DOI:
10.1039/D2QM01271E
(Review Article)
Mater. Chem. Front., 2023,
7, 1737-1759
Tailoring carbon-based nanofiber microstructures for electromagnetic absorption, shielding, and devices
Received
8th December 2022
, Accepted 11th February 2023
First published on 21st February 2023
Abstract
Carbon-based nanofibers have been a hot spot in various fields due to their distinctive nanostructures, functions, and performances. Especially in the field of electromagnetic (EM) functional materials and devices, carbon-based nanofibers have been sought after. In this review, we systematically elaborate the preparation methods and relevant synthesis mechanisms of carbon-based fiber materials, discuss the influence mechanism of nanofiber microstructure on the EM response, and dissect the tailoring strategies of nanofibers with different configurations to manipulate the EM attenuation performance. More importantly, multi-function EM devices constructed using carbon-based nanofibers are highlighted. Finally, the current opportunities of carbon-based nanofibers for EM materials and devices are analyzed, and the future challenges are predicted.
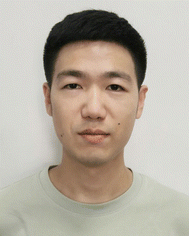
Qi Zheng
| Qi Zheng received his BS degree from Chang’an University in 2017 and MS degree from Shandong University in 2021. He is currently a PhD student at the Beijing Institute of Technology. His current research interest includes advanced electromagnetic functional materials and devices. |
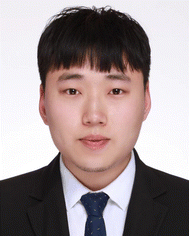
Wen-Qiang Cao
| Wen-Qiang Cao received his BS degree from Minzu University of China in 2011 and MS degree from Minzu University of China in 2017. He is currently a PhD student at the Beijing Institute of Technology. His current research interest includes advanced electromagnetic functional materialsand energy storage materials and devices. |
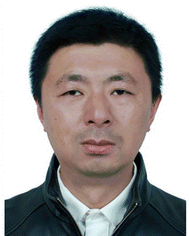
Huazhang Zhai
| Hua-Zhang Zhai received his PhD degree from Tsinghua University in 2003. He is currently an associate professor of Beijing Institute of Technology. His current research interest includes low-dimensional energy materials and environmental adsorption materials. |
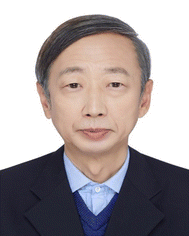
Mao-Sheng Cao
| Mao-Sheng Cao received his MS and PhD degrees from Harbin Institute of Technology in 1989 and 1998, respectively. He is currently a distinguished professor of Beijing Institute of Technology. His current research interest includes low-dimensional electromagnetic functional materials and devices. |
1. Introduction
The development and innovation of electronic communication technology have brought radical changes to human life. At the same time, unwanted electromagnetic (EM) pollution has caused harm to human beings and the environment. EM functional materials, including microwave absorption (MA) materials and electromagnetic interference (EMI) shielding materials, emerging as the times require, can attenuate EM waves and prevent EM pollution.1–4 Multifarious works have been made to prepare efficient EM functional materials. However, with the popularization and prosperity of intelligent information technology and the Internet of Things, the requirements of EM functional materials in practical applications have become higher.
Carbon materials, including carbon nanotubes (CNTs), carbon nanofibers (CNFs), graphene, graphite, and carbon microspheres have proved their application potential in EM functional materials due to their favorable dielectric loss. Among them, carbon-based nanofibers have been favored by researchers because they integrate the advantages of the carbon family such as light weight, high conductivity, excellent, stability, restorