 |
49 |
12.5 |
62 |
16.10 |
79
|
(C6H5CH2NH3)2CrBr4 |
52 |
13.1 |
77 |
16.24 |
77
|
(C6H5CH2NH3)2CrBr3.3I0.7 |
51 |
11.0 |
69 |
16.15 |
72
|
[DFCBA]2CrCl4 |
32.6 |
7.15 |
45 |
5.4 |
78
|
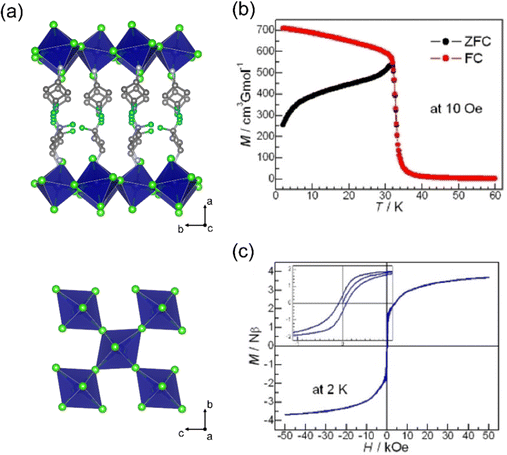 |
| Fig. 7 (a) Crystal structure of [DFCBA]2CrCl4. (b) The temperature dependent ZFC/FC plots at 10 Oe field. (c) The isothermal magnetization curve at 2 K, the inset is the low field region. Reproduced from ref. 78 with permission from Wiley-VCH, Copyright 2022. | |
4.2.5 2D Halide double perovskites.
Halide double perovskites provide a platform to incorporate transition metal ions and to tune the magnetic properties through composition modulation. The formula of typical 3D double perovskite is (A2MIMIIIX6). The combination of diamagnetic metal and transition metal ions (e.g. AgI/NaI–FeIII) will give an MIII–X–MI–X–MIII linkage, different from the traditional M–X–M superexchange pathway. We have studied the magnetic properties of a series of AgI/NaI–FeIII double perovskites, including 3D structures and their 2D analogues.15 As depicted in Fig. 8(a), three different lengths of organic ammoniums were inserted into the 3D perovskite, resulting in a 2D (100) structure. Magnetic measurements were then conducted on Ag–Fe double perovskites with three different organic cations (phenylethylammonium (PEA), R-(+)-β-methylphenethylammonium (R-MPA) and butanediammonium (BDA)). The impact of monovalent metal ions on magnetic properties is also examined in PEA4NaFeCl4 (Fig. 8(b) and (c)). In the Ag–Fe–Cl series, we found a linear relationship between the Curie–Weiss temperature and the nearest Fe–Fe distance represented by the red line in Fig. 8(c), indicating that the longer distance between Fe–Fe leads to weaker magnetic coupling. However, when replacing Ag+ with Na+, we observed that the antiferromagnetic coupling did not increase, despite the further decrease in Fe–Fe distance, suggesting that bridging metal is also involved in the magnetic exchange interaction. It further indicates that the antiferromagnetism comes from FeIII–Cl–MI–Cl–FeIII long-range superexchange rather than a direct magnetic exchange pathway. Compared to the geometrically frustrated 3D Cs2AgFeCl6 and Cs2NaFeCl6, the frustration factors of 2D analogues are dramatically reduced due to the square lattice. Furthermore, AFM coupling in the AgI–MoIII and Ag–RuIII layered double perovskites have been studied by different groups.80,81 The experiment results are consistent with the MIII–X–MI–X–MIII long-range superexchange pathway.
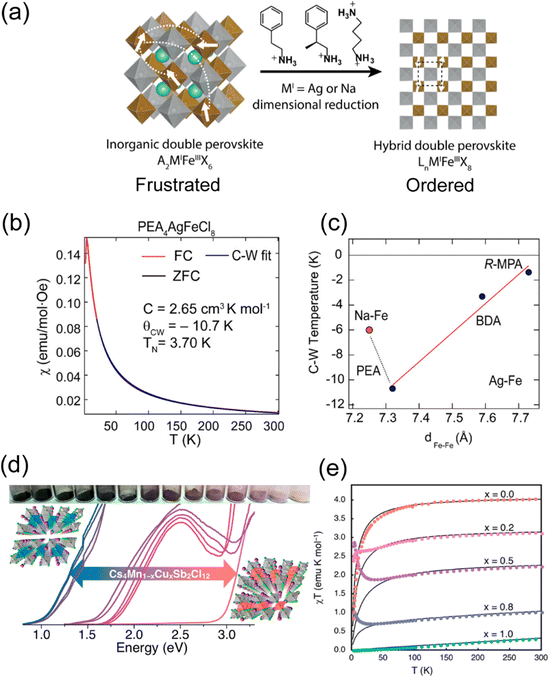 |
| Fig. 8 (a) Schematic illustration of the evolution from 3D double perovskite to 2D double perovskite by different organic amines. (b) Magnetic susceptibility vs. temperature for PEA4AgFeCl8. (c) Correspondence between Curie–Weiss temperature and nearest Fe–Fe distance in the hybrid layered Fe–Cl double-perovskite series. The orange dot represents PEA4NaFeCl8, and black dots represent the Ag–Fe–Cl series. The red line is the linear fit for the Ag–Fe–Cl series. (PEA = phenylethylammonium, R-MPA = R-(+)-β-methylphenethylammonium, BDA = butanediammonium). (a)–(c) are reproduced from ref. 15 with permission from the American Chemical Society, Copyright 2022. (d) Photographs of Cs4Mn1−xCuxSb2Cl12 (x = 0–1) and the corresponding absorption spectra. (e) Temperature dependence of the inverse magnetic susceptibility-temperature of Cs4Mn1−xCuxSb2Cl12, the solid lines are the best fit of the data. (d) and (e) Are reproduced from ref. 82 with permission from American Chemical Society, Copyright 2018. | |
Another series of layered double perovskite is the [111] oriented structure, which is achieved through the incorporation of vacancies rather than the large organic cations found in the [100] perovskite. In the [111] layered double perovskite, one vacancy and one bivalent metal ion replace two monovalent metal ions of [100] perovskites, resulting in a general formula of A4MIIM2IIIX12.83 Bivalent metals, such as CuII or MnII, often provide unpaired electrons, whereas trivalent metals are typically diamagnetic (e.g., SbIII or BiIII). In 2017, the first [111] layered double perovskite, Cs4CuSb2Cl12, was reported.84 Follow-up research on the Cs4Mn1−xCuxSb2Cl12 series (Fig. 8(d)) demonstrated that Mn alloying can modulate the antiferromagnetic behavior (Fig. 8(e)).82 As x values increase, the temperature-independent paramagnetism and the Pauli paramagnetism state gradually increase, originating from the rising concentrations of movable charge carriers. In addition, DFT calculation showed that intralayer antiferromagnetic behavior occurs within each [MCl6] (M = Cu2+, Mn2+) octahedral chain along the [010] direction.
4.3 1D and 0D Hybrid magnetic metal halides
In 1D and 0D TMHs, the magnetic coupling is expected to be weakened owing to the reduced connectivity. In the 1D structures, the [MX6] octahedra are usually connected in a face-sharing manner. Magnetic interactions can also be divided into two parts in 1D TMHs, namely, the intrachain interaction, and interchain interaction. Since the intrachain interaction plays a dominant role and is working through the superexchange via the bridging halides. The M–X–M angle will be crucial to determine the overall magnetic order.
Recently, the Cava group studied the magnetic properties of 1D ANiCl3 (A = MA+, Gu+, FA+, DMA+, TMA+) compounds with different organic spacers.85 These Ni halides crystallize in a hexagonal-perovskite-type structure. The [NiCl6] octahedra are face-sharing connected to form 1D chains, which are separated by organic cations. From the Curie–Weiss fitting, TMANiCl3 shows a positive Curie–Weiss temperature, while the other four compounds have negative Curie–Weiss temperatures. TMANiCl3 has the largest Ni–Cl–Ni angle of 78.75° among the above five compounds (Fig. 9). The intrachain ferromagnetic coupling increases as the Ni–Cl–Ni angle approaches 90°, which is consistent with the superexchange model.
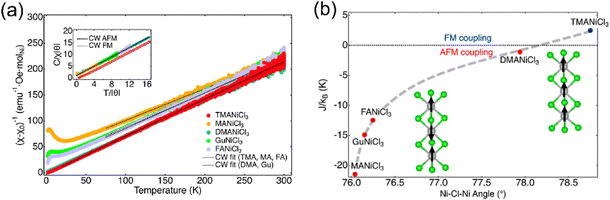 |
| Fig. 9 The structure (a) and magnetic properties (b) of the 1D ANiCl3 (A = MA+, Gu+, FA+, DMA+, TMA+) compound. TMA = (N(CH3)4+), MA = (CH3NH3+), DMA = ((CH3)2NH2+), Gu = (C(NH2)3+), and FA = (CH(NH2)2+). Reproduced from ref. 85 with permission from American Chemical Society, Copyright 2022. | |
In the 1D series of MACoCl3, FACoCl3, and GuCoCl3, the structure-magnetic property relationship is more complex.86 The one-dimensional intrachain coupling is antiferromagnetic (Fig. 10(a)). However, there is no direct relationship between the Co–Cl–Co angle and the corresponding intrachain coupling constant because the unpaired t2g electron in high-spin Co2+ contributes to the complexity of magnetic exchange within the system. Instead, there is a direct relationship between the interchain Co–Co distance and the critical temperature of the long-range magnetic order. With the organic cation changing from MA+ to FA+, and Gu+, the size of organics is larger, resulting in a longer interchain Co-Co distance and lower critical long-range ordering temperatures (Fig. 10(b)).
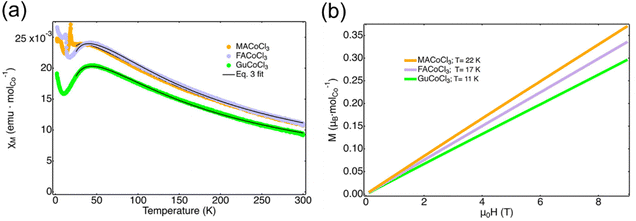 |
| Fig. 10 (a) Temperature-dependent magnetic susceptibility of MACoCl3, FACoCl3, and GuCoCl3. (b) Field-dependent magnetization of MACoCl3, FACoCl3, and GuCoCl3 at the critical long-range ordering temperature. Reproduced from ref. 86 with permission from American Chemical Society, Copyright 2023. | |
The magnetic properties of a series of low-dimensional Ru metal halides were studied by the Cheetham group.87 MA2RuCl6 crystallizes as a vacancy-ordered halide perovskite, and the [RuCl6]2− octahedra are isolated and undistorted (Fig. 11(a)). Their magnetic properties are expected to show isolated spin-ion behavior without the exchange interactions between the [RuCl6]2− octahedron. The theoretical value of meff can be predicted by the octahedral crystal field with spin–orbit coupling. The experimental data of MA2RuCl6 coincides well with the Kotani plot calculated from a spin–orbit coupling constant ξ of 1400 cm−1, which is consistent with the d4 Ru4+ ions (Fig. 11(b)). As the temperature decreases, the effective moment falls to the ground state J = 0, indicating the absence of coupling between the isolated [RuCl6]2− octahedra. MA2NaRuCl6 crystallizes in a 1D structure with the infinite face-sharing chains of [RuCl6]3− octahedra. Intrachain interaction plays a dominant role in the overall magnetic order. Therefore, the experimental meff value is varied from the Kotani prediction for isolated d5 Ru3+ ions. Different from the MA2RuCl6, at lower temperatures, the effective moment of Ru3+ is not zero, as the ground state is J = 1/2. The moment is always lower than the ideal Kotani prediction for isolated [RuX6]3− ions, which indicates the AFM exchange interactions inside the inorganic chains. The MA3Ru2Br9 crystallizes in the 0D structure with the basic structural units of face-shared [Ru2Br9]3− dimers. Due to the exchange interaction inside the [Ru2Br9]3− dimer, the magnetic moment of MA3Ru2Br9 is much lower than the ideal d5 Ru3+ behavior. They have also studied another two series of vacancy-ordered Ru4+ halides, A2RuCl6 and A2RuBr6, where A is K+, NH4+, Rb+ or Cs+.88 It shows that Ru–X covalency, and the delocalization of metal d-electrons, can modulate the spin–orbit coupling constants systematically by changing the A cation and the halide anion. The study on the vacancy-ordered Os4+ (5d4) halides, K2OsCl6, K2OsBr6, and Na2OsBr6, suggests that they also display a Kotani-like behavior consistent with a J = 0 ground state.89
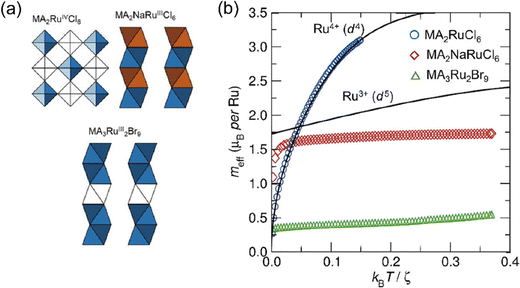 |
| Fig. 11 (a) The structure of MA2RuCl6, MA2NaRuCl6, and MA3Ru2Br9. (b) Experimental Kotani plots for MA2RuCl6, MA2NaRuCl6, and MA3Ru2Br9. The theoretical Kotani plots are given as black lines. Reproduced from ref. 87 with permission from Wiley-VCH, Copyright 2020. | |
Zheng et al. systematically studied a series of manganese halides of 2D (4-fluorobenzylamine)2MnCl4, 1D ((R)-3-fluoropyrrolidinium)MnCl3, and 0D (pyrrolidinium)2MnCl4 and demonstrated the effect of structure dimensions on spin decoherence processes.90 The spin decoherence time follows the trend 2D > 1D > 0D due to the reduced connectivity and larger distance between adjacent Mn2+–Mn2+ ions in lower structure dimensions. It indicates that the structure dimensions can strongly affect the magnetic interaction.
The 1D [(S)/(R)-MPA]2[MnCl4(H2O)] is found to be a weak chiral ferromagnet below 3.2 K, which is attributed to a spin canting AFM state induced by Dzyaloshinskii–Moriya interactions owing to spatial inversion symmetry breaking in a chiral MHS.91 (C5H14N2)[CuCl4] and (C5H8N3)[CuCl3] are 1D structures, constructed from a double edge-sharing Cu2Cl6 chain.92,93 Their magnetic properties are correlated with the long Cu–Cl distance and the bridging Cu–Cl–Cu angle, and obey the magnetostructural correlations in Cu dimer structure discovered by Hatfield and Hodgson.94 0D Mn2+ halides with isolated MnX6 octahedra or MnX4 tetrahedra are paramagnet, owing to the absence of magnetic coupling.95 Cs3Fe2Cl9 and Cs3Fe2Br9 crystallize in buckled honeycomb networks and contain isolated face-sharing [Fe2X9]3− dimers, which order antiferromagnetically through the superexchange inside the dimer. The easy-axis anisotropy and complex magnetic coupling between the dimers contribute to the complicated magnetic phase diagram, which remains to be further understood.25,96
5. Emerging properties and potential applications of magnetic metal halides
5.1 Chiral ferromagnetic TMHs
The interesting magnetic properties of metal halides are expected to couple with their semiconducting property, giving rise to multifunctional materials. For example, in chiral ferromagnetic TMHs, chirality breaks the space inversion and mirror symmetry and contributes to novel coupling effects, such as magneto circular dichroism (MCD) and the magneto-electric coupling effect. In 2020, Zhang's group first reported a pair of 2D chiral ferromagnetic perovskite, (R-MPEA)2CuCl4 and (S-MPEA)2CuCl4 (MPEA is β-methylphenethylamine) (Fig. 12(a) and (b)).18 The temperature dependent magnetization curve of the chiral and racemic compounds indicates the transition from paramagnet to ferromagnet at the Curie temperature of 6 K (Fig. 12(b)). It should be noted that (R-MPEA)2CuCl4 and (S-MPEA)2CuCl4 have almost the same magnetic moment values, while the racemic compound has a smaller moment value at low temperatures, which might be attributed to the symmetric structure of the racemic one. The MCD effect is present when a chiral compound absorbs unpolarized light under an external magnetic field. The structure origin of the MCD effect is the magneto-chiral anisotropy. MCD spectra (B = 1 T) of (R-MPEA)2CuCl4 and (S-MPEA)2CuCl4 at 2 K are shown in Fig. 12(d). The R and S compounds exhibit symmetric and opposite optical signal ΔA, suggesting the co-existence of the chirality and magnetism in the structures.
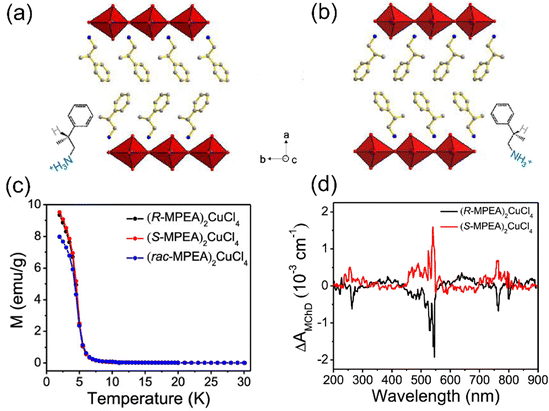 |
| Fig. 12 Single crystal structures of (R-MPEA)2CuCl4 (a) and (S-MPEA)2CuCl4 (b). (c) Temperature dependence of the magnetizations of (R-MPEA)2CuCl4, (S-MPEA)2CuCl4, and (rac-MPEA)2CuCl4 in applied fields of 100 Oe. (d) MCD spectrum recorded on (R-MPEA)2CuCl4 and (S-MPEA)2CuCl4 at 2 K. Reproduced from ref. 18 with permission from American Chemical Society, Copyright 2020. | |
Taniguchi et al. demonstrated the magneto-electric correlation in (R/S-MPEA)2CuCl4.97 The chiral-polar molecules were intercalated between ferromagnetic Cu–Cl layers to afford a non-centrosymmetric ferromagnet. Electric polarization occurs not only in the diamagnetic organic spacers but also in the magnetic inorganic layers. The magneto-electric correlation was clearly observed by measuring the magneto-electric directional anisotropy, in which an optical absorption coefficient changes with reversal of the light propagating direction under a low magnetic field of about 50 mT. This work suggests a new strategy to design functional magneto-electric multiferroics. Researchers also found spin-dependent charge transport with a high filtration efficiency (90%) in chiral ferromagnetic (R/S-MBA)2CuX4 (MBA = methylbenzylammonium, X = Cl, Br).98 The lead-free and air-stable chiral TMHs exhibit potential applications in spintronic devices.
5.2 Dilute magnetic metal halides
Apart from the above-mentioned magnetic metal halides, which consist of the periodic lattice of transition metal ions, there is another kind of magnetic metal halides, dilute magnetic metal halides. They are formed by doping with a small number of paramagnetic transition metal ions into the diamagnetic perovskite lattice. Dilute magnetic semiconductors (DMS) combine the properties of semiconductors and ferromagnets. The mechanism of ferromagnetism in typical DMS including metal oxides, III–V and II–VI semiconductors has been demonstrated. The ferromagnetism in Mn-doped semiconductors can be explained using the p–d Zener model, which attributes it to the exchange between band carriers and localized magnetic spins.99 Considering the Friedel oscillations of spin density, Ruderman, Kittel, Kasuya, and Yosida proposed the (Ruderman–Kittel–Kasuya–Yosida) RKKY theory,100 which shows that magnetic spins from impurity dopants can interact with charge carriers or lattice polarons and results in long-range FM coupling. Metal halide perovskites possess both excellent defect tolerance and convenient solution processability, enabling them to be a tunable platform to incorporate paramagnetic transition metal ions. The coupling between long-range ferromagnetic order and excellent optoelectronic properties in dilute magnetic metal halides can induce emerging properties and applications. The modulation of charge carriers in dilute magnetic metal halides can potentially tune the magnetic properties in a new way.
The Horvath group reported the ferromagnetic dilute perovskite and revealed that the photo-excited electrons rapidly melt the local magnetic order through the RKKY interactions.19 They synthesized the Mn-doped CH3NH3(Mn:Pb)I3 crystals with a Mn doping ratio of 10% (Fig. 13(a)). The temperature dependent magnetization curve indicates the ferromagnetic order below the Curie temperature Tc = 25 K. The spontaneous ferromagnetic order results in the rapid shift of the resonance field B0 and broadening of the electron spin resonance (ESR) linewidth below Tc (Fig. 13(b)). Through the in situ ESR measurement under illumination, the light-on ESR signal is weaker and narrower than the dark signal (Fig. 13(c)). As the intensity of the ESR signal is proportional to the ferromagnetic volume, the decreased intensity under illumination suggests a decrease of the ferromagnetic order. This phenomenon is attributed to the light-induced RKKY interactions, in which the photo-excited conduction electrons melt the aligned spin order in the ferromagnetic ground state. The illumination melted ferromagnetism provides a fast and convenient method to manipulate the spin order. By using the light to melt the ferromagnetic ground state, only a small magnetic field is needed to rewrite the spin order in the sample (Fig. 13(d)). Ren et al. also found that the photocurrent of Mn-doped MAPbI3 perovskite solar cells was slightly increased under a magnetic field of 1 T as the FM coupling enhanced the electron hopping and decreased the photoresistance.101 It suggests the potential of TMHs in modulating the photovoltaic performance.
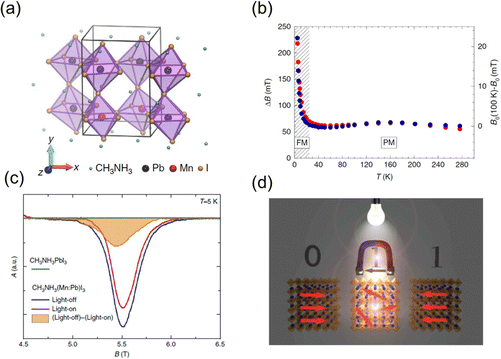 |
| Fig. 13 (a) Sketch of the crystal structure of the CH3NH3(Mn:Pb)I3 crystal. (b) ESR linewidth (red dots) and resonant field (blue dots, offset by a reference value B0) as a function of temperature recorded at 9.4 GHz. (c) ESR spectra at 157 GHz and 5 K of pristine CH3NH3PbI3 (green line–no signal), of CH3NH3(Mn:Pb)I3 in dark (blue line) and its reduction (red line) on visible light illumination. (d) Schematic illustration of rewriting a magnetic bit. Reproduced from ref. 19 with permission from Nature Springer, Copyright 2016. | |
Neumann et al. studied the magneto-optical properties of Mn-doped Ruddlesden–Popper hybrid perovskite thin-film Mn:(PEA)2PbI4 (Fig. 14(a)) and found that Mn doping will affect the spin polarization.102 The introduction of Mn2+ can induce the paramagnetic order (Fig. 14(b)) and Zeeman effect in the perovskite structure and increase the energy degeneracy, which gives rise to the imbalanced population of the exciton spin states. A dark exciton is populated in the presence of a magnetic field. The spin polarization reaches a saturation value of 13% at 4 K under a magnetic field of 6 T (Fig. 14(c)). This is induced by the spin-dependent exciton dynamics, indicating an Mn-mediated spin–flip process (Fig. 14(d)), which enables the direct control of exciton spin physics via a magnetic field. Subsequent study of Co2+ doped (PEA)2PbI4 also confirmed the above findings.103 These studies highlight the potential of TMHs in the application of magneto-optics.”
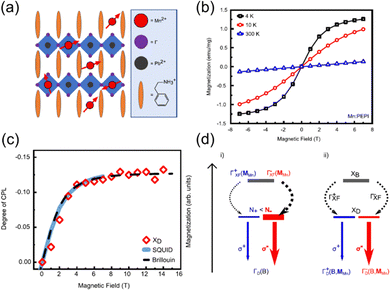 |
| Fig. 14 (a) Sketch of the Ruddlesden–Popper quantum well structure of Mn:(PEA)2PbI4. (b) The isothermal magnetization curves at different temperatures. (c) Circular polarization of the PL and magnetization curve of Mn:(PEA)2PbI4 at 4 K and Brillouin function for a non-interacting J = 5/2 spin system. (d) Proposed interaction mechanism between excitons and Mn2+ spins in an external magnetic field. XB and XD denote bright and dark excitons, respectively. Reproduced from ref. 102 with permission from Nature Springer, Copyright 2021. | |
These findings revealed that the doped magnetic ions in perovskite could induce various cross effects, which enable the manipulation of spin order and exciton spin physics via external stimulate. Although various dilute magnetic perovskites have been synthesized to date,19,102–104 there is an absence of strong ferromagnetism. This might be attributed to the lack of manipulation of carrier concentration. It is thus critical to realize the simultaneous control of localized magnetic moments and carrier concentration to tune the magnetic properties in dilute magnetic perovskites.
Conclusion
In summary, we have summarized the chemical and structure diversity and magnetic properties of transition metal halides in terms of different structure dimensions and metal ions. The magnetic order in metal halides is determined by the structure geometry, which enables tuning magnetic properties through structural engineering. The abundant composition in metal halides provides a splendid platform to design functional magnetic properties. We expect that future research in this field will be focused on the following directions.
(1) Design of functional organic spacers in hybrid transition metal halides. The magnetic order in various transition metal halides has been extensively studied. There remains a sizeable exploration space for the design of organic spacers. For example, chiral-polar organics are expected to bring chiral ferromagnetic metal halides with multiferroic behavior. Incorporating the abundant magnetic and electric properties in a single material can expand their application and induce distinct cross coupling effects.
(2) One of the advantages of MHS is the solution-processable structure feature, which is convenient to make thin films for device fabrication. Most studies on the magnetic properties of TMHs are focused on single crystals. Expanding the study to thin films and nanocrystals is expected to bring new magnetic phenomenon, which is of huge potential in spintronic devices.
(3) Weak FM coupling and low Curie temperature are generally observed in TMHs, due to the M–X–M superexchange path. Dilute magnetic metal halides provide a new platform to modulate the magnetic properties. The charge carrier concentration in MHS can be easily modulated by photo-excitation and electrical doping, which may induce dramatic modification in the long-range magnetic order.
Conflicts of interest
There are no conflicts to declare.
Acknowledgements
This work was supported by the National Natural Science Foundation of China (Grant No. 22205186), the Research Grants Council of Hong Kong via the Early Career Scheme (ECS, 26300721), and Fei Chi En Education and Research Fund from Y-Lot Foundation. We acknowledge the start-up funding support from the Hong Kong University of Science and Technology (HKUST) School of Science (SSCI) and the Department of Chemistry (R9270).
References
- X.-K. Liu, W. Xu, S. Bai, Y. Jin, J. Wang, R. H. Friend and F. Gao, Metal halide perovskites for light-emitting diodes, Nat. Mater., 2021, 20, 10–21 CrossRef CAS.
- S. D. Stranks and H. J. Snaith, Metal–halide perovskites for photovoltaic and light-emitting devices, Nat. Nanotechnol., 2015, 10, 391–402 CrossRef CAS.
- W. Zhang, G. E. Eperon and H. J. Snaith, Metal halide perovskites for energy applications, Nat. Energy, 2016, 1, 16048 CrossRef CAS.
- B. Dieny and M. Chshiev, Perpendicular magnetic anisotropy at transition metal/oxide interfaces and applications, Rev. Mod. Phys., 2017, 89, 025008 CrossRef.
- D. Kan, R. Aso, R. Sato, M. Haruta, H. Kurata and Y. Shimakawa, Tuning magnetic anisotropy by interfacially engineering the oxygen coordination environment in a transition metal oxide, Nat. Mater., 2016, 15, 432–437 CrossRef CAS PubMed.
- C. Bellitto, E. M. Bauer and G. Righini, Organic–inorganic hybrids: from magnetic perovskite metal(II) halides to multifunctional metal(II) phosphonates, Coord. Chem. Rev., 2015, 289–290, 123–136 CrossRef CAS.
- L. Mao, J. Chen, P. Vishnoi and A. K. Cheetham, The Renaissance of Functional Hybrid Transition-Metal Halides, Acc. Mater. Res., 2022, 3, 439–448 CrossRef CAS.
- W. Ning and F. Gao, Structural and Functional Diversity in Lead-Free Halide Perovskite Materials, Adv. Mater., 2019, 31, e1900326 CrossRef PubMed.
