DOI:
10.1039/D3QM00210A
(Review Article)
Mater. Chem. Front., 2023,
7, 3524-3542
The pivotal role of non-covalent interactions in single-molecule charge transport
Received
27th February 2023
, Accepted 27th April 2023
First published on 2nd May 2023
Abstract
Integrating multidisciplinary efforts from physics, chemistry, biology, and materials science, the field of single-molecule electronics has witnessed remarkable progress over the past two decades thanks to the development of single-molecule junction techniques. To date, researchers have interrogated charge transport across a broad spectrum of single molecules. While the electrical properties of covalently linked molecules have been extensively investigated, the impact of non-covalent interactions has only started to garner increasing attention in recent years. Undoubtedly, a deep understanding of both covalent and non-covalent interactions is imperative to expand the functionality and scalability of molecular-scale devices with the potential of using molecules as active components in various applications. In this review, we survey recent advances in probing how non-covalent interactions affect electron transmission through single molecules using single-molecule junction techniques. We concentrate on understanding the role of several key non-covalent interactions, including π–π and σ–σ stacking, hydrogen bonding, host–guest interactions, charge transfer complexation, and mechanically interlocked molecules. We aim to provide molecular-level insights into the structure–property relations of molecular junctions that feature these interactions from both experimental and theoretical perspectives.
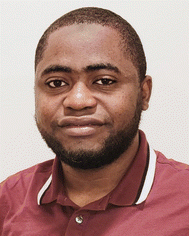
Ridwan Tobi Ayinla
| Ridwan Tobi Ayinla received a Bachelor of Physics (summa cum laude) in 2015 at Ladoke Akintola University of Tech, Nigeria and Master's degree in Applied Sciences in 2019 at University Teknologi Petronas, Malaysia. He was then awarded PTDF to University of Grenoble, France for M2 in 2020. He is presently a doctoral student at the Department of Chemistry, Mississippi State University where his research combines the fabrication of 2D plasmonic nanomaterials and understanding chemical reactions at the single molecular level. |
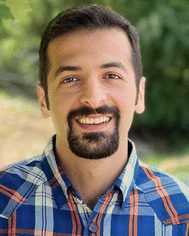
Mehrdad Shiri
| Mehrdad Shiri Received his master's degree from department of Electrical & Computer Engineering, University of Tabriz. Currently, he is pursuing his PhD degree under the supervision of Dr Kun Wang at Mississippi State University. His research interests are in the field of molecular optoelectronics, with a main focus on plasmonic phenomena in molecular junctions. |
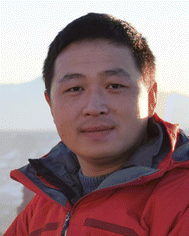
Bo Song
| Dr. Bo Song received a bachelor's degree from Zhejiang University majoring in biomedical engineering in 2011. After obtaining a master's degree in chemical engineering from Lanzhou University in 2015, he went to the United States and started his PhD studies at the University of South Florida, where he obtained his PhD in December 2019. During that time, he mainly focused on coordination-driven self-assemblies and mass spectrometric characterizations. He is currently a postdoctoral fellow at Northwestern University under the supervision of Prof. Sir Fraser Stoddart. His current research focuses on redox-controlled molecular machines and related functional materials. |
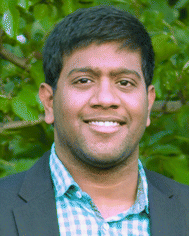
Mahesh Gangishetty
| Dr. Mahesh Gangishetty is currently an Assistant Professor in the Chemistry Department at Mississippi State University. He received his MSc from IIT-Roorkee. Then he moved to the University of Saskatchewan, Canada in 2012 to do his PhD. His PhD was focused on solar energy harvesting for photovoltaics and photocatalysis. After finishing his PhD in 2017, he moved to Harvard University, Rowland Institute, for his postdoc on excitonic materials and devices. His research is primarily centered on Optoelectronic Materials and Devices for energy conversion applications. |
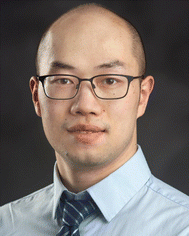
Kun Wang
| Dr. Kun Wang is currently an Assistant Professor in Physics and Chemistry at Mississippi State University (USA). He received a BS in Physics from Shandong University in China in 2011, and a PhD in Physics from the University of Georgia in Athens, GA (USA) in 2016. Prior to joining Mississippi State University, he worked as a Research Fellow at the University of Michigan (USA) from 2017–2020. His research group focuses on exploring intriguing optoelectronics, quantum transport, and energy conversion phenomena at the atomic and molecular scale using scanning probe microscopy-based techniques. |
Introduction
Understanding charge transport through a single molecule, the central mission of single-molecule electronics (SME), is key to the development of a variety of novel nanotechnologies for computing, optoelectronics, energy harvesting, and chemical/bio-sensing. The idea of using individual molecules as active units in nanoscale devices was originally motivated by the exhaustive functionalities of molecules and robust molecular synthetic flexibility.1,2 Over the past two decades, one of the major focuses in the field has been to interrogate how single molecule charge transport responds to different environmental conditions, including electrical, structural, optical, mechanical, magnetic, and thermal stimuli.3–5 Along this line, collaborative efforts between experimentalists and theorists have led to the successful creation of various single-molecule devices that mimic the conventional solid-state devices,6,7 such as conductive wires,8–11 diodes,12–15 switches,16–19 transistors,20–23 and memory devices.24–26 This remarkable progress was made possible thanks to the development of nanofabrication-based methods in the early days and later the invention of the now mainstream single-molecule junction (SMJ)-based techniques, including scanning tunneling microscope break junction (STM-BJ),27–31 mechanically controlled break junction (MCBJ),32,33 conductive atomic force microscope break junction (CAFM-BJ),34–37 electromigration break junction (EBJ),38–40 and graphene–molecule–graphene junction (GMGJ).41–44 These SMJ techniques allow one to trap a single molecule between two conductive electrodes to form an electrode–molecule–electrode junction reliably and repeatably.45,46 Researchers are also able to establish concrete charge transport mechanisms for different categories of molecules driven by careful conversion of chemical information to electrical signals in both theoretical and experimental observations.3,47 So far, extensive results from SMJ measurements have indicated that charge transport through single molecules is fundamentally determined by the nature of bonds/interaction(s) in the molecules.48,49
Covalent and non-covalent interactions can be distinguished by the nature and origin of their bonds. Covalent bonds involve the hybridization of partially filled orbitals of interacting atoms consisting of a shared pair of electrons.50 Therefore, they are considered short-range (<3 Å) and strong. On the other hand, non-covalent interactions are usually long-range (several angstroms), and do not require the sharing of electrons.51,52 Molecular design principles and synthesis strategies that harness both the covalent and non-covalent interactions have offered vast opportunities to create more complex molecular structures and functionalities, which could potentially expand the breadth of applications and scalability of molecular-scale devices. Experimentally, a majority of past SMJ studies have focused on exploring covalently bonded molecules, like organic homologous series,53–55 organometallics,56–58etc. It is only very recently that molecular species involving non-covalent interactions have started to garner increasing attention.59 This is catalyzed by several reasons: (i) the rapid advances in the fields of supramolecular chemistry and molecular machines have enabled the integration of molecular systems with carefully tailored non-covalent interactions into the SMJ setup;60 (ii) the technical development of robust SMJ characterization protocols, e.g. flicker noise analysis and time-dependent dynamics, have made it possible to detect weak non-covalent interactions that are otherwise challenging using conventional techniques;61 (iii) non-covalent interaction is quite ubiquitous as it can be found in natural biosystems, such as peptides, proteins, and DNA, and electrically recognizing such interaction with high sensitivity holds promise for transforming next generation bioelectronics and biosensing technologies;62,63 (iv) harnessing self-assembly processes driven by non-covalent interactions between multiple molecules or sub-molecular components represents a promising route for scaling up future molecular-scale devices.
In general, non-covalent interaction is a collection of intermolecular binding forces consisting of halogen bonds, London dispersions, hydrogen bonds, steric repulsion, electrostatic interactions, stacking interactions, and dipole–dipole interactions, which governs multiple chemical and biochemical processes, such as DNA base pairing, protein–drug interactions, molecule–substrate interaction, and self-assembly complexation of supramolecules.64,65 Over the years, key accomplishments have been achieved in the synthetic protocols of non-covalent molecules. Also, the high degree of reversibility and flexibility enables the tunability of non-covalent molecules using several external stimuli (e.g., light, temperature, chemical environment, electric field, molecular density, and mechanical force).66 Despite the flourish in the synthesis of exotic non-covalently interacting molecular and supramolecular structures, knowledge about how these non-covalent interactions affect charge transport through single molecules remains to be developed.59 In this review, we aim to survey recent advances in probing charge transport through single molecule systems containing non-covalent interactions. We focus our attention on elucidating the impact of a range of non-covalent interactions on the resulting electrical properties of the SMJ from both experimental and theoretical perspectives. Fig. 1 highlights the key interactions that will be discussed. The rest of this article is categorized into five sections: π–π and σ–σ stacking interactions, hydrogen bonding, host–guest interactions, charge transfer complexation, and mechanical bonds (present in mechanically interlocked molecules (MIMs)). The first section focuses on SMJs featuring π–π and σ–σ stacking effects in the transport pathway. In the second section, we discuss the impact of hydrogen bonding on charge transport through SMJs. In the third section, we highlight charge transport in a variety of host–guest configurations and how the interaction between the host and guest molecules or components alters the molecular junction conductance. The fourth section summarizes the donor–acceptor charge transfer complexation and its potential to tune conductance at the single molecular level. In the fifth section, we examine the rarely studied mechanical bonds in MIMs. Finally, we present potential new opportunities of employing non-covalent interactions in the design and realization of desired molecular functionality in future molecular-scale electronics and sensing applications.
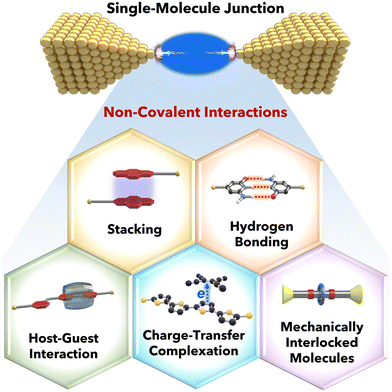 |
| Fig. 1 Schematic of different non-covalent interactions featured in a single-molecule junction. | |
π–π and σ–σ stacking
The primary means of investigating charge transport in aromatic molecular systems is to capitalize on the covalent intramolecular π-electron delocalization that exists in molecular cores where charge transport is “through-bond”. The emergence of novel experimental designs and extreme flexibility displayed in molecular synthesis have introduced yet another unique charge transport medium – “through-space”: where charge transport is dominated by non-covalent, intermolecular delocalization of proximate π-electrons of adjacently stacked aromatic units.66,67 π–π stacked molecules have great potential in advancing molecular electronics because they represent one unique way to simultaneously harness multiple conducting channels (through-bond and through-space) to aid molecular conductance at the single molecular level.46,68,69 Naturally inspired, π–π stacking is also popular for providing desirable stability to biomolecules such as DNA and tertiary protein molecules.70 Recently, the rarely studied σ–σ stacking was also reported to have comparable conductance with π–π stacked molecules.71 Therefore, understanding the detailed mechanism of charge transport in π–π and σ–σ stacking is crucial to advancing the field of molecular electronics. Experimentally, π–π or σ–σ stacking can be realized using different approaches: (i) intra-molecular π–π stacking: a situation where a single molecular core is designed to stack π-electrons of two or more adjacent aromatic rings,72,73 (ii) π–π stacking foldamer: careful mechanical modulation enables researchers to repeatably fold a single-molecule in situ, aiding the stacking of π-delocalized aromatic rings within the same molecular core,74 and (iii) stacked dimer: two identical and individual molecules (having a single linker to covalently bond to an electrode) are brought close to each other to form π–π or σ–σ stacking.68 For instance, Frisenda et al. used the MCBJ method to experimentally show the tunability of destructive quantum interference along the breaking tracks of π-stacked dimers of oligo-phenylene-ethynylene (OPE) (Fig. 2a).75 They investigated three-unit ringed OPE3 with sulfur anchors on one (S-OPE3) and both ends (S-OPE3-S). Theoretical and experimental results of S-OPE3-S are in good agreement with previously reported data with a clear and single-stage conductance plateau around 10−4G0 (Fig. 2b right panel). Interestingly, the theoretical results of S-OPE3 showed a strong quasiperiodic conductance that is dependent on the π-stacking geometry. Experimental data of S-OPE3 showed two stages of conductance at 10−5G0 (1 nm) and 10−7G0 (1.7 nm) which corroborate the theoretical findings (Fig. 2b left panel). Since the S-OPE3 can only connect to the electrode on one end, it is safe to conclude that any observable phenomena are inherently sponsored by non-covalent interaction between π-electrons of the aromatic rings of the molecules. The result of their experiment demonstrated the possibility of tuning quantum interference (QI) in weak non-covalent interactions. Li et al. controlled QI and conductance by switching connection sites of π-stacked anthanthrene using STM-BJ (Fig. 2c).76 Contrary to the similar and previously reported studies where monomer units have higher conductance as compared to the conductance of π-stacked dimers,68,77,78 they reported counterintuitive non-classical room temperature constructive QI (originating from anchor site tuning) whose monomer (M) conductance is 25 times lower than the conductance of the π-stacked dimer (D) (Fig. 2d). Their results indicated that anchor groups and connection sites are key parameters that can effectively manipulate QI and the inherent conductance of non-covalent molecules. The quest to manipulate π–π stacking in situ spurred Stefani et al. to carefully design a spring-like single molecule with an intramolecular π–π stacking core to imitate the less probably intermolecular π–π stacking.79 In their experiment, they used MCBJ to manipulate π–π stacking in a carefully designed [2.2]paracyclophane (consisting of two stacked benzene rings, mechanically stabilized by two non-conjugated linkers) single molecule at room temperature. They reported one order of magnitude conductance modulation due to shear displacement of the π–π stacked core of the mechanosensitive molecule. Contrary to the conventional intermolecular approach to probing π–π stacking, their work suggests an alternative means to design and manipulate π–π stacking with precise control.
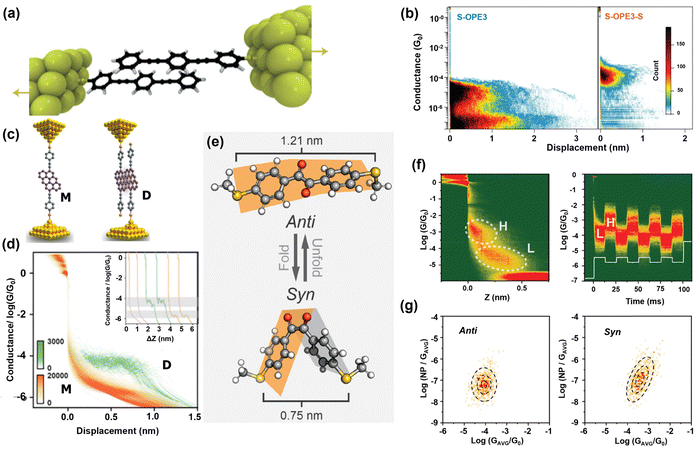 |
| Fig. 2 (a) Schematic of S-OPE molecules connected to one Au-electrode, each dimerizing through π–π stacking. (b) Two-dimensional conductance histogram of dimerized S-OPE (left) and single S-OPE-S (right) connected to both electrodes. Reprinted with permission of ref. 75. Copyright 2016 Springer Nature. (c) Schematic of anthanthrene monomer bonded to two Au-electrodes (left) and single molecules of anthanthrene bonded to one and separate electrodes dimerized via π–π stacking (right). (d) Two-dimensional conductance histogram of anthanthrene monomer (red) and dimer (green). Reprinted with permission of ref. 76. Copyright 2022 American Chemical Society. (e) Three-dimensional reversible structural configurations of diketone flanked phenyl rings. (f) Two-dimensional conductance–displacement map of diketone flanked phenyl rings showing high (syn) and low (anti) conductance states (left). Two-dimensional density map of mechanically reversible conductance switch of diketone flanked phenyl rings (right). (g) Noise power heat maps of anti (left) and syn (right) conformation. Reprinted with permission of ref. 81. Copyright 2020 American Chemical Society. | |
Inspired by an early theoretical study of Franco et al.80 that predicted the possibility of mechanically folding a propyl chain to switch the conductance on and off, Wu et al. presented an experimental report of a mechanically responsive single molecule of diketone chain flanked by two phenyl rings.81 They took advantage of the conformational flexibility that exists between the carbonyl groups to mechanically fold (syn) and unfold (anti) the molecule using STM-BJ (Fig. 2e). The anti-conformation behaves like a sheet with lower conductance while the syn-conformation brings the two phenyl rings close enough to establish π–π stacking with higher conductance (Fig. 2f left). Relying on mechanical modulation of the stable junction, they achieved a reversible conductance switch between the anti (low) and syn (high) conformations (Fig. 2f right). To establish the origin of the rise in conductance observed in the syn-conformation, they conducted a flicker noise analysis and showed that the noise power of the anti-conformation is independent of conductance, which depicts that charge transport is through-bond. However, the noise power of the syn-conformation is strongly dependent on the conductance, which revealed that through-space is contributing to charge transport (Fig. 2g). The result of their experiment showed that π–π stacking can be achieved using molecular functionalities to induce mechanoresistivity.
Recently, Feng et al. introduced σ–σ stacking at the interplay of nanostructured electrodes using STM-BJ.71 They employed careful experimental design to study and compare charge transport in unsaturated benzenethiol, saturated cyclohexanethiol, and 1-adamantanethiol with one anchor (Fig. 3a). The benzene ring showed two conductance (high and low) plateaus corresponding to charge transport through π–π stacking of adjacent and proximate benzene rings (Fig. 3b left). Surprisingly, similar and comparable conductance plateaus (high and low) were reported for the unsaturated rings, which denote charge transport through σ–σ stacked rings of cyclohexanes (Fig. 3b right). The high and low conductance plateaus were associated with different configurations of the stacked rings. In the case of saturated adamantane, three conductance plateaus (high, medium, and low) relating to charge transport through one monomer of adamantane and two different σ–σ stacking orientations were reported (Fig. 3c). To further establish the nature of charge transport in high, medium, and low configurations, they conducted flicker noise analysis for the three conductance scenarios. In general, the flicker noise power (NP) is proportional to the nth (scaling indicator of electronic coupling) power of conductance (G) i.e., NP is proportional to Gn. When n = 1.0, the charge transport is said to be through-bond. When n = 2.0, charge transport is though space. In their flicker noise analysis, they obtained scaling indicators to be 1.8 or 2 for 1-adamantanethiol, benzenthiol, and cyclohexane, which suggests that the dominant transport in the junctions is through space (Fig. 3d). Their results further establish the relevance of non-covalent interactions through the rarely studied σ–σ stacking.
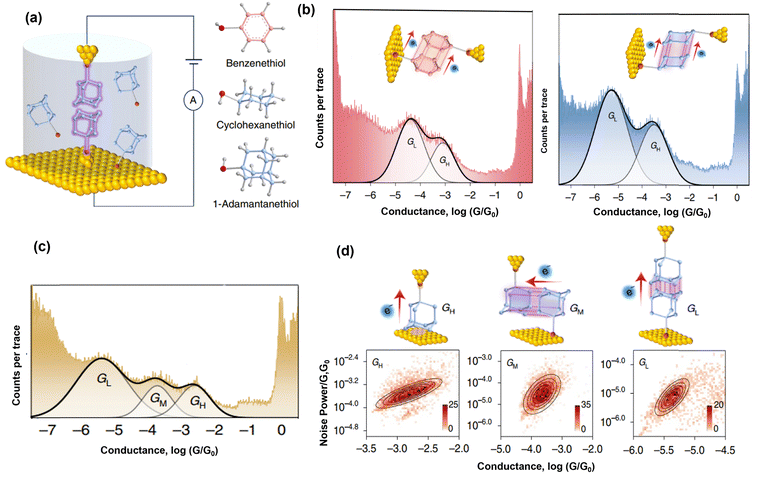 |
| Fig. 3 (a) Schematic of σ–σ stacking in STM-BJ and typical structures of benzenethiol, cyclohexanethiol, and 1-adamantanethiol. (b) One-dimensional conductance histogram of benzenethiol (left) and cyclohexane (right). (c) One-dimensional histogram of 1-adamantane showing high, medium, and low conductance plateaus. (d) Schematics of three charge transport configurations of 1-adamantane (top) with their corresponding flicker noise profile (bottom). Reprinted with permission of ref. 71. Copyright 2022 Springer Nature. | |
Hydrogen bonding
Hydrogen bonds (a short range and weak electrostatic force of attraction that exists between hydrogen atoms which are covalently bonded to a “donor” – a more electronegative atom or group and another “acceptor” – another electronegative atom/group having a lone pair of electrons) represent a unique and ubiquitous form of non-covalent interaction that hold several natural relevancies. Many naturally occurring biological, chemical, and physical processes rely on hydrogen bonds for stability. Therefore, understanding the details of charge transport in hydrogen bonds at the single molecule level could potentially impact the broad fields of supramolecular chemistry, molecular electronics, and biosensing. Nishino et al. functionalized both the tip and substrate with ω-carboxyl alkanethiols and measured charge transport through hydrogen bonds between two individual molecules using STM-BJ.82 They observed that although the electronic conductance of the hydrogen bond decays rapidly as the chain length of the hydrogen bonded molecules increases, it conducts better than a covalent σ-bond. Their work implies that hydrogen bonds can independently mediate charge transport with non-trivial transmission efficiency.
Solvent and temperature are two important parameters that have been determined to influence the formation and strength of hydrogen bonds.66,83 Specifically, protic (proton donor) solvents are known for breaking hydrogen bonds due to the formation of more intermolecular interactions.84 Ge et al. took advantage of protic solvents to induce electrostatic gating and hydrogen bond formation using STM-BJ.85 They interrogated the possibility of using protic solvent to tune hydrogen bond-mediated charge transport and even switch between destructive quantum interference (DQI) and constructive quantum interference (CQI) in a SMJ. Specifically, they used zwitterionic (BIT-Z) and neutral (BIT-N) forms of [2,2′-Bi-1H-indene]-3,3′-dihydroxy-1,1-dione (BIT-OH2) because theoretical calculations predicted that in the absence of solvents charge transport through BIT-Z and BIT-N is controlled by DQI and CQI, respectively. 1,2,4-Trichlorobenzene (TCB) (aprotic) and ethanol mixed with propylene carbonate (PC:EtOH) (protic) solvents were used to tailor the conductance of the target molecules (Fig. 4a). BIT-Z showed a single-stage conductance plateau in TCB around 10−3.67G0 (Fig. 4bi) while PC:EtOH showed a lower conductance plateau at 10−3.96G0 (Fig. 4bii). These results showed that the conductance of BIT-Z in TCB is higher compared to that of PC:EtOH. However, the conductance of the BIT-N form changed from 10−4.39 to 10−3.96G0 when the solvent changed from TCB to PC:EtOH. The change in conductance of the neutral form of the sample in a protic solvent was associated with the changes from DQI to CQI resulting from the conformational change from planar to folded. Their work highlighted the impact of solvents on manipulating QI associated with hydrogen bonds. Zhou et al. used GMGJ to investigate the effect of solvent on hydrogen bonding dynamics in ureido pyrimidine at different temperatures.86 They measured the current profile of hydrogen bond formation in 1,1,2,2-tetrachloroethane (TCE) and diphenyl ether (DPE) solvent at temperatures: 273 K, 293 K, 313 K, and 323 K, 333 K, 343 K, respectively. The current profile in TCE shows similar and bimodal current with large amplitude at all temperatures (Fig. 4c(i–iii)). In contrast, the current profile of DPE solvent shows multimodal distribution (Fig. 4d(i–iii)). As the temperature increases, the frequency of hydrogen bond formation increases. The different conductance spikes were attributed to distinct solvent-dependent intermolecular hydrogen bonding mechanisms at different temperatures. Their work established the dominant role of temperature and solvent in the formation dynamics of hydrogen bonds at the single molecule level.
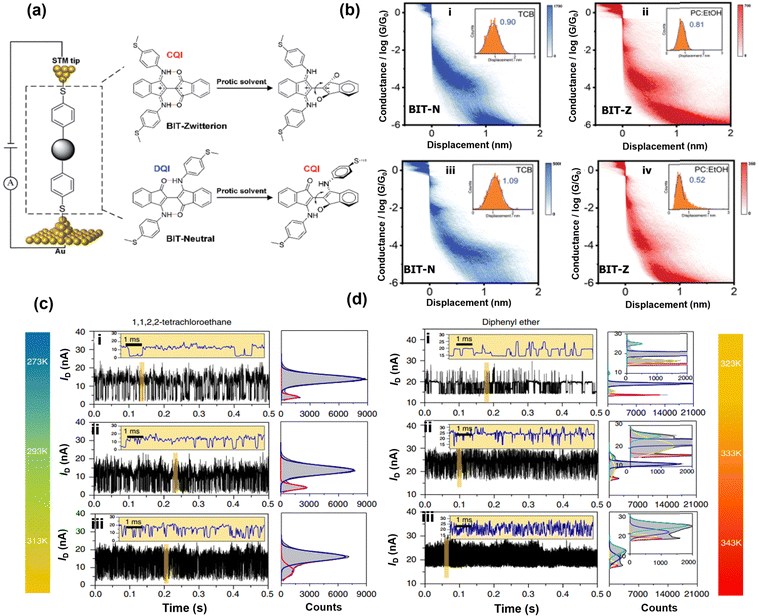 |
| Fig. 4 (a) Schematic of BIT isomers (neutral (N) and zwitterion (Z)) sandwiched between STM-BJ electrodes. (b) Two-dimensional conductance–displacement histograms of (i) BIT-Z in aprotic TCB, (ii) BIT-Z in protic PC:EtOH, (iii) BIT-N in aprotic TCB and (iv) BIT-N in protic EtOH. Reprinted with permission of ref. 85. Copyright 2022 Royal Society of Chemistry (c) temperature and solvent-dependent current–time profile of ureido pyrimidine in (c) 1,1,2,2-tetrachloromethane at (i) 273 K, (ii) 293 K and (iii) 313 K, and (d) diphenyl ether at (i) 323 K, (ii) 333 K and (iii) 343 K. Reprinted with permission of ref. 86. Copyright 2018 Springer Nature. | |
In another experiment, Chang et al. used STM-BJ to examine the strength of three hydrogen bonds in guanine–cytosine (G–C) and two hydrogen bonds in adenine–thymine (A–T) DNA base pairs.87 Their results showed that the triple hydrogen bond contact in G–C was stronger than the double bond contact of A–T. They associated weaker attractive interactions with transient hydrogen bonds and used signature sensitivity to differentiate hydrogen bonds from Au–S bonds. Their work demonstrated the possibility of quantifying the strength of weak hydrogen bonds that are otherwise impossible to quantify. Although hydrogen bonds are often considered weak, their response to external stimuli can have a non-trivial influence on the electronic properties of molecules. The above-mentioned studies clearly imply that one can take full advantage of hydrogen bonds in the future design of molecular electronic materials.
Host–guest interaction
Host–guest interaction is an aspect of non-covalent interaction that involves the complexation of two or more molecules or ions transiently or permanently. The increasing complexity of supramolecules comprising host and guest molecules has been harnessed in developing new computing technologies.20,88–92 Therefore, a deep understanding of electron propagation, pathways, and mechanisms at the single molecule level in host–guest structures can enhance our understanding of the intrinsic nature of these molecules and, by extension, suggest a more promising way to design single supramolecular electronic and sensing components. A single molecule scale channel is associated with pronounced quantum effects meaning a higher degree of freedom to manipulate charge transport. Therefore, this has led researchers to investigate quantum rings, known as conjugated macrocycles, that can introduce multichannel electronic pathways taking advantage of the wave nature of electrons.
Host–guest systems have enabled more flexibility to not only deliberately perturb the electron transmission channels on the macrocycle but also introduce new charge transport pathways. Host–guest complexation/decomplexation has also been applied in various disciplines, including biosciences,93–96 nanosciences97–99 and molecular machines.100–102 Interestingly, single-molecule label-free investigation of reaction dynamics has benefited from accurate temporal information collected in host–guest complexation at the single molecule level, namely molecular recognition, which is crucial to biological functionalities. However, controlling and monitoring the dynamic processes is still challenging due to the complexity of host–guest configurations and the interdependence of different components within the system.103–106 Experimentally, three different configurations have been developed to probe the charge transport and dynamics of a molecule hosting a guest(s) in an SMJ setup.
Firstly, the host molecule (usually a macrocycle cavity) bridges two electrodes while accommodating the guest. The precise control over the size of the cavity provides enough affinity/driving force for complexation,104 consequently resulting in a considerable change in the charge transport properties of the host backbone. In the second experimental approach, the guest molecule is typically terminated by anchoring groups at both ends to complete the contact with the two electrodes. Upon encircling the guest backbone by a ring-like host, the conductance could vary owing to non-covalent interactions inside the cavity.107,108 Apart from a change in the conductance, introducing the host molecule can screen electrostatic repulsions and suppress the intermolecular interactions, giving a well-defined conductance feature consistent with the length of the guest.109 In the last scenario, availing the stability of the molecular junction in GMGJ,110 a molecular wire equipped with a pendant ring can convert the stochastic and dynamic behavior of the guest into electric signals. This platform has enabled direct real-time measurements at single-molecule sensitivity levels,111 which is absent in other detection methods, such as nuclear magnetic resonance (NMR) and UV-vis spectroscopy or labeling techniques.112–114
Host–guest interaction has also been studied through the mentioned experimental approaches by investigating charge transport in a macrocycle when incorporating the guest inside its cavity. The metallocyclic molecule, made by coordination-driven self-assembly, allowed Tang et al. to have precise control over the shape and size of the host cavity incorporating the C60 guest molecule.104 In this work, they synthesized three different host organoplatinum(II) metallocycle molecules with different cavity sizes (Fig. 5a). After the addition of C60, the STM-BJ conductance measurements revealed a new conductance plateau one order of magnitude higher in the two host molecules with a smaller cavity size of 10.84 and 14.10 Å for molecules 1 and 2, respectively (Fig. 5b), while no change was observed in the molecule with the largest cavity [3] with cavity size of 22.85 Å. These results indicate that only host molecules 1 and 2 can interact with C60 due to the suitable size match. The representative current–distance trace in Fig. 5b shows that the conductance switches from high to low upon molecule elongation. This switching behavior was attributed to the release of the guest during the mechanical stretching. The results and control experiments elucidated that host–guest formation is due to donor–acceptor Coulombic interaction between the electron-rich metallocyclic skeleton and electron-deficient C60.
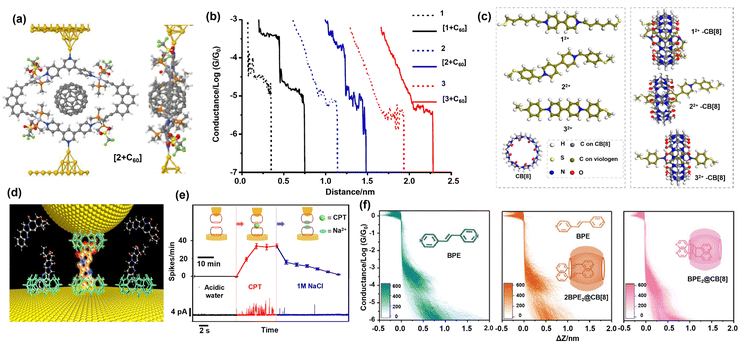 |
| Fig. 5 (a) Schematic illustrations of the geometrically optimized host–guest system upon formation of a single molecular junction. (b) Representative conductance traces as a function of distance obtained for 1,2,3, [1-C60], [2-C60] and [3-C60]. Reprinted with permission of ref. 104. Copyright 2019 Springer Nature. (c) Chemical structure of CB[8] and viologen without counterions. Reprinted with permission of ref. 106. Copyright 2016 American Chemical Society. (d) Schematic of a trapped molecule (CPT) between the tip and substrate both functionalized with CB[7]. (e) Time-series current measurement of drug molecule CPT during the formation of a CB7-CPTH + -CB7 and, then the releasing process by adding Na+ ions. Reprinted with permission of ref. 125. Copyright 2022 Wiley. (f) 2D histogram of conductance versus displacement for BPE and the mixture of BPE2@CB[8]. Reprinted with permission of ref. 131. Copyright 2022 Wiley. | |
In the following configuration, the guest molecule is threaded inside a ring-like host and completes the circuit by anchoring to both electrodes. In this regard, Zhang et al. have studied viologen (bipyridinium) wire when it is threaded into the cucurbit[8]uril (CB[8]) cavity.106 As shown in Fig. 5c, they immobilized the three different viologen-CB[8] host–guest systems on the gold surface to form single-molecule junctions. Numerous studies before,105,107,108,115–117 have shown that the dipole–dipole and hydrophobic interaction between these two molecules is responsible for high inclusion constants. Statistical results derived from STM-BJ measurements showed that changing the environment of the viologen by CB[8] leads to a noticeable conductance increase as high as three-fold. Furthermore, based on the positioning of the bipyridinium moiety inside the CB[8], different conductance states were observed. Controlling the environment through electrochemical gating further showed that the noticeable conductance change results from a change in the reorganization energies of the outer sphere.
As a ubiquitous type of interaction that has garnered attention recently, cation–π interaction plays a significant role in molecular aggregation and supramolecular assembly.118–123 The electrostatic interaction between the negatively charged π system and positively charged cation can give rise to cation–π interaction as a relatively strong non-covalent interaction. Wang et al. have studied the influence of cation–π interaction on the charge transport properties of the viologen derivative in a host–guest system.124 In their supramolecular compound, the interaction takes place between the π system of the pillar[5]arene ring and the positively charged core of VSMe (1,1′-bis(4-(methylthio)phenyl)-[4,4′-bipyridine]-1,1′-diium hexafluorophosphate). They observed that the complexation resulted in a two-fold conductance enhancement, which was attributed to the HOMO–LUMO narrowing and planarization of the VSMe component induced by the cation–π interaction.
The recognition tunneling technique has also been carried out using STM in which CB[7] was used to functionalized both contacts as host molecules. Xiao et al. identified drug molecules such as camptothecin (CPT) and their conductance response to pH and concentration variation by monitoring the current through the guest molecules trapped within two CB[7] cavities (Fig. 5d).125 The molecular interconversions are determining factors in obtaining the physiologically active form in solutions with different pH. Therefore, temporally resolved information about such molecular conversions can deepen our understanding of the physical properties at a particular molecular state, which conventional techniques cannot achieve. It was shown that the frequency of the tunneling recognition signal could identify the pH-sensitive molecular state. Furthermore, they studied the effects of external stimuli on complexation. Increasing the concentration of NaCl in the solution could significantly inhibit the host–guest formation by displacing the CPT from the junction (Fig. 5e). However, the non-covalent interactions upon encapsulation of the guest molecule do not always lead to a considerable molecule conductance change, as seen in the works mentioned above. These interactions have also proven advantageous when the molecule insulation from the environment is of interest. The advantage of this system is two-fold. Firstly, the encapsulation can cause lower aggregation leading to well-defined, less distributed statistical conductance results,126 and secondly, it suppresses the influence of intra and intermolecular interactions.105,109
The application of non-covalent interactions in host–guest systems is not limited to conductance change or isolation. It can also provide a reaction chamber by maintaining space proximity between two individual molecules, and give information about reaction kinetics.127–130 By this means, Yuan et al. showed that single-molecule junctions formed in an STM-BJ setup could provide a testbed to detect temporal signals from reactants in ultra-low concentrations, which are invisible to methods such as mass spectroscopy.131 They used host–guest assembly to study the conductance change during complexation of 1,2-bis(4-pyridinyl)ethylene (BPE) monomer and CB[8], and finally the dimerization product to BPE2@CB[8] upon UV irradiation. As seen in Fig. 5f, the negligible conductance change after encapsulation implies that bulky CB[8] hinders the formation of SMJ. After irradiation for a certain time, the dimerization reaction is complete, and the product exhibited a significant conductance decrease. By host–guest assembly in an SMJ one can maintain multiple molecules in certain proximity giving the opportunity of tracking the dimerization process with reactant concentrations as low as 0.5 × 10−6 M.
In the last experimental approach, the host–guest complex is chemically gated by a pendant covalently bonded to the molecular backbone. Therefore, non-covalent interactions can be monitored in real-time since the guest molecule shuttling can momentarily perturb the carrier density of the conducting pathway, which is reflected as spikes in current. Using rationally designed molecules, the platform can quantify binding energies and recognize the interactions in complex biosystems.46,132 To better understand the current distribution passing through the host molecule in this system, especially when it comes to sensing at the molecular level, a high stability and long lifetime of the junction is necessary. GMGJ has successfully provided the junction stability required for real-time probing of the gating effect and conductance changes under external stimuli.132–135 Taking advantage of such a platform, Wen et al. have established nanogap contacts capable of probing the temporal non-covalent interactions.136 As schematically represented in Fig. 6a, the electrical characterization was performed in a solution consisting of host molecules with a conjugated backbone with an electron-rich bis-p-phenylene[34]crown-10 (BPP34C10) side arm and the electron-deficit methyl viologen (MV2+) as the guest. The bimodal current distribution shown in Fig. 6b and c suggests that the transient complexation increases the conductance. They used the changes in thermodynamic and kinetic parameters to confirm the temperature-dependent pseudorotaxane (de)formation processes. The introduced system by non-invasively monitoring the molecular dynamics has unfolded new opportunities for label-free bio detections, such as DNA sequencing and enzymatic activity.
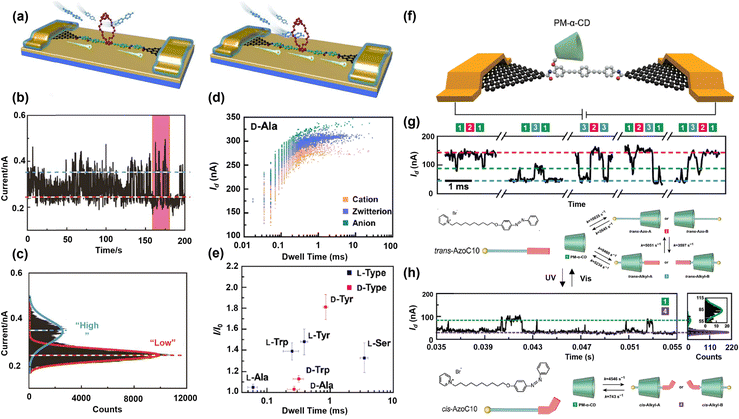 |
| Fig. 6 (a) Schematic representations of the graphene–molecular–graphene junction consisting of (left) BPP34C10, which has a lower conductance, and (right) a more conductive host–guest system when a charged molecule of MV2+ passes through the host's cavity. (b) Measured current as a function of time for BPP34C10-SMJ once it is immersed in a solution containing MV2+, and (c) the corresponding 1D histogram for the current measurement showing bimodal conductance states. Reprinted with permission of ref. 136. Copyright 2016 Science. (d) Current versus dwell time 2D histogram for L-Ala at pH = 7. (e) Conductance variation (I/I0) as a function of dwell time used as enantiomer recognition for various amino acids. I0 is the basic current for empty PM-β-CD. Reprinted with permission of ref. 111. Copyright 2021 Science. (f) Schematic of the GMGJ nanodevice consisting of a photosensitive single supramolecular junction. Current–time signal and molecular structure in (g) trans and (h) cis form under visible light and UV, respectively, showing a reversible binding process. Reprinted with permission of ref. 110. Copyright 2022 Wiley. | |
The same technique has also been adopted by Liu et al. to identify different amino acids by unveiling their dynamics at the single-event level.111 They formed the junction by using a conjugated organic molecule as a rigid molecular backbone and a permethylated-β-cyclodextrin (PM-β-CD) pendant as the recognition chamber. The selection of (PM-β-CD) was rationalized by the fact that the absence of intermolecular hydrogen bonds in this molecule can slow down the recognition process, consequently leading to significant chiral discrimination in the macrocycle.137–141 As shown in Fig. 6d and e, their studies showed that the reaction rate obtained from dwell time and current fluctuations monitored in real-time could be used to recognize each amino acid. Current–time data from the solution containing the chiral molecule along with the kinetic data can resolve the chirality, which promises novel approaches for single-molecule level bio-detection. In another work, Su et al. monitored the stochastic binding dynamics in a system involving a light-sensitive azo compound.110 They used high temporal resolution of current data captured in a GMGJ platform to detect transient states at the single molecule level, which is beyond the limitations of conventional techniques like NMR,114,142 and fluorescence spectroscopy.143 In this work, the azobenzene moiety was utilized as the guest since it could switch reversibly between trans and cis form under irradiation (Fig. 6f). Under low bias I–t measurements, trimodal and bimodal conductance states were observed, which were further attributed to the different binding states at trans and cis conformation, respectively (Fig. 6g and h). The dynamics of the light-sensitive guest molecule threaded inside the host can be easily visualized in real-time and compared in different light-induced motion modes. The host–guest interactions have unfolded single events in a single-supramolecular level by its influence on the charge transport. Supramolecular chemistry can take advantage of this precise control over conductance to deepen and rectify the understanding of host–guest complexation in novel applications such as drug discovery and catalysis.
Charge-transfer complexation
Charge-transfer (CT) complexation refers to a molecular interaction between a donor (electron-rich) molecule and an acceptor (electron deficient) molecule, in which electron(s) are transferred from the donor to the acceptor. Exchanging charges between a donor(s) and an acceptor(s) molecule can create a new supramolecular system with different electronic and physical properties than individual conglomerate molecules. The unique charge transport characteristic in bulk systems consisting of organic CT complexes has been adopted to realize organic materials with metallic behavior and superconductivity.144–147 For instance, electron donor–acceptor complexation of tetrathiofulvalene (TTF):tetracyano-p-quinodimethane (TCNQ) with the ratio of 1
:
1 has exhibited metallic behavior in a wide temperature range.148 An organic conductor consisting of CT salt of di-(tetramethyltetraselenafulvalene)-hexafluorophosphate ([TMTSF]2PF6) has been proven to exhibit superconductivity.149 In general, the effect of CT complexation on charge transport properties has been studied at the single molecular level. Formation of a CT complex by introducing π-acceptors such as tetracyanoethylene (TCNE) or tetrafluoro-tetracyanoquinodimethane (F4TCNQ) to a single molecule junction can considerably enhance the conductance by creating new resonance in the transmission window. Garcia et al. studied the charge transport properties of a molecular wire containing a π-extended tetrathiafulvalene (exTTF) and its CT complex with F4TCNQ.150 Based on their experimental results, the conductance of neutral exTTF was below the measurement limit; however, upon complexation, the CT complex exhibited more than two orders of magnitude higher conductance. The conductance increase was concluded to be owing to the planarization of the molecule once it is oxidized by the acceptor in the CT complex.
Vezzoli et al. reported over an order of magnitude conductance enhancement in an aromatic donor moiety (dialkylterthiophene, dialkylbenzene) through CT complexation with TCNE.151 Theoretical calculations revealed that the high conductance was due to the appearance of Fano resonance close to the Fermi level. To further investigate the evolution and geometry of the CT complex-based molecular junction, Vezzoli et al. used bis(thiaalkyl)arene as a donor molecule to measure charge transport with and without complexation with TCNE.152 The uncomplexed molecule revealed three individual peaks accounting for low, medium, and high range conductance, which was later attributed to different S–Au binding locations. After CT complexation with TCNE, these three peaks were increased by factors of 70, 70, and 46, respectively. Calculations showed that the conductance enhancement is the largest for the configuration with the lowest conductance since the position of the Fano resonance is pinned to the Fermi energy in the CT complex. Moreover, Wang et al. studied the conductance change in thiophene-based molecular wires upon CT complexation with electron-deficient TCNE.153 Using the STM-BJ approach, they reported almost two orders of magnitude in a ∼2 nm long α-quaterthiophene wire after CT complexation. Based on their study, CT complexation can introduce constructive quantum interference in conventional, fully conjugated molecules. Fig. 7a demonstrates the series of thiomethyl-terminated oligothiophenes (T2–T4) used in this study and complexation with TCNE. The change in the energy levels with respect to the electrodes before and after complexation is schematically shown in Fig. 7b. They measured the conductance before and after complexation as shown in Fig. 7c and d. The exponential decay of conductance indicates the off-resonance, length-dependence charge transport of non-complex molecules (T2–T4) with the decay constant of 5 nm−1. Albeit the result of the charge transfer complex exhibits higher conductance with weak length dependence (Fig. 7e). The calculated transmissions shown in Fig. 7f explain the higher conductance at the CT complex as an additional Fano resonance is created. They attributed the Fano resonance to the partly filled orbitals on the molecular backbone and TCNE, acting as a scattering center. These partly filled orbitals associated with the CT complex can also give rise to spin splitting in the transmission profile. A distinct transmission characteristic has been widely sorted for the design of molecular thermoelectric devices that possess a high Seebeck coefficient and figure of merit. This investigation underscores the significance of quantum interference characteristics in the transmission profile, where slight supramolecular interactions can significantly alter the transport behavior of molecular junctions.
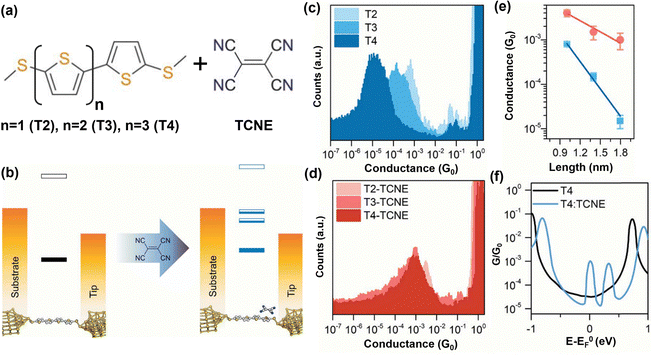 |
| Fig. 7 (a) The chemical structure of the oligothiophene-based molecules used as a molecular wire in the junction (T2–T4), and TCNE. (b) Schematic diagram representing the calculated energy levels of T4 with respect to the electrodes before and after complexation with TCNE. (c) 1D conductance histogram for isolated molecules T2, T3 and T4. (d) 1D conductance histogram for CT complex molecules. (e) The conductance as a function of length for isolated molecules T2 to T4 (blue) and CT complex molecules T2:TCNE to T4:TCNE (red). (f) Calculated transmission function for T4 and T4:TCNE. Reprinted with permission of ref. 153. Copyright 2019 Royal Society of Chemistry. | |
Non-covalent interactions in mechanically interlocked molecules
Mechanically interlocked molecules (MIMs) consist of spatially entangled sub-molecular components such that the final structure is dictated by the topology and architecture of the constituents.154–158 Thanks to the entanglements, multiple molecules that are linked together cannot be separated unless the covalent bond of conjoined molecules is broken. During the past two decades, the flourishing of a large variety of mechanically interlocked molecules has paved the way to the design and construction of artificial molecular machines.159 In general, the non-covalent interactions have been widely explored in two typical MIMs, namely catenanes and rotaxanes, to develop switches,160–163 transistors,164,165 logic gates,166,167 and memories.168 These two kinds of MIMs serve as typical representations of mechanical bonds and have thus attracted attention in the past few decades.168,169 Aside from the distinctive physical and chemical properties, these molecules are able to show switching behavior arising from the displacement of components over different recognition sites by external stimuli.170 This has allowed researchers to monitor the dynamic processes in the systems involving mechanical bonds.
In an oligoyne system (Hexayne) inside a macrocycle mechanically trapped by two 3,5-diphenylpyridine stoppers at both ends, Milan et al. showed that the use of non-covalent interaction in terms of “rotaxation” could make an insulated molecular wire (Fig. 8a).171 They combined sterically shielding end groups with rotaxation (threading the molecule inside a macrocycle) to stabilize the notoriously unstable long oligoyne molecules. STM-BJ measurements demonstrated that the presence of a macrocycle lowered the possibility of forming a single molecule junction, suggesting that the bulky macrocycle hinders different contact geometries. The addition of the macrocycle had a marginal influence on the conductance of the molecular bridge.
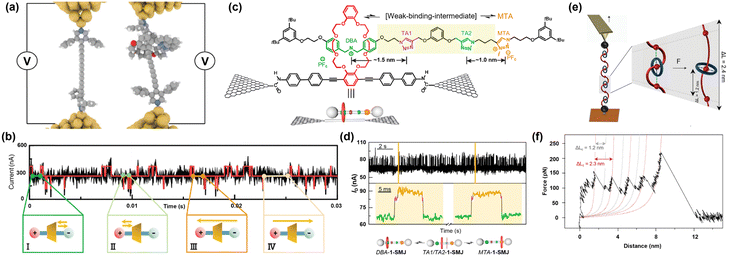 |
| Fig. 8 (a) Schematic representation of conductance measurement in the STM single-molecule junction. (left) Oligoyne guest molecule (right) after rotaxation. Reprinted with permission of ref. 171. Copyright 2017 Royal Society of Chemistry. (b) Current–time measurement based on which the signal is categorized into four possible shuttling scenarios. (I) Two consecutive high-conductance states (II), two consecutive low-conductance states (III), complete shuttling from the high-conductance state to the middle-conductance and then to the low-conductance state and (IV) from the low-conductance state to the middle-conductance and then to the high-conductance state. Reprinted with permission of ref. 172. Copyright 2019 Wiley. (c) Structure of the molecular shuttle threaded inside a crown ether. The axle features MTA and DBA units and two intermediate TA1 and TA2 binding sites. (d) (top) Real-time current measurement conducted for 1-SMJ inside the DMSO environment at 338 K. (bottom) Expanded region of the current–time curve indicated by yellow lines. The intermediate conductance states corresponding to TA1/TA2 binding sites are labeled purple. These short-lived co-conformers occur during transitions of the macrocycle between DBA (green) and MTA (orange). Reprinted with permission of ref. 173. Copyright 2022 Elsevier. (e) Schematic of the cantilever retraction process at a constant velocity. The mechanical elongation leads to the breaking of the non-covalent interaction between a DNP and adjacent ring, and consequently, mechanical unfolding. (f) Force–distance curve recorded at a low speed of 40 nm s−1 demonstrating a sawtooth profile with existing second peaks. Reprinted with permission of ref. 176. Copyright 2018 Springer Nature. | |
Mechanically bonded units can shuttle over different binding sites and change the transmission by local electrostatic gating. In a timescale of a millisecond, Zhou et al. explored the stochastic movement of mechanically interlocked alkyl chains inside a macrocycle.172 They observed bimodal fluctuations in the current, which is highly dependent upon temperature and local charge. This measurement enabled them to monitor the transient shuttling in a rotaxane system for the first time in real-time. A pseudo-rotaxane was formed in solution by threading the alkylene axle inside a permethylated α-cyclodextrin (PM-αCD) macrocycle. The shuttling unit was designed to have positive, negative and amphoteric-charged terminals at both ends to investigate the electrostatic gating effect on the conductive channel providing real-time information about shuttling. In analogy to conventional field effect transistors, the shuttling event can act similarly to electrostatic gating, which changes the charge density distribution on the conducting pathway, leading to a noticeable change in the conductance. As depicted in Fig. 8b(I) and (II), the transitions between two high consecutive conductance states or two consecutive low conductance states imply that the rotaxane is shuttling incompletely around one of the axle ends. The introduced platform offers new opportunities for nanodevices capable of having precise control over biomolecular dynamics.
The measurements of MIMs using SMJ also enabled the studies of molecular machines at the single molecule level. Recently, while studying the dynamics of MIMs shuttling in GMGJ with a high temporal resolution, Chen et al. detected weak intermediate binding states between the triazole units on the axle and the crown ether.173 As shown in Fig. 8c, the rotaxane formation was established once the two ends of the axle were terminated by a relatively large molecule of 3,5-di-tert-butylbenzyl groups, blocking the further dethreading of the macrocycle from the axle. As the ring resides on two major equilibrium sites of the dibenzylammonium motif (DBA) and methyl triazolium group (MTA) with different affinities, the current fluctuates between high and low conductance states with different lifetimes. Besides, various parameters, such as shuttling kinetics and thermodynamics, were investigated in different solvents and temperatures. Although DMSO is a strong hydrogen-bond-disrupting solvent, it is weakly able to solvate crown ether while the ring moves over hydrogen-binding sites, resulting in a slow shuttling rate compared to that in acetonitrile. Slowing down the process allowed them to identify the intermediate weak binding states, which happen when the ring resides over triazole units TA1 and TA2 (Fig. 8d). Revealing the weak transient states could be used to understand the effects of each component in a rotaxation process, making this system preferable to the techniques with time-averaged results.
Intermolecular interactions in MIMs have also been studied in terms of their mechanochemical properties.174,175 Sluysmans et al. took advantage of the exquisite complexity of mechanically interlocked rotaxanes and catenanes to unveil the mechanochemical properties of oligorotaxane foldamers using an atomic force microscope.176 Their system consists of [5] rotaxane with dithiolane ends and serpentine flexible dumbbell featuring 1,5-dioxynaphthalene (DNP) (donor) threading inside four cyclobis(paraquat-p-phenylene) (CBPQT4+) (acceptor) rings (Fig. 8e). Owing to multiple π-stacked donor–acceptor interactions, the molecule converts to a secondary folded structure. Molecular dynamics simulations revealed that although the entropy works against the folding, the molecule tends to fold due to the dominating energetic contributions. The equally spaced peaks in the sawtooth-like force–distance curve (Fig. 8f) were explained based on the sequential rupture of the intermolecular interactions between the ring and the DNP balls on their sides. They also observed rapid fluctuations during the pulling and relaxation process, which implies that immediately after breaking, the molecule tends to reform the broken bond again and exerts an opposite force. Their results show that oligorotaxanes have the potential to outperform the natural folding proteins.
Conclusion and outlook
Before a perfect marriage between structure and functionality can be achieved in molecular electronics, a thorough understanding of the role of non-covalent interactions in single-molecule charge transport is imperative and requires increasingly collaborative efforts from researchers specialized in molecular design and synthesis, experimental characterization, and theoretical simulation and prediction. As discussed above, recent research has evidently revealed the unimaginable promise of non-covalent interactions in tuning charge transport in a variety of molecules.50,59,66 The remarkable development of supramolecular chemistry has enabled the synthesis of hundreds, if not thousands, of amazingly complex molecular structures through self-assembly as a result of the elegant mixture of covalent and non-covalent interactions. It has been well accepted that harnessing molecular self-assembly might be the most promising route to circumvent the scalability issue in future molecular-scale devices. There is little doubt that now is the perfect time to examine many of these emerging supramolecular systems with the well-developed SMJ techniques. We note that an important step towards making key progress in this direction is to foster close and continuous communication between different counterparts involved: namely, chemists and materials scientists need to design and synthesize molecules suitable for SMJ measurements; experimentalists should attempt to enrich the information that can be detected in an SMJ and, hopefully, collect electrical, mechanical, optical, and chemical signals simultaneously with high temporal resolution; equally importantly, theoreticians need to develop models that can explain experimental observations quantitatively under the real measurement conditions, and ideally, make reliable predictions and suggest future directions.
What can we do with non-covalent interactions? Before one can give a concrete answer to this, the field should focus on addressing several existing hurdles and open questions ahead. First, can the contribution of a specific non-covalent interaction in a molecular bridge be isolated in an SMJ measurement? This requires careful molecular design and delicate experimental characterization, such as creating an SMJ in which non-covalent interactions are on the dominant transport pathway, instead of on the side chain or acting as a perturbation to the main covalent conduction channel. Then, similar to how the covalent molecules were studied, one needs to understand how charge transport through such non-covalent pathways responds to different external stimuli, such as light, heat, electric field, magnetic field, and electrochemical environment. Second, built upon the knowledge of traditional covalent molecular wires, we should develop strategies to harness the interplay of the coexisting covalent and non-covalent interactions in a molecular bridge. In this regard, there are already several excellent candidates worth investigating immediately, including supramolecules, MIMs (e.g., molecular knots), and biomolecules (e.g., DNA, peptides, and proteins). A potential challenge one may face is: how to extract universally applicable physical and chemical insights from data obtained using different experimental platforms and how to utilize these insights to better the design of next generation molecular systems and experimental methods? Third, can a clever use of non-covalent interactions resolve the thorny issues researchers have been dealing with in covalent molecular wires? For instance, could appropriate non-covalent interactions help to enhance the performance of a molecular rectifier (e.g., lower the onset voltage while increase the rectification ratio), the on/off ratio of a molecular switch or transistor, and the efficiency of thermoelectric energy conversion. Fourth, at some point, if not now, the promising molecular candidates need to be integrated into a scalable device fabrication. It would be best if extra attention is given to the stability and robustness of molecular junction devices in future research. In fact, extra credit should be given to the effort of developing revolutionary device architectures that easily accommodate molecular components in an integrated system. Fifth and last, the fundamental single-molecule studies of non-covalent interactions may pave the path toward understanding some of the most sophisticated yet fascinating biological processes, such as photosynthesis and radical-pairing magnetoreception. More single-molecule experimental and theoretical investigations along this line should be devoted as well.
While we focus on addressing the above questions, one can also expect that both exciting opportunities and new challenges will unfold as single-molecule electronics actively interface with the communities of supramolecular chemistry, molecular machines, and biomaterials. We believe the intimate communication and collaboration among researchers in relevant areas will continue to make remarkable progress, as we have seen in recent years, which will subsequently unveil roadmaps for future application and expansion in every aspect of the field.
Author contributions
Conceptualization, K. W.; writing—original draft preparation, R. T. A. and M. S.; schematic, B. S. and M. S.; writing—review and editing, K. W., B. S., M. G.; supervision, K. W. All authors have read and agreed to the published version of the manuscript.
Conflicts of interest
The authors declare no conflict of interest.
Acknowledgements
We gratefully thank the support from the U.S. Department of Energy, Office of Basic Energy Sciences under Award No. DE-SC0021942.
References
- F. Evers, R. Korytár, S. Tewari and J. M. van Ruitenbeek, Advances and challenges in single-molecule electron transport, Rev. Mod. Phys., 2020, 92, 035001 CrossRef CAS
.
- C. Tang, R. T. Ayinla and K. Wang, Beyond electrical conductance: progress and prospects in single-molecule junctions, J. Mater. Chem. C, 2022, 10, 13717–13733 RSC
.
- S. V. Aradhya and L. Venkataraman, Single-molecule junctions beyond electronic transport, Nat. Nanotechnol., 2013, 8, 399–410 CrossRef CAS PubMed
.
- P. Gehring, J. M. Thijssen and H. S. van der Zant, Single-molecule quantum-transport phenomena in break junctions, Nat. Rev. Phys., 2019, 1, 381–396 CrossRef
.
- Y. Komoto, S. Fujii, M. Iwane and M. Kiguchi, Single-molecule junctions for molecular electronics, J. Mater. Chem. C, 2016, 4, 8842–8858 RSC
.
- C. A. Mirkin and M. A. Ratner, Molecular electronics, Annu. Rev. Phys. Chem., 1992, 43, 719–754 CrossRef CAS
.
- M. Ratner, A brief history of molecular electronics, Nat. Nanotechnol., 2013, 8, 378–381 CrossRef CAS PubMed
.
- S. A. Mavrommati and S. S. Skourtis, Molecular Wires for Efficient Long-Distance Triplet Energy Transfer, J. Phys. Chem. Lett., 2022, 13, 9679–9687 CrossRef CAS PubMed
.
- D. Pavc, N. Sebastian, L. Spindler, I. Drevenšek-Olenik, G. K. Podboršek, J. Plavec and P. Šket, Understanding self-assembly at molecular level enables controlled design of DNA G-wires of different properties, Nat. Commun., 2022, 13, 1–11 Search PubMed
.
- Y. Tanaka, K. Ohmura, S. Fujii, T. Tada, M. Kiguchi and M. Akita, Single-Molecule Junction of a Cationic Rh(III) Polyyne Molecular Wire, Inorg. Chem., 2020, 59, 13254–13261 CrossRef CAS PubMed
.
- C. Yang, Q. Zhu, M. Sadakane, Z. Zhang, Y. Li and W. Ueda, Vanadium-Enhanced Intramolecular Redox Property of a Transition-Metal Oxide Molecular Wire, Inorg. Chem., 2020, 59, 16557–16566 CrossRef CAS PubMed
.
- I. Díez-Pérez, J. Hihath, Y. Lee, L. Yu, L. Adamska, M. A. Kozhushner, I. I. Oleynik and N. Tao, Rectification and stability of a single molecular diode with controlled orientation, Nat. Chem., 2009, 1, 635–641 CrossRef PubMed
.
- C. Guo, K. Wang, E. Zerah-Harush, J. Hamill, B. Wang, Y. Dubi and B. Xu, Molecular rectifier composed of DNA with high rectification ratio enabled by intercalation, Nat. Chem., 2016, 8, 484–490 CrossRef CAS PubMed
.
- T. Li, V. K. Bandari, M. Hantusch, J. Xin, R. Kuhrt, R. Ravishankar, L. Xu, J. Zhang, M. Knupfer and F. Zhu, Integrated molecular diode as 10 MHz half-wave rectifier based on an organic nanostructure heterojunction, Nat. Commun., 2020, 11, 1–10 CrossRef PubMed
.
- M. L. Perrin, E. Galán, R. Eelkema, J. M. Thijssen, F. Grozema and H. S. van der Zant, A gate-tunable single-molecule diode, Nanoscale, 2016, 8, 8919–8923 RSC
.
- J. Li, S. Pudar, H. Yu, S. Li, J. S. Moore, J. Rodríguez-López, N. E. Jackson and C. M. Schroeder, Reversible Switching of Molecular Conductance in Viologens is Controlled by the Electrochemical Environment, J. Phys. Chem. C, 2021, 125, 21862–21872 CrossRef CAS
.
- S. Y. Quek, M. Kamenetska, M. L. Steigerwald, H. J. Choi, S. G. Louie, M. S. Hybertsen, J. Neaton and L. Venkataraman, Mechanically controlled binary conductance switching of a single-molecule junction, Nat. Nanotechnol., 2009, 4, 230–234 CrossRef CAS PubMed
.
- T. A. Su, H. Li, M. L. Steigerwald, L. Venkataraman and C. Nuckolls, Stereoelectronic switching in single-molecule junctions, Nat. Chem., 2015, 7, 215–220 CrossRef CAS PubMed
.
- X. Yin, Y. Zang, L. Zhu, J. Z. Low, Z.-F. Liu, J. Cui, J. B. Neaton, L. Venkataraman and L. M. Campos, A reversible single-molecule switch based on activated antiaromaticity, Sci. Adv., 2017, 3, eaao2615 CrossRef PubMed
.
- H. Fu, X. Zhu, P. Li, M. Li, L. Yang, C. Jia and X. Guo, Recent progress in single-molecule transistors: their designs, mechanisms and applications, J. Mater. Chem. C, 2022, 10, 2375–2389 RSC
.
- J. Martínez-Blanco, C. Nacci, S. C. Erwin, K. Kanisawa, E. Locane, M. Thomas, F. Von Oppen, P. W. Brouwer and S. Fölsch, Gating a single-molecule transistor with individual atoms, Nat. Phys., 2015, 11, 640–644 Search PubMed
.
- J. Park, A. N. Pasupathy, J. I. Goldsmith, C. Chang, Y. Yaish, J. R. Petta, M. Rinkoski, J. P. Sethna, H. D. Abruña and P. L. McEuen, Coulomb blockade and the Kondo effect in single-atom transistors, Nature, 2002, 417, 722–725 CrossRef CAS PubMed
.
- H. Song, Y. Kim, Y. H. Jang, H. Jeong, M. A. Reed and T. Lee, Observation of molecular orbital gating, Nature, 2009, 462, 1039–1043 CrossRef CAS PubMed
.
- S. Goswami, R. Pramanick, A. Patra, S. P. Rath, M. Foltin, A. Ariando, D. Thompson, T. Venkatesan, S. Goswami and R. S. Williams, Decision trees within a molecular memristor, Nature, 2021, 597, 51–56 CrossRef CAS PubMed
.
- M. Mannini, F. Pineider, P. Sainctavit, C. Danieli, E. Otero, C. Sciancalepore, A. M. Talarico, M.-A. Arrio, A. Cornia and D. Gatteschi, Magnetic memory of a single-molecule quantum magnet wired to a gold surface, Nat. Mater., 2009, 8, 194–197 CrossRef CAS PubMed
.
- J. Y. Mao, L. Zhou, X. Zhu, Y. Zhou and S. T. Han, Photonic memristor for future computing: a perspective, Adv. Opt. Mater., 2019, 7, 1900766 CrossRef CAS
.
- B. Xu and N. J. Tao, Measurement of single-molecule resistance by repeated formation of molecular junctions, Science, 2003, 301, 1221–1223 CrossRef CAS PubMed
.
- W. Lee and P. Reddy, Creation of stable molecular junctions with a custom-designed scanning tunneling microscope, Nanotechnology, 2011, 22, 485703 CrossRef PubMed
.
- A. K. Ismael, K. Wang, A. Vezzoli, M. K. Al-Khaykanee, H. E. Gallagher, I. M. Grace, C. J. Lambert, B. Xu, R. J. Nichols and S. J. Higgins, Side-Group-Mediated Mechanical Conductance Switching in Molecular Junctions, Angew. Chem., Int. Ed., 2017, 56, 15378–15382 CrossRef CAS PubMed
.
- M. Kamenetska, M. Koentopp, A. C. Whalley, Y. S. Park, M. L. Steigerwald, C. Nuckolls, M. S. Hybertsen and L. Venkataraman, Formation and evolution of single-molecule junctions, Phys. Rev. Lett., 2009, 102, 126803 CrossRef CAS PubMed
.
- Y. Zang, A. Pinkard, Z. F. Liu, J. B. Neaton, M. L. Steigerwald, X. Roy and L. Venkataraman, Electronically Transparent Au-N Bonds for Molecular Junctions, J. Am. Chem. Soc., 2017, 139, 14845–14848 CrossRef CAS PubMed
.
- Y. Zhao, W. Liu, J. Zhao, Y. Wang, J. Zheng, J. Liu, W. Hong and Z.-Q. Tian, The fabrication, characterization and functionalization in molecular electronics, Int. J. Extreme Manuf., 2022, 4, 022003 CrossRef
.
- S. Vrouwe, E. Van der Giessen, S. Van der Molen, D. Dulic, M. Trouwborst and B. Van, Wees, Mechanics of lithographically defined break junctions, Phys. Rev. B, 2005, 71, 035313 CrossRef
.
- A. Pirrotta, L. De Vico, G. C. Solomon and I. Franco, Single-molecule force-conductance spectroscopy of hydrogen-bonded complexes, J. Chem. Phys., 2017, 146, 092329 CrossRef
.
- L. Mejía and I. Franco, Force–conductance spectroscopy of a single-molecule reaction, Chem. Sci., 2019, 10, 3249–3256 RSC
.
- J. M. Hamill, K. Wang and B. Xu, Force and conductance molecular break junctions with time series crosscorrelation, Nanoscale, 2014, 6, 5657–5661 RSC
.
- K. Wang, J. M. Hamill, J. Zhou and B. Xu, Mapping the details of contact effect of modulated Au-octanedithiol-Au break junction by force-conductance cross-correlation, J. Am. Chem. Soc., 2014, 136, 17406–17409 CrossRef CAS PubMed
.
- L. Arzubiaga, F. Golmar, R. Llopis, F. Casanova and L. E. Hueso, Tailoring palladium nanocontacts by electromigration, Appl. Phys. Lett., 2013, 102, 193103 CrossRef
.
- Y. Noguchi, T. Nagase, T. Kubota, T. Kamikado and S. Mashiko, Fabrication of Au–molecule–Au junctions using electromigration method, Thin Solid Films, 2006, 499, 90–94 CrossRef CAS
.
- A. Umeno and K. Hirakawa, Spectroscopic analysis of electromigration at gold nanojunctions, Phys. E, 2010, 42, 2826–2829 CrossRef CAS
.
- M. El Abbassi, S. Sangtarash, X. Liu, M. L. Perrin, O. Braun, C. Lambert, H. S. J. van der Zant, S. Yitzchaik, S. Decurtins and S.-X. Liu, Robust graphene-based molecular devices, Nat. Nanotechnol., 2019, 14, 957–961 CrossRef CAS PubMed
.
- S. Lumetti, A. Candini, C. Godfrin, F. Balestro, W. Wernsdorfer, S. Klyatskaya, M. Ruben and M. Affronte, Single-molecule devices with graphene electrodes, Dalton Trans., 2016, 45, 16570–16574 RSC
.
- Q. Wu, S. Hou, H. Sadeghi and C. J. Lambert, A single-molecule porphyrin-based switch for graphene nano-gaps, Nanoscale, 2018, 10, 6524–6530 RSC
.
- C. Yang, A. Qin, B. Z. Tang and X. Guo, Fabrication and functions of graphene–molecule–graphene single-molecule junctions, J. Chem. Phys., 2020, 152, 120902 CrossRef CAS PubMed
.
- N. Xin, J. Guan, C. Zhou, X. Chen, C. Gu, Y. Li, M. A. Ratner, A. Nitzan, J. F. Stoddart and X. Guo, Concepts in the design and engineering of single-molecule electronic devices, Nat. Rev. Phys., 2019, 1, 211–230 CrossRef
.
- X. Xie, P. Li, Y. Xu, L. Zhou, Y. Yan, L. Xie, C. Jia and X. Guo, Single-Molecule Junction: A Reliable Platform for Monitoring Molecular Physical and Chemical Processes, ACS Nano, 2022, 16, 3476–3505 CrossRef CAS PubMed
.
- L. Sun, Y. A. Diaz-Fernandez, T. A. Gschneidtner, F. Westerlund, S. Lara-Avila and K. Moth-Poulsen, Single-molecule electronics: from chemical design to functional devices, Chem. Soc. Rev., 2014, 43, 7378–7411 RSC
.
- H. Chen, C. Jia, X. Zhu, C. Yang, X. Guo and J. F. Stoddart, Reactions in single-molecule junctions, Nat. Rev. Mater., 2023, 8, 165–185 CrossRef
.
- T. A. Su, M. Neupane, M. L. Steigerwald, L. Venkataraman and C. Nuckolls, Chemical principles of single-molecule electronics, Nat. Rev. Mater., 2016, 1, 1–15 Search PubMed
.
- I. K. Mati and S. L. Cockroft, Molecular balances for quantifying non-covalent interactions, Chem. Soc. Rev., 2010, 39, 4195–4205 RSC
.
- K. Müller-Dethlefs and P. Hobza, Noncovalent interactions: a challenge for experiment and theory, Chem. Rev., 2000, 100, 143–168 CrossRef PubMed
.
- D. Umadevi, S. Panigrahi and G. N. Sastry, Noncovalent interaction of carbon nanostructures, Acc. Chem. Res., 2014, 47, 2574–2581 CrossRef CAS PubMed
.
- A. K. Ismael and C. J. Lambert, Single-molecule conductance oscillations in alkane rings, J. Mater. Chem. C, 2019, 7, 6578–6581 RSC
.
- L. Venkataraman, J. E. Klare, I. W. Tam, C. Nuckolls, M. S. Hybertsen and M. L. Steigerwald, Single-molecule circuits with well-defined molecular conductance, Nano Lett., 2006, 6, 458–462 CrossRef CAS PubMed
.
- S. Y. Quek, H. J. Choi, S. G. Louie and J. B. Neaton, Length dependence of conductance in aromatic single-molecule junctions, Nano Lett., 2009, 9, 3949–3953 CrossRef CAS PubMed
.
- Y. Tanaka, M. Kiguchi and M. Akita, Inorganic and Organometallic Molecular Wires for Single-Molecule Devices, Chem. – Eur. J., 2017, 23, 4741–4749 CrossRef CAS PubMed
.
- K. Sugimoto, Y. Tanaka, S. Fujii, T. Tada, M. Kiguchi and M. Akita, Organometallic molecular wires as versatile modules for energy-level alignment of the metal–molecule–metal junction, Chem. Commun., 2016, 52, 5796–5799 RSC
.
- M. Akita and Y. Tanaka, Carbon-rich organometallics: application to molecular electronics, Coord. Chem. Rev., 2022, 461, 214501 CrossRef CAS
.
- H. Chen and J. Fraser, Stoddart, From molecular to supramolecular electronics, Nat. Rev. Mater., 2021, 6, 804–828 CrossRef CAS
.
- G. Gryn’ova and C. Corminboeuf, Noncovalent molecular electronics, J. Phys. Chem. Lett., 2018, 9, 2298–2304 CrossRef PubMed
.
- O. Adak, E. Rosenthal, J. Meisner, E. F. Andrade, A. N. Pasupathy, C. Nuckolls, M. S. Hybertsen and L. Venkataraman, Flicker noise as a probe of electronic interaction at metal–single molecule interfaces, Nano Lett., 2015, 15, 4143–4149 CrossRef CAS PubMed
.
- M. Taniguchi, Paving the way to single-molecule chemistry through molecular electronics, Phys. Chem. Chem. Phys., 2019, 21, 9641–9650 RSC
.
- T. Q. Ha, I. J. Planje, J. R. White, A. C. Aragonès and I. Díez-Pérez, Charge transport at the protein–electrode interface in the emerging field of BioMolecular Electronics, Curr. Opin. Electrochem., 2021, 28, 100734 CrossRef CAS
.
- S. Song, L. Wang, J. Su, Z. Xu, C.-H. Hsu, C. Hua, P. Lyu, J. Li, X. Peng and T. Kojima, Manifold dynamic non-covalent interactions for steering molecular assembly and cyclization, Chem. Sci., 2021, 12, 11659–11667 RSC
.
- L. Andrezálová and Z. Országhová, Covalent and noncovalent interactions of coordination compounds with DNA: an overview, J. Inorg. Biochem., 2021, 225, 111624 CrossRef PubMed
.
- X. Li, W. Ge, S. Guo, J. Bai and W. Hong, Characterization and Application of Supramolecular Junctions, Angew. Chem., Int. Ed., 2023, 62, e202216819 CAS
.
- A. Magyarkuti, O. Adak, A. Halbritter and L. Venkataraman, Electronic and mechanical characteristics of stacked dimer molecular junctions, Nanoscale, 2018, 10, 3362–3368 RSC
.
- X. Li, Q. Wu, J. Bai, S. Hou, W. Jiang, C. Tang, H. Song, X. Huang, J. Zheng and Y. Yang, Structure-Independent Conductance of Thiophene-Based Single-Stacking Junctions, Angew. Chem., Int. Ed., 2020, 59, 3280–3286 CrossRef CAS PubMed
.
- T. Chen, M. Li and J. Liu, π–π stacking interaction: a nondestructive and facile means in material engineering for bioapplications, Cryst. Growth Des., 2018, 18, 2765–2783 CrossRef CAS
.
- X. Li, D. Hu, Z. Tan, J. Bai, Z. Xiao, Y. Yang, J. Shi and W. Hong, Supramolecular Systems and Chemical Reactions in Single-Molecule Break Junctions, Top. Curr. Chem., 2017, 375, 1–19 CrossRef CAS PubMed
.
- A. Feng, Y. Zhou, M. A. Al-Shebami, L. Chen, Z. Pan, W. Xu, S. Zhao, B. Zeng, Z. Xiao and Y. Yang, σ–σ Stacked supramolecular junctions, Nat. Chem., 2022, 14, 1158–1164 CrossRef CAS PubMed
.
- S. Y. Yang, Y. K. Qu, L. S. Liao, Z. Q. Jiang and S. T. Lee, Research Progress of Intramolecular π-Stacked Small Molecules for Device Applications, Adv. Mater., 2022, 34, 2104125 CrossRef CAS PubMed
.
- S. T. Schneebeli, M. Kamenetska, Z. Cheng, R. Skouta, R. A. Friesner, L. Venkataraman and R. Breslow, Single-molecule conductance through multiple π–π-stacked benzene rings determined with direct electrode-to-benzene ring connections, J. Am. Chem. Soc., 2011, 133, 2136–2139 CrossRef CAS PubMed
.
- J. Li, P. Shen, S. Zhen, C. Tang, Y. Ye, D. Zhou, W. Hong, Z. Zhao and B. Z. Tang, Mechanical single-molecule potentiometers with large switching factors from ortho-pentaphenylene foldamers, Nat. Commun., 2021, 12, 1–11 CrossRef PubMed
.
- R. Frisenda, V. A. Janssen, F. C. Grozema, H. S. Van Der Zant and N. Renaud, Mechanically controlled quantum interference in individual π-stacked dimers, Nat. Chem., 2016, 8, 1099–1104 CrossRef CAS PubMed
.
- P. Li, S. Hou, B. Alharbi, Q. Wu, Y. Chen, L. Zhou, T. Gao, R. Li, L. Yang and X. Chang, Quantum interference-controlled conductance enhancement in stacked graphene-like dimers, J. Am. Chem. Soc., 2022, 144, 15689–15697 CrossRef CAS PubMed
.
- G. C. Solomon, C. Herrmann, J. Vura-Weis, M. R. Wasielewski and M. A. Ratner, The chameleonic nature of electron transport through π-stacked systems, J. Am. Chem. Soc., 2010, 132, 7887–7889 CrossRef CAS PubMed
.