- L. J. de Jongh, A. C. Botterman, F. R. de Boer and A. R. Miedema, Transition Temperature of the Two-Dimensional Heisenberg Ferromagnet with S = ½, J. Appl. Phys., 1969, 40, 1363–1365 CrossRef CAS.
- J. E. Drumheller, P. H. Amundson and K. Emerson, Electron Paramagnetic Resonance of Ethylammonium- and Methylammonium-Tetrachlorocuprate, J. Chem. Phys., 1969, 51, 5729–5730 CrossRef CAS.
- M. Gibertini, M. Koperski, A. F. Morpurgo and K. S. Novoselov, Magnetic 2D materials and heterostructures, Nat. Nanotechnol., 2019, 14, 408–419 CrossRef CAS PubMed.
- B. Zhang, Y. Zeng, Z.-J. Zhao, D.-P. Qiu, T. Zhang and Y.-L. Hou, Magnetic two-dimensional chromium trihalides: structure, properties and modulation, Rare Met., 2022, 41, 2921–2942 CrossRef CAS.
- M. Cai, M.-P. Miao, Y. Liang, Z. Jiang, Z.-Y. Liu, W.-H. Zhang, X. Liao, L.-F. Zhu, D. West, S. Zhang and Y.-S. Fu, Manipulating single excess electrons in monolayer transition metal dihalide, Nat. Commun., 2023, 14, 3691 CrossRef CAS.
- D. Lam, D. Lebedev, L. Kuo, V. K. Sangwan, B. M. Szydłowska, F. Ferraresi, A. Söll, Z. Sofer and M. C. Hersam, Liquid-Phase Exfoliation of Magnetically and Optoelectronically Active Ruthenium Trichloride Nanosheets, ACS Nano, 2022, 16, 11315–11324 CrossRef CAS PubMed.
- J. Xue, Z. Wang, A. Comstock, Z. Wang, H. H. Y. Sung, I. D. Williams, D. Sun, J. Liu and H. Lu, Chemical Control of Magnetic Ordering in Hybrid Fe–Cl Layered Double Perovskites, Chem. Mater., 2022, 34, 2813–2823 CrossRef CAS.
- Y. Asensio, S. Marras, D. Spirito, M. Gobbi, M. Ipatov, F. Casanova, A. Mateo-Alonso, L. E. Hueso and B. Martín-García, Magnetic Properties of Layered Hybrid Organic-Inorganic Metal-Halide Perovskites: Transition Metal, Organic Cation and Perovskite Phase Effects, Adv. Funct. Mater., 2022, 32, 2207988 CrossRef CAS.
- A. H. Comstock, C. T. Chou, Z. Wang, T. Wang, R. Song, J. Sklenar, A. Amassian, W. Zhang, H. Lu, L. Liu, M. C. Beard and D. Sun, Hybrid magnonics in hybrid perovskite antiferromagnets, Nat. Commun., 2023, 14, 1834 CrossRef CAS.
- B. Sun, X.-F. Liu, X.-Y. Li, Y. Zhang, X. Shao, D. Yang and H.-L. Zhang, Two-Dimensional Perovskite Chiral Ferromagnets, Chem. Mater., 2020, 32, 8914–8920 CrossRef CAS.
- B. Nafradi, P. Szirmai, M. Spina, H. Lee, O. V. Yazyev, A. Arakcheeva, D. Chernyshov, M. Gibert, L. Forro and E. Horvath, Optically switched magnetism in photovoltaic perovskite CH3NH3(Mn:Pb)I3, Nat. Commun., 2016, 7, 13406 CrossRef CAS.
- A. Okazaki and Y. Suemune, The Crystal Structures of KMnF3, KFeF3, KCoF3, KNiF3 and KCuF3 above and below their Néel Temperatures, J. Phys. Soc. Jpn., 1961, 16, 671–675 CrossRef CAS.
- C. Li, X. Lu, W. Ding, L. Feng, Y. Gao and Z. Guo, Formability of ABX3 (X = F, Cl, Br, I) halide perovskites, Acta Crystallogr., 2008, 64, 702–707 CAS.
- J. P. Steadman and R. D. Willett, The crystal structure of (C2H5NH3)2CuCl4, Inorg. Chim. Acta, 1970, 4, 367–371 CrossRef CAS.
- K. Tichy, J. Benes, W. Halg and H. Arend, Neutron diffraction study of twinned crystals of ethylenediammonium copper tetrachloride and ethylenediammonium manganese tetrachloride, Acta Crystallogr., 1978, 34, 2970–2981 CrossRef.
- R. D. Willett, Crystal Structure of CH3NH3NiCl3, J. Chem. Phys., 2004, 45, 3737–3740 CrossRef.
- F. Wei, F. Brivio, Y. Wu, S. Sun, P. D. Bristowe and A. K. Cheetham, Synthesis, crystal structure, magnetic and electronic properties of the caesium-based transition metal halide Cs3Fe2Br9, J. Mater. Chem. C, 2018, 6, 3573–3577 RSC.
- K.-Y. Kim, G. Park, J. Cho, J. Kim, J.-S. Kim, J. Jung, K. Park, C.-Y. You and I.-H. Oh, Intrinsic Magnetic Order of Chemically Exfoliated 2D Ruddlesden–Popper Organic–Inorganic Halide Perovskite Ultrathin Films, Small, 2020, 16, 2005445 CrossRef CAS PubMed.
- T. A. Fasasi, C. M. Sin, L. Taili, S. W. Tsang and A. Ruotolo, Effect of the magnetic order on the magneto-photocurrent of organo-metal halide perovskites, Opt. Mater., 2022, 124, 112011 CrossRef CAS.
- P. W. Anderson, New Approach to the Theory of Superexchange Interactions, Phys. Rev., 1959, 115, 2–13 CrossRef CAS.
- W. D. van Amstel and L. J. de Jongh, Magnetic measurements on (CH3NH3)2MnCl4, a quasi two-dimensional Heisenberg antiferromagnet, Solid State Commun., 1972, 11, 1423–1429 CrossRef CAS.
- G. Heger, E. Henrich and B. Kanellakopulos, Investigations of a quasi two-dimensional Heisenberg antiferromagnetic system. Nondeuterated and deuterated alkyl-ammonium-tetrachloromanganate, Solid State Commun., 1973, 12, 1157–1165 CrossRef CAS.
- P. Day, New transparent ferromagnets, Acc. Chem. Res., 1979, 12, 236–243 CrossRef CAS.
- L. J. de Jongh, Observation of lattice- and spin-dimensionality crossovers in the susceptibility of quasi 2-dimensional Heisenberg ferromagnets, Phys. B + C, 1976, 82, 247–261 CrossRef.
- Z. G. Soos, K. T. McGregor, T. T. P. Cheung and A. J. Silverstein, Antisymmetric and anisotropic exchange in ferromagnetic copper(II) layers, Phys. Rev. B: Condens. Matter Mater. Phys., 1977, 16, 3036–3048 CrossRef CAS.
- C. Bellitto and P. Day, Bis(monoalkylammonium) tetrachlorochromates(II): a new series of two-dimensional ionic ferromagnets, J. Chem. Soc., Chem. Commun., 1976, 870–871 RSC.
- C. Bellitto and P. Day, Magnetic susceptibility and optical spectra of the
organic-intercalated two-dimensional ferromagnets bis(monomethylammonium)- and bis(monoethylammonium) tetrachlorochromate(II), J. Chem. Soc., Dalton Trans., 1978, 1207–1212 RSC.