- S. Wu, M. T. González, R. Huber, S. Grunder, M. Mayor, C. Schönenberger and M. Calame, Molecular junctions based on aromatic coupling, Nat. Nanotechnol., 2008, 3, 569–574 CrossRef CAS PubMed
.
- D. Stefani, K. J. Weiland, M. Skripnik, C. Hsu, M. L. Perrin, M. Mayor, F. Pauly and H. S. Van Der Zant, Large conductance variations in a mechanosensitive single-molecule junction, Nano Lett., 2018, 18, 5981–5988 CrossRef CAS PubMed
.
- I. Franco, C. B. George, G. C. Solomon, G. C. Schatz and M. A. Ratner, Mechanically activated molecular switch through single-molecule pulling, J. Am. Chem. Soc., 2011, 133, 2242–2249 CrossRef CAS PubMed
.
- C. Wu, D. Bates, S. Sangtarash, N. Ferri, A. Thomas, S. J. Higgins, C. M. Robertson, R. J. Nichols, H. Sadeghi and A. Vezzoli, Folding a single-molecule junction, Nano Lett., 2020, 20, 7980–7986 CrossRef CAS PubMed
.
- T. Nishino, N. Hayashi and P. T. Bui, Direct measurement of electron transfer through a hydrogen bond between single molecules, J. Am. Chem. Soc., 2013, 135, 4592–4595 CrossRef CAS PubMed
.
- Y. Chen, H.-C. Wang, Y. Tang, Y. Zhou, L. Huang, J. Cao, C. Tang, M. Zhang, J. Shi and J. Liu, Modulation of charge transport through single-molecule bilactam junctions by tuning hydrogen bonds, Chem. Commun., 2021, 57, 1935–1938 RSC
.
- D. C. Milan, O. A. Al-Owaedi, M.-C. Oerthel, S. Marqués-González, R. J. Brooke, M. R. Bryce, P. Cea, J. Ferrer, S. J. Higgins and C. J. Lambert, Solvent dependence of the single molecule conductance of oligoyne-based molecular wires, J. Phys. Chem. C, 2016, 120, 15666–15674 CrossRef CAS
.
- L. Ge, S. Hou, Y. Chen, Q. Wu, L. Long, X. Yang, Y. Ji, L. Lin, G. Xue and J. Liu, Hydrogen-bond-induced quantum interference in single-molecule junctions of regioisomers, Chem. Sci., 2022, 13, 9552–9559 RSC
.
- C. Zhou, X. Li, Z. Gong, C. Jia, Y. Lin, C. Gu, G. He, Y. Zhong, J. Yang and X. Guo, Direct observation of single-molecule hydrogen-bond dynamics with single-bond resolution, Nat. Commun., 2018, 9, 1–9 Search PubMed
.
- S. Chang, J. He, A. Kibel, M. Lee, O. Sankey, P. Zhang and S. Lindsay, Tunnelling readout of hydrogen-bonding-based recognition, Nat. Nanotechnol., 2009, 4, 297–301 CrossRef CAS PubMed
.
- M. F. Budyka, Molecular switches and logic gates for information processing, the bottom-up strategy: from silicon to carbon, from molecules to supermolecules, Russ. Chem. Rev., 2017, 86, 181 CrossRef CAS
.
- S. Cai, W. Deng, F. Huang, L. Chen, C. Tang, W. He, S. Long, R. Li, Z. Tan and J. Liu, Light-Driven Reversible Intermolecular Proton Transfer at Single-Molecule Junctions, Angew. Chem., Int. Ed., 2019, 58, 3829–3833 CrossRef CAS PubMed
.
- B. Daly, T. S. Moody, A. J. Huxley, C. Yao, B. Schazmann, A. Alves-Areias, J. F. Malone, H. N. Gunaratne, P. Nockemann and A. P. de Silva, Molecular memory with downstream logic processing exemplified by switchable and self-indicating guest capture and release, Nat. Commun., 2019, 10, 49 CrossRef PubMed
.
- J. C. Ellenbogen and J. C. Love, Architectures for molecular electronic computers. I. Logic structures and an adder designed from molecular electronic diodes, Proc. IEEE, 2000, 88, 386–426 CAS
.
- J. Li, S. Hou, Y.-R. Yao, C. Zhang, Q. Wu, H.-C. Wang, H. Zhang, X. Liu, C. Tang and M. Wei, Room-temperature logic-in-memory operations in single-metallofullerene devices, Nat. Mater., 2022, 21, 917–923 CrossRef CAS PubMed
.
- J. An, S. Kim, A. Shrinidhi, J. Kim, H. Banna, G. Sung, K. M. Park and K. Kim, Purification of protein therapeutics via high-affinity supramolecular host–guest interactions, Nat. Biomed. Eng., 2020, 4, 1044–1052 CrossRef CAS PubMed
.
- B. Leśniewska, A. W. Coleman and K. Suwińska, Host–Guest Complexation of the para-Sulfonato-calix[4]arene Receptor with Biomolecules, Cryst. Growth Des., 2022, 22, 5947–5957 CrossRef
.
- J. S. Mosquera, I. García, M. Henriksen-Lacey, M. Martínez-Calvo, M. N. Dhanjani, J. L. Mascareñas and L. M. Liz-Marzán, Reversible control of protein corona formation on gold nanoparticles using host–guest interactions, ACS Nano, 2020, 14, 5382–5391 CrossRef CAS PubMed
.
- L. Shao, Y. Pan, B. Hua, S. Xu, G. Yu, M. Wang, B. Liu and F. Huang, Constructing adaptive photosensitizers via supramolecular modification based on pillararene host–guest interactions, Angew. Chem., Int. Ed., 2020, 59, 11779–11783 CrossRef CAS PubMed
.
- Q. Ai, L. Jin, Z. Gong and F. Liang, Observing Host–Guest Interactions at Molecular Interfaces by Monitoring the Electrochemical Current, ACS Omega, 2020, 5, 10581–10585 CrossRef CAS PubMed
.
- Y.-W. Yang, Y.-L. Sun and N. Song, Switchable host–guest systems on surfaces, Acc. Chem. Res., 2014, 47, 1950–1960 CrossRef CAS PubMed
.
- J. Zhang and P. X. Ma, Host–guest interactions mediated nano-assemblies using cyclodextrin-containing hydrophilic polymers and their biomedical applications, Nano Today, 2010, 5, 337–350 CrossRef CAS PubMed
.
- J. M. Abendroth, O. S. Bushuyev, P. S. Weiss and C. J. Barrett, Controlling motion at the nanoscale: rise of the molecular machines, ACS Nano, 2015, 9, 7746–7768 CrossRef CAS PubMed
.
- W. S. Jeon, E. Kim, Y. H. Ko, I. Hwang, J. W. Lee, S. Y. Kim, H. J. Kim and K. Kim, Molecular loop lock: a redox-driven molecular machine based on a host-stabilized charge-transfer complex, Angew. Chem., Int. Ed., 2005, 117, 89–93 CrossRef
.
- S. Krause and B. L. Feringa, Towards artificial molecular factories from framework-embedded molecular machines, Nat. Rev. Chem., 2020, 4, 550–562 CrossRef CAS
.
- W. Ong and A. E. Kaifer, Salt effects on the apparent stability of the cucurbit[7]uril-methyl viologen inclusion complex, J. Org. Chem., 2004, 69, 1383–1385 CrossRef CAS PubMed
.
- J.-H. Tang, Y. Li, Q. Wu, Z. Wang, S. Hou, K. Tang, Y. Sun, H. Wang, H. Wang and C. Lu, Single-molecule level control of host–guest interactions in metallocycle-C60 complexes, Nat. Commun., 2019, 10, 1–9 CrossRef PubMed
.
- X. Xiao, Z. Tao, S.-F. Xue, Q.-J. Zhu, J.-X. Zhang, G. A. Lawrance, B. Raguse and G. Wei, Interaction between cucurbit[8]uril and viologen derivatives, J. Inclusion Phenom. Macrocyclic Chem., 2008, 61, 131–138 CrossRef CAS
.
- W. Zhang, S. Gan, A. Vezzoli, R. J. Davidson, D. C. Milan, K. V. Luzyanin, S. J. Higgins, R. J. Nichols, A. Beeby and P. J. Low, Single-molecule conductance of viologen–cucurbit[8]uril host–guest complexes, ACS Nano, 2016, 10, 5212–5220 CrossRef CAS PubMed
.
- W. S. Jeon, H.-J. Kim, C. Lee and K. Kim, Control of the stoichiometry in host–guest complexation by redox chemistry of guests: inclusion of methylviologen in cucurbit[8]uril, Chem. Commun., 2002, 1828–1829 RSC
.
- H.-J. Kim, W. S. Jeon, Y. H. Ko and K. Kim, Inclusion of methylviologen in cucurbit[7]uril, Proc. Natl. Acad. Sci. U. S. A., 2002, 99, 5007–5011 CrossRef CAS PubMed
.
- S. Li, J. Li, H. Yu, S. Pudar, B. Li, J. Rodríguez-López, J. S. Moore and C. M. Schroeder, Characterizing intermolecular interactions in redox-active pyridinium-based molecular junctions, J. Electroanal. Chem., 2020, 875, 114070 CrossRef CAS
.
- D. Su, S. Zhou, H. Masai, Z. Liu, C. Zhou, C. Yang, Z. Li, S. Tsuda, Z. Liu and J. Terao, Stochastic Binding Dynamics of a Photoswitchable Single Supramolecular Complex, Adv. Sci., 2022, 9, 2200022 CrossRef CAS PubMed
.
- Z. Liu, X. Li, H. Masai, X. Huang, S. Tsuda, J. Terao, J. Yang and X. Guo, A single-molecule electrical approach for amino acid detection and chirality recognition, Sci. Adv., 2021, 7, eabe4365 CrossRef CAS PubMed
.
- E. Saparbaev, R. Yamaletdinov and O. V. Boyarkin, Identification of Isomeric Lipids by UV Spectroscopy of Noncovalent Complexes with Aromatic Molecules, Anal. Chem., 2021, 93, 12822–12826 CrossRef CAS PubMed
.
- H.-J. Schneider, F. Hacket, V. Rudiger and H. Ikeda, NMR studies of cyclodextrins and cyclodextrin complexes, Chem. Rev., 1998, 98, 1755–1785 CrossRef CAS PubMed
.
- Y. Xu, P. M. Szell, V. Kumar and D. L. Bryce, Solid-state NMR spectroscopy for the analysis of element-based non-covalent interactions, Coord. Chem. Rev., 2020, 411, 213237 CrossRef CAS
.
- M. E. Bush, N. D. Bouley and A. R. Urbach, Charge-mediated recognition of N-terminal tryptophan in aqueous solution by a synthetic host, J. Am. Chem. Soc., 2005, 127, 14511–14517 CrossRef CAS PubMed
.
- K. Moon and A. E. Kaifer, Modes of binding interaction between viologen guests and the cucurbit[7]uril host, Org. Lett., 2004, 6, 185–188 CrossRef CAS PubMed
.
- G. R. Patzke, F. Krumeich and R. Nesper, Oxidic nanotubes and nanorods—anisotropic modules for a future nanotechnology, Angew. Chem., Int. Ed., 2002, 41, 2446–2461 CrossRef CAS PubMed
.
- M. O. Mitchell and J. Means, Cation− Π Interactions in Biochemistry: A Primer, J. Chem. Educ., 2018, 95, 2284–2288 CrossRef CAS
.
- C. R. Kennedy, S. Lin and E. N. Jacobsen, The Cation–π Interaction in Small-Molecule Catalysis, Angew. Chem., Int. Ed., 2016, 55, 12596–12624 CrossRef CAS PubMed
.
- T. Ogoshi, S. Kanai, S. Fujinami, T.-A. Yamagishi and Y. Nakamoto,
para-Bridged symmetrical pillar[5]arenes: their Lewis acid catalyzed synthesis and host–guest property, J. Am. Chem. Soc., 2008, 130, 5022–5023 CrossRef CAS PubMed
.
- X. Li, S. Zhou, Q. Zhao, Y. Chen, P. Qi, Y. Zhang, L. Wang, C. Guo and S. Chen, Supramolecular enhancement of charge transport through pillar[5]arene-based self-assembled monolayers, Angew. Chem., Int. Ed., 2023, e202216987 CAS
.
- J. C. Ma and D. A. Dougherty, The cation–π interaction, Chem. Rev., 1997, 97, 1303–1324 CrossRef CAS PubMed
.
- A. S. Mahadevi and G. N. Sastry, Cation–π interaction: its role and relevance in chemistry, biology, and material science, Chem. Rev., 2013, 113, 2100–2138 CrossRef CAS PubMed
.
- R. Wang, Y. Li, A. Tang, Y. Li and H. Li, Gating the conductance of a single-molecule junction with ion-π interaction, Chem. Commun., 2022, 58, 8290–8293 RSC
.
- B. Xiao, S. He, M. Sun, J. Zhou, Z. Wang, Y. Li, S. Liu, W. M. Nau and S. Chang, Dynamic interconversions of single molecules probed by recognition tunneling at cucurbit[7]uril-functionalized supramolecular junctions, Angew. Chem., Int. Ed., 2022, e202203830 CAS
.
- M. Kiguchi, S. Nakashima, T. Tada, S. Watanabe, S. Tsuda, Y. Tsuji and J. Terao, Single-molecule conductance of π-conjugated rotaxane: new method for measuring stipulated electric conductance of π-conjugated molecular wire using STM break junction, Small, 2012, 8, 726–730 CrossRef CAS PubMed
.
- S. Y. Jon, Y. H. Ko, S. H. Park, H.-J. Kim and K. Kim, A facile, stereoselective [2+2] photoreaction mediated by cucurbit[8]uril, Chem. Commun., 2001, 1938–1939 RSC
.
- D. V. W. Kankanamalage, J. H. Tran, N. Beltrami, K. Meng, X. Zhou, P. Pathak, L. Isaacs, A. L. Burin, M. F. Ali and J. Jayawickramarajah, DNA Strand Displacement Driven by Host–Guest Interactions, J. Am. Chem. Soc., 2022, 144, 16502–16511 CrossRef CAS PubMed
.
- D. Tuncel and J. H. Steinke, Catalytic self-threading: a new route for the synthesis of polyrotaxanes, Macromolecules, 2004, 37, 288–302 CrossRef CAS
.
- J. Zhou, Y. Wu, I. Roy, A. Samanta, J. F. Stoddart, R. M. Young and M. R. Wasielewski, Choosing sides: unusual ultrafast charge transfer pathways in an asymmetric electron-accepting cyclophane that binds an electron donor, Chem. Sci., 2019, 10, 4282–4292 RSC
.
- S. Yuan, Q. Qian, Y. Zhou, S. Zhao, L. Lin, P. Duan, X. Xu, J. Shi, W. Xu and A. Feng, Tracking confined reaction based on host–guest interaction using single-molecule conductance measurement, Small, 2022, 18, 2104554 CrossRef CAS PubMed
.
- C. Gu, C. Jia and X. Guo, Single-molecule electrical detection with real-time label-free capability and ultrasensitivity, Small Methods, 2017, 1, 1700071 CrossRef
.
- C. Gu, C. Hu, Y. Wei, D. Lin, C. Jia, M. Li, D. Su, J. Guan, A. Xia and L. Xie, Label-free dynamic detection of single-molecule nucleophilic-substitution reactions, Nano Lett., 2018, 18, 4156–4162 CrossRef CAS PubMed
.
- C. Jia, A. Migliore, N. Xin, S. Huang, J. Wang, Q. Yang, S. Wang, H. Chen, D. Wang and B. Feng, Covalently bonded single-molecule junctions with stable and reversible photoswitched conductivity, Science, 2016, 352, 1443–1445 CrossRef CAS PubMed
.
- G. Ke, C. Duan, F. Huang and X. Guo, Electrical and spin switches in single-molecule junctions, InfoMat, 2020, 2, 92–112 CrossRef CAS
.
- H. Wen, W. Li, J. Chen, G. He, L. Li, M. A. Olson, A. C.-H. Sue, J. F. Stoddart and X. Guo, Complex formation dynamics in a single-molecule electronic device, Sci. Adv., 2016, 2, e1601113 CrossRef PubMed
.
- Y. Amharar, A. Grandeury, M. Sanselme, S. Petit and G. R. Coquerel, A hybrid mechanism in chiral discrimination induced by crystallization of supramolecular compounds, J. Phys. Chem. B, 2012, 116, 6027–6040 CrossRef CAS PubMed
.
- A. Douhal, Ultrafast guest dynamics in cyclodextrin nanocavities, Chem. Rev., 2004, 104, 1955–1976 CrossRef CAS PubMed
.
- K. Harata, Structural aspects of stereodifferentiation in the solid state, Chem. Rev., 1998, 98, 1803–1828 CrossRef CAS PubMed
.
- M. Rekharsky and Y. Inoue, Chiral recognition thermodynamics of β-cyclodextrin: the thermodynamic origin of enantioselectivity and the enthalpy− entropy compensation effect, J. Am. Chem. Soc., 2000, 122, 4418–4435 CrossRef CAS
.
- M. V. Rekharsky and Y. Inoue, Complexation thermodynamics of cyclodextrins, Chem. Rev., 1998, 98, 1875–1918 CrossRef CAS PubMed
.
- M. A. Nanny and J. P. Maza, Noncovalent interactions between monoaromatic compounds and dissolved humic acids: a deuterium NMR T1 relaxation study, Environ. Sci. Technol., 2001, 35, 379–384 CrossRef CAS PubMed
.
- Y.-H. Zhang, Y.-M. Zhang, X. Sheng, J. Wang and Y. Liu, Enzyme-responsive fluorescent camptothecin prodrug/polysaccharide supramolecular assembly for targeted cellular imaging and in situ controlled drug release, Chem. Commun., 2020, 56, 1042–1045 RSC
.
- M. R. Bryce, Recent progress on conducting organic charge-transfer salts, Chem. Soc. Rev., 1991, 20, 355–390 RSC
.
- D. N. Dhar, The Chemistry of tetracyanoethylene, Chem. Rev., 1967, 67, 611–622 CrossRef CAS
.
- L. Melby, R. Harder, W. Hertler, W. Mahler, R. Benson and W. Mochel, Substituted quinodimethans. II. Anion-radical derivatives and complexes of 7,7,8,8-tetracyanoquinodimethan, J. Am. Chem. Soc., 1962, 84, 3374–3387 CrossRef CAS
.
- H. Akamatu, H. Inokuchi and Y. Matsunaga, Electrical conductivity of the perylene–bromine complex, Nature, 1954, 173, 168–169 CrossRef
.
- J. Ferraris, D. Cowan, V. T. Walatka and J. Perlstein, Electron transfer in a new highly conducting donor–acceptor complex, J. Am. Chem. Soc., 1973, 95, 948–949 CrossRef CAS
.
- D. Jérome, A. Mazaud, M. Ribault and K. Bechgaard, Superconductivity in a synthetic organic conductor (TMTSF) 2PF 6, J. Phys., Lett., 1980, 41, 95–98 CrossRef
.
- R. García, M. Á. Herranz, E. Leary, M. T. González, G. R. Bollinger, M. Bürkle, L. A. Zotti, Y. Asai, F. Pauly and J. C. Cuevas, Single-molecule conductance of a chemically modified, π-extended tetrathiafulvalene and its charge-transfer complex with F4TCNQ, Beilstein J. Org. Chem., 2015, 11, 1068–1078 CrossRef PubMed
.
- A. Vezzoli, I. Grace, C. Brooke, K. Wang, C. J. Lambert, B. Xu, R. J. Nichols and S. J. Higgins, Gating of single molecule junction conductance by charge transfer complex formation, Nanoscale, 2015, 7, 18949–18955 RSC
.
- A. Vezzoli, I. M. Grace, C. Brooke, R. J. Nichols, C. J. Lambert and S. J. Higgins, Soft versus hard junction formation for α-terthiophene molecular wires and their charge transfer complexes, J. Chem. Phys., 2017, 146, 092307 CrossRef
.
- K. Wang, A. Vezzoli, I. M. Grace, M. McLaughlin, R. J. Nichols, B. Xu, C. J. Lambert and S. J. Higgins, Charge transfer complexation boosts molecular conductance through Fermi level pinning, Chem. Sci., 2019, 10, 2396–2403 RSC
.
- I. Aprahamian, The future of molecular machines, ACS Cent. Sci., 2020, 6, 347–358 CrossRef CAS PubMed
.
- S. Erbas-Cakmak, D. A. Leigh, C. T. McTernan and A. L. Nussbaumer, Artificial molecular machines, Chem. Rev., 2015, 115, 10081–10206 CrossRef CAS PubMed
.
- E. R. Kay and D. A. Leigh, Rise of the molecular machines, Angew. Chem., Int. Ed., 2015, 54, 10080–10088 CrossRef CAS PubMed
.
- J. F. Stoddart, Mechanically interlocked molecules (MIMs)—molecular shuttles, switches, and machines (Nobel Lecture), Angew. Chem., Int. Ed., 2017, 56, 11094–11125 CrossRef CAS PubMed
.
- C. Yang and H. Chen, A roadmap for mechanically interlocked molecular junctions at nanoscale, ACS Appl. Nano Mater., 2022, 5, 13874–13886 CrossRef CAS
.
- C. Pezzato, C. Cheng, J. Stoddart and R. Astumian, Non-equilibrium supramolecular polymerization, Chem. Soc. Rev., 2017, 46, 5467–5680 RSC
.
- M. Baroncini, S. Silvi and A. Credi, Photo-and redox-driven artificial molecular motors, Chem. Rev., 2019, 120, 200–268 CrossRef PubMed
.
- D.-H. Qu, Q.-C. Wang, Q.-W. Zhang, X. Ma and H. Tian, Photoresponsive host–guest functional systems, Chem. Rev., 2015, 115, 7543–7588 CrossRef CAS PubMed
.
- S. Saha and J. F. Stoddart, Photo-driven molecular devices, Chem. Soc. Rev., 2007, 36, 77–92 RSC
.
- H.-Y. Zhou, Y. Han and C.-F. Chen, pH-Controlled motions in mechanically interlocked molecules, Mater. Chem. Front., 2020, 4, 12–28 RSC
.
- G. D. Scott, K. S. Chichak, A. J. Peters, S. J. Cantrill, J. F. Stoddart and H. Jiang, Mechanism of enhanced rectification in unimolecular Borromean ring devices, Phys. Rev. B, 2006, 74, 113404 CrossRef
.
- H. Yu, Y. Luo, K. Beverly, J. F. Stoddart, H. R. Tseng and J. R. Heath, The molecule–electrode interface in single-molecule transistors, Angew. Chem., Int. Ed., 2003, 42, 5706–5711 CrossRef CAS PubMed
.
- V. Balzani, A. Credi and M. Venturi, Molecular logic circuits, ChemPhysChem, 2003, 4, 49–59 CrossRef CAS PubMed
.
- C. N. R. Rao, B. Satishkumar, A. Govindaraj and M. Nath, Nanotubes, ChemPhysChem, 2001, 2, 78–105 CrossRef CAS PubMed
.
- J. E. Green, J. Wook Choi, A. Boukai, Y. Bunimovich, E. Johnston-Halperin, E. DeIonno, Y. Luo, B. A. Sheriff, K. Xu and Y. Shik Shin, A 160-kilobit molecular electronic memory patterned at 1011 bits per square centimetre, Nature, 2007, 445, 414–417 CrossRef CAS PubMed
.
- S. Mena-Hernando and E. M. Pérez, Mechanically interlocked materials. Rotaxanes and catenanes beyond the small molecule, Chem. Soc. Rev., 2019, 48, 5016–5032 RSC
.
- J. Sun, Y. Wu, Y. Wang, Z. Liu, C. Cheng, K. J. Hartlieb, M. R. Wasielewski and J. F. Stoddart, An electrochromic tristable molecular switch, J. Am. Chem. Soc., 2015, 137, 13484–13487 CrossRef CAS PubMed
.
- D. C. Milan, M. Krempe, A. K. Ismael, L. D. Movsisyan, M. Franz, I. Grace, R. J. Brooke, W. Schwarzacher, S. J. Higgins and H. L. Anderson, The single-molecule electrical conductance of a rotaxane-hexayne supramolecular assembly, Nanoscale, 2017, 9, 355–361 RSC
.
- C. Zhou, X. Li, H. Masai, Z. Liu, Y. Lin, T. Tamaki, J. Terao, J. Yang and X. Guo, Revealing charge-and temperature-dependent movement dynamics and mechanism of individual molecular machines, Small Methods, 2019, 3, 1900464 CrossRef CAS
.
- S. Chen, D. Su, C. Jia, Y. Li, X. Li, X. Guo, D. A. Leigh and L. Zhang, Real-time observation of the dynamics of an individual rotaxane molecular shuttle using a single-molecule junction, Chem, 2022, 8, 243–252 CAS
.
- D. Sluysmans, F. Devaux, C. J. Bruns, J. F. Stoddart and A.-S. Duwez, Dynamic force spectroscopy of synthetic oligorotaxane foldamers, Proc. Natl. Acad. Sci. U. S. A., 2018, 115, 9362–9366 CrossRef CAS PubMed
.
- Y. Wang, M. Frasconi, W.-G. Liu, J. Sun, Y. Wu, M. S. Nassar, Y. Y. Botros, W. A. Goddard III, M. R. Wasielewski and J. F. Stoddart, Oligorotaxane radicals under orders, ACS Cent. Sci., 2016, 2, 89–98 CrossRef CAS PubMed
.
- D. Sluysmans, S. Hubert, C. J. Bruns, Z. Zhu, J. F. Stoddart and A. S. Duwez, Synthetic oligorotaxanes exert high forces when folding under mechanical load, Nat. Nanotechnol., 2018, 13, 209–213 CrossRef CAS PubMed
.
Footnote |
† These authors contributed equally to this work. |
|
This journal is © the Partner Organisations 2023 |