- P. Day, M. T. Hutchings, E. Janke and P. J. Walker, Co-operative Jahn–Teller ordering in the crystal structure of Rb2CrCl4: a two-dimensional easy-plane ionic ferromagnet, J. Chem. Soc., Chem. Commun., 1979, 711–713 RSC.
- D. I. Khomskii and K. I. Kugel, Orbital and magnetic structure of two-dimensional ferromagnets with Jahn–Teller ions, Solid State Commun., 1973, 13, 763–766 CrossRef CAS.
- D. B. Mitzi, Synthesis, Structure, and Properties of Organic-Inorganic Perovskites and Related Materials, Prog. Inorg. Chem., 1999, 48, 1–121 CAS.
- H. T. Witteveen, Magnetic measurements on polycrystalline samples of the layer-type compounds Rb2CuCl4, Rb2CuCl3Br and Rb2CuCl2Br2, Physica, 1974, 71, 204–236 CrossRef CAS.
- B. J. Hathaway and D. E. Billing, The electronic properties and stereochemistry of mono-nuclear complexes of the copper(II) ion, Coord. Chem. Rev., 1970, 5, 143–207 CrossRef CAS.
- W. E. Estes, D. B. Losee and W. E. Hatfield, The magnetic properties of several quasi two-dimensional Heisenberg layer compounds: a new class of ferromagnetic insulators involving halocuprates, J. Chem. Phys., 2008, 72, 630–638 CrossRef.
- A. Furrer, A. Podlesnyak and K. W. Krämer, Extraction of exchange parameters in transition-metal perovskites, Phys. Rev. B: Condens. Matter Mater. Phys., 2015, 92, 104415 CrossRef.
- E. M. Mozur and R. Seshadri, Methods and Protocols: Practical Magnetic Measurement, Chem. Mater., 2023, 35, 3450–3463 CrossRef CAS.
- P. Harris, S. Larsen and B. Lebech, A single crystal neutron diffraction study of KMnCl3. Its twinning and magnetic structure, J. Phys. Chem. Solids, 1992, 53, 1021–1025 CrossRef CAS.
- D. B. Straus, T. Klimczuk, X. Xu and R. J. Cava, Antiferromagnetic Order in the Rare-Earth Halide Perovskites CsEuBr3 and CsEuCl3, Chem. Mater., 2022, 34, 10772–10777 CrossRef CAS.
- P. Zhang, F. Benner, N. F. Chilton and S. Demir, Organometallic lanthanide bismuth cluster single-molecule magnets, Chem, 2022, 8, 717–730 CAS.
- G. Kent, E. Morgan, K. R. Albanese, A. Kallistova, A. Brumberg, L. Kautzsch, G. Wu, P. Vishnoi, R. Seshadri and A. K. Cheetham, Elusive Double Perovskite Iodides: Structural, Optical, and Magnetic Properties, Angew. Chem., Int. Ed., 2023, e202306000 CAS.
- A. Epstein, E. Gurewitz, J. Makovsky and H. Shaked, Magnetic Structure and Two-Dimensional Behavior of Rb2MnCl4 and Cs2MnCl4, Phys. Rev. B: Condens. Matter Mater. Phys., 1970, 2, 3703–3706 CrossRef.
- B. Huang, J.-Y. Zhang, R.-K. Huang, M.-K. Chen, W. Xue, W.-X. Zhang, M.-H. Zeng and X.-M. Chen, Spin-reorientation-induced magnetodielectric coupling effects in two layered perovskite magnets, Chem. Sci., 2018, 9, 7413–7418 RSC.
- S. H. Park, I. H. Oh, S. Park, Y. Park, J. H. Kim and Y. D. Huh, Canted antiferromagnetism and spin reorientation transition in layered inorganic-organic perovskite (C6H5CH2CH2NH3)2MnCl4, Dalton Trans., 2012, 41, 1237–1242 RSC.
- L. Septiany, D. Tulip, M. Chislov, J. Baas and G. R. Blake, Polar Structure and Two-Dimensional Heisenberg Antiferromagnetic Properties of Arylamine-Based Manganese Chloride Layered Organic–Inorganic Perovskites, Inorg. Chem., 2021, 60, 15151–15158 CrossRef CAS.
- H. Zhao, H. Fu, Z. Hu, Q. Fu, H. Tao, J. Weng, L. Xiong and Z. Cheng, Magnetic hybrid organic–inorganic perovskite (CH3NH3)2XCl4 (X = Mn, Cu, Co) crystals, CrystEngComm, 2021, 23, 5208–5213 RSC.
- J. Han, S. Nishihara, K. Inoue and M. Kurmoo, On the Nature of the Structural and Magnetic Phase Transitions in the Layered Perovskite-Like (CH3NH3)2[FeIICl4], Inorg. Chem., 2014, 53, 2068–2075 CrossRef CAS PubMed.
- J. Han, S. Nishihara, K. Inoue and M. Kurmoo, High Magnetic Hardness for the Canted Antiferromagnetic, Ferroelectric, and Ferroelastic Layered Perovskite-like (C2H5NH3)2[FeIICl4], Inorg. Chem., 2015, 54, 2866–2874 CrossRef CAS.
- Y. Nakayama, S. Nishihara, K. Inoue, T. Suzuki and M. Kurmoo, Coupling of Magnetic and Elastic Domains in the Organic-Inorganic Layered Perovskite-Like (C6H5C2H4NH3)2Fe(II)Cl4 Crystal, Angew. Chem., Int. Ed., 2017, 56, 9367–9370 CrossRef CAS PubMed.
- L. O. Snively, P. L. Seifert, K. Emerson and J. E. Drumheller, Magnetic susceptibility of (NH3CH2CH2NH3)CuCl4: a layered structure with strong interlayer magnetic coupling, Phys. Rev. B: Condens. Matter Mater. Phys., 1979, 20, 2101–2104 CrossRef CAS.
- L. J. De Jongh and A. R. Miedema, Experiments on simple magnetic model systems, Adv. Phys., 2001, 50, 947–1170 CrossRef.
- J. J. M. Steijger, E. Frikkee, L. J. De Jongh and W. J. Huiskamp, On the magnetic behaviour of the layered ferromagnets (CH3NH3)2CuCl4 and (CD3ND3)2CuCl4, Phys. B + C, 1984, 123, 271–283 CrossRef CAS.
- R. D. Willett, F. H. Jardine, I. Rouse, R. J. Wong, C. P. Landee and M. Numata, Crystal structure, magnetic susceptibility, and EPR study of bis-(P-alaninium tetrachlorocuprate(II)): spin-diffusion effects in a two-dimensional square planar ferromagnet with anisotropic and antisymmetric exchange, Phys. Rev. B: Condens. Matter Mater. Phys., 1981, 24, 5372–5381 CrossRef CAS.
- R. J. H. Wong, R. D. Willett and J. E. Drumheller, An EPR study of interlayer exchange coupling in the quasi-two-dimensional salts, (CnH2n+1NH3)2CuCl4, with n = 1, 2, and 3, J. Chem. Phys., 1981, 74, 6018–6021 CrossRef CAS.
- Y. Kimishima, The Magnetic Behaviors of Quasi-Two Dimensional Antiferromagnet (CH3NH3)2CuBr4, J. Phys. Soc. Jpn., 1980, 49, 62–66 CrossRef CAS.
- G. V. Rubenacker, D. N. Haines, J. E. Drumheller and K. Emerson, Magnetic properties of the alkanediammonium copper halides, J. Magn. Magn. Mater., 1984, 43, 238–242 CrossRef CAS.
- T. M. Kite and J. E. Drumheller, Electron paramagnetic resonance of the eclipsed layered compounds NH3(CH2)nNH3CuBr4 with n = 2, 3, 4, J. Magn. Reson., 1983, 54, 253–256 CAS.
- T. M. Kite, J. E. Drumheller and K. Emerson, EPR investigations of the quasi-two-dimensional eclipsed structures of (NH3(CH2)nNH3)CuCl4 with n = 2, 3, and 4, J. Magn. Reson., 1982, 48, 20–25 CAS.
- L. O. Snively, G. F. Tuthill and J. E. Drumheller, Measurement and caiculation of the superexchange interaction through the two-halide bridge in the eclipsed layered compounds [NH3(CH2)nNH3]CuX for n = 2-5 and X = C14 and Cl2Br2, Phys. Rev. B, 1981, 24, 5349–5355 CrossRef CAS.
- G. V. Rubenacker, S. Waplak, S. L. Hutton, D. N. Haines and J. E. Drumheller, Magnetic susceptibility and magnetic resonance in the ordered state of single-crystal [NH3(CH2)2HN3]CuBr4, J. Appl. Phys., 1985, 57, 3341–3342 CrossRef CAS.
- G. V. Rubenacker and J. E. Drumheller, Magnetic susceptibility of [NH3(CH2)nNH3]CuCl4xBr4(1−x) with n = 5 and 7, J. Magn. Magn. Mater., 1989, 79, 119–121 CrossRef CAS.
- D. B. Losee and W. E. Hatfield, Single-crystal magnetic-susceptibility measurements on the nearly two-dimensional Heisenberg system [NH3(CH2CH2)2NH2][CuCl4]Cl, Phys. Rev. B: Condens. Matter Mater. Phys., 1974, 10, 212–218 CrossRef CAS.
- B. Sun, X. F. Liu, X. Y. Li, Y. Cao, Z. Yan, L. Fu, N. Tang, Q. Wang, X. Shao, D. Yang and H. L. Zhang, Reversible Thermochromism and Strong Ferromagnetism in Two-Dimensional Hybrid Perovskites, Angew. Chem., Int. Ed., 2020, 59, 203–208 CrossRef CAS.
- A. O. Polyakov, A. H. Arkenbout, J. Baas, G. R. Blake, A. Meetsma, A. Caretta, P. H. M. Van Loosdrecht and T. T. M. Palstra, Coexisting Ferromagnetic and Ferroelectric Order in a CuCl4-based Organic–Inorganic Hybrid, Chem. Mater., 2012, 24, 133–139 CrossRef CAS.
- C. Bellitto, P. Day and T. E. Wood, Magnetic susceptibility and optical study of the organic-intercalated two-dimensional ionic ferromagnet bis(benzylammonium) tetrachlorochromate(II), J. Chem. Soc., Dalton Trans., 1986, 847–851 RSC.
- G. Staulo and C. Bellitto, (C6H5CH2NH3)2CrBr3.3I0.7: a new insulating ferromagnet with a Curie temperature of 51 K, J. Mater. Chem., 1991, 1, 915–918 RSC.
- M. J. Stead and P. Day, Preparation, characterization, and magnetic properties of organic-intercalated two-dimensional ionic ferromagnets (CnH2n+1NH3)2CrCl4 (n = 3, 5, or 12), J. Chem. Soc., Dalton Trans., 1982, 1081–1084 RSC.
- P. Day, Correlation of structures and properties of ferromagnetic tetrahalogenochromate(II) salts, J. Magn. Magn. Mater., 1986, 54–57, 1442–1446 CrossRef CAS.
- C. Bellitto and P. Day, Organic-intercalated halogenochromates(II): low-dimensional magnets, J. Mater. Chem., 1992, 2, 265–271 RSC.
- C. Bellitto, H. Brunner and H. J. I. C. Guedel, Optical spectroscopy of one-and two-dimensional ionic magnets of Cr2+: CsCrCl3, (CH3)4NCrCl3, (CH3)4NCrBr3, CrCl2, (C2H5NH3)2CrCl4, and (C2H5NH3)2CrBr4, Inorg. Chem., 1987, 26, 2750–2754 CrossRef CAS.
- C. Bellitto, P. Filaci and S. J. I. C. Patrizio, Zero-field magnetic susceptibility study of the magnetic phase transition in the two-dimensional ionic ferromagnet bis (benzylammonium) tetrabromochromate(II),(C6H5CH2NH3)2CrBr4, Inorg. Chem., 1987, 26, 191–194 CrossRef CAS.
- Y. Ai, R. Sun, W. Q. Liao, X. J. Song, Y. Y. Tang, B. W. Wang, Z. M. Wang, S. Gao and R. G. Xiong, Unprecedented Ferroelectricity and Ferromagnetism in a Cr2+-Based Two-Dimensional Hybrid Perovskite, Angew. Chem., Int. Ed., 2022, 61, e202206034 CrossRef CAS PubMed.
- C. Bellitto and P. Day, Feature article. Organic-intercalated halogenochromates(II): low-dimensional magnets, J. Mater. Chem., 1992, 2, 265–271 RSC.
- P. Vishnoi, J. L. Zuo, X. Li, D. C. Binwal, K. E. Wyckoff, L. Mao, L. Kautzsch, G. Wu, S. D. Wilson, M. G. Kanatzidis, R. Seshadri and A. K. Cheetham, Hybrid Layered Double Perovskite Halides of Transition Metals, J. Am. Chem. Soc., 2022, 144, 6661–6666 CrossRef CAS.
- D. C. Binwal, P. P. Mudoi, D. P. Panda and P. Vishnoi, Molybdenum chloride double perovskites: dimensionality control of optical and magnetic properties, Chem. Sci., 2023, 14, 3982–3989 RSC.
- B. Vargas, R. Torres-Cadena, J. Rodríguez-Hernández, M. Gembicky, H. Xie, J. Jiménez-Mier, Y.-S. Liu, E. Menéndez-Proupin, K. R. Dunbar, N. Lopez, P. Olalde-Velasco and D. Solis-Ibarra, Optical, Electronic, and Magnetic Engineering of 〈111〉 Layered Halide Perovskites, Chem. Mater., 2018, 30, 5315–5321 CrossRef CAS.
- B. Vargas, G. Rodríguez-López and D. Solis-Ibarra, The Emergence of Halide Layered Double Perovskites, ACS Energy Lett., 2020, 5, 3591–3608 CrossRef CAS.
- B. Vargas, E. Ramos, E. Pérez-Gutiérrez, J. C. Alonso and D. Solis-Ibarra, A Direct Bandgap Copper–Antimony Halide Perovskite, J. Am. Chem. Soc., 2017, 139, 9116–9119 CrossRef CAS PubMed.
- T. Lee, D. B. Straus, K. P. Devlin, X. Gui, P. Louka, W. Xie and R. J. Cava, Antiferromagnetic to Ferromagnetic Coupling Crossover in Hybrid Nickel Chain Perovskites, Inorg. Chem., 2022, 61, 10486–10492 CrossRef CAS PubMed.
- T. Lee, D. B. Straus, X. Xu, W. Xie and R. J. Cava, Tunable Magnetic Transition Temperatures in Organic–Inorganic Hybrid Cobalt Chloride Hexagonal Perovskites, Chem. Mater., 2023, 35, 1745–1751 CrossRef CAS.
- P. Vishnoi, J. L. Zuo, T. A. Strom, G. Wu, S. D. Wilson, R. Seshadri and A. K. Cheetham, Structural Diversity and Magnetic Properties of Hybrid Ruthenium Halide Perovskites and Related Compounds, Angew. Chem., Int. Ed., 2020, 59, 8974–8981 CrossRef CAS.
- P. Vishnoi, J. L. Zuo, J. A. Cooley, L. Kautzsch, A. Gómez-Torres, J. Murillo, S. Fortier, S. D. Wilson, R. Seshadri and A. K. Cheetham, Chemical Control of Spin–Orbit Coupling and Charge Transfer in Vacancy-Ordered Ruthenium(IV) Halide Perovskites, Angew. Chem., Int. Ed., 2021, 60, 5184–5188 CrossRef CAS PubMed.
- M. Saura-Múzquiz, M. Avdeev, H. E. A. Brand and B. J. Kennedy, Structural and Magnetic Properties of Some Vacancy-Ordered Osmium Halide Perovskites, Inorg. Chem., 2022, 61, 15961–15972 CrossRef.
- H. Zheng, A. Ghosh, M. J. Swamynadhan, G. Wang, Q. Zhang, X. Wu, I. Abdelwahab, W. P. D. Wong, Q.-H. Xu, S. Ghosh, J. Chen, B. J. Campbell, A. Stroppa, J. Lin, R. Mahendiran and K. P. Loh, Electron Spin Decoherence Dynamics in Magnetic Manganese Hybrid Organic–Inorganic Crystals: The Effect of Lattice Dimensionality, J. Am. Chem. Soc., 2023, 145, 18549–18559 CrossRef CAS PubMed.
- K. Taniguchi, P.-J. Huang, S. Kimura and H. Miyasaka, Chiral weak ferromagnets formed in one-dimensional organic–inorganic hybrid manganese chloride hydrates, Dalton Trans., 2022, 51, 17030–17034 RSC.
- M. Bourwina, R. Msalmi, S. Walha, M. M. Turnbull, T. Roisnel, F. Costantino, E. Mosconi and H. Naïli, A new lead-free 1D hybrid copper perovskite and its structural, thermal, vibrational, optical and magnetic characterization, J. Mater. Chem. C, 2021, 9, 5970–5976 RSC.
- W. Jabeur, R. Msalmi, M. Korb, M. Holub, E. Mosconi, E. Čižmár, A. Tozri, N. A. Althubiti and H. Naïli, Optical and magnetic characterization of one-dimensional Cu(II)-based perovskite: a high UV-Vis-NIR absorber, J. Mater. Chem. C, 2021, 9, 17158–17166 RSC.
- W. E. Marsh, K. C. Patel, W. E. Hatfield and D. J. Hodgson, Magnetic interactions in chloro-bridged copper(II) dimers. Structural and magnetic characterization of bis(.mu.-chloro)bis[chloro(N,N,N′-triethylethylenediamine)copper(II)], [Cu(Et3en)Cl2]2, Inorg. Chem., 1983, 22, 511–515 CrossRef CAS.
- D. P. Panda, D. Swain and A. Sundaresan, Photophysical and Magnetic Properties in Zero-Dimensional (H2DABCO)MX4·nH2O (M = Mn and Cu; X = Cl and Br; n = 0, 1, and 4), J. Phys. Chem. C, 2022, 126, 13291–13299 CrossRef CAS.
- Y. Ishii, Y. Narumi, Y. Matsushita, M. Oda, T. Kida, M. Hagiwara and H. K. Yoshida, Field-induced successive phase transitions in the J1–J2 buckled honeycomb antiferromagnet Cs3Fe2Cl9, Phys. Rev. B, 2021, 103, 104433 CrossRef CAS.
- K. Taniguchi, M. Nishio, N. Abe, P. J. Huang, S. Kimura, T. H. Arima and H. Miyasaka, Magneto-Electric Directional Anisotropy in Polar Soft Ferromagnets of Two-Dimensional Organic–Inorganic Hybrid Perovskites, Angew. Chem., Int. Ed., 2021, 60, 14350–14354 CrossRef CAS PubMed.
- Y. Lu, Q. Wang, R. He, F. Zhou, X. Yang, D. Wang, H. Cao, W. He, F. Pan, Z. Yang and C. Song, Highly Efficient Spin-Filtering Transport in Chiral Hybrid Copper Halides, Angew. Chem., Int. Ed., 2021, 60, 23578–23583 CrossRef CAS PubMed.
- T. Dietl, A ten-year perspective on dilute magnetic semiconductors and oxides, Nat. Mater., 2010, 9, 965–974 CrossRef CAS.
- T. Dietl, A. Haury and Y. Merle, d'Aubigné, Free carrier-induced ferromagnetism in structures of diluted magnetic semiconductors, Phys. Rev. B: Condens. Matter Mater. Phys., 1997, 55, R3347–R3350 CrossRef CAS.
- L. Ren, Y. Wang, M. Wang, S. Wang, Y. Zhao, C. Cazorla, C. Chen, T. Wu and K. Jin, Tuning Magnetism and Photocurrent in Mn-Doped Organic–Inorganic Perovskites, J. Phys. Chem. Lett., 2020, 11, 2577–2584 CrossRef CAS PubMed.
- T. Neumann, S. Feldmann, P. Moser, A. Delhomme, J. Zerhoch, T. van de Goor, S. Wang, M. Dyksik, T. Winkler, J. J. Finley, P. Plochocka, M. S. Brandt, C. Faugeras, A. V. Stier and F. Deschler, Manganese doping for enhanced magnetic brightening and circular polarization control of dark excitons in paramagnetic layered hybrid metal–halide perovskites, Nat. Commun., 2021, 12, 3489 CrossRef CAS PubMed.
- Y. Liu, Y. Jiang, Z. Xu, L. Li, D. Zhang, W. Zheng, D. Liang, B. Zheng, H. Liu, X. Sun, C. Zhu, L. Lin, X. Zhu, H. Duan, Q. Yuan, X. Wang, S. Wang, D. Li and A. Pan, Magnetic Doping Induced Strong Circularly Polarized Light Emission and Detection in 2D Layered Halide Perovskite, Adv. Opt. Mater., 2022, 10, 2200183 CrossRef CAS.
- W. Ning, J. Bao, Y. Puttisong, F. Moro, L. Kobera, S. Shimono, L. Wang, F. Ji, M. Cuartero, S. Kawaguchi, S. Abbrent, H. Ishibashi, R. De Marco, I. A. Bouianova, G. A. Crespo, Y. Kubota, J. Brus, D. Y. Chung, L. Sun, W. M. Chen, M. G. Kanatzidis and F. Gao, Magnetizing lead-free halide double perovskites, Sci. Adv., 2020, 6, eabb5381 CrossRef CAS PubMed.
|
This journal is © the Partner Organisations 2024 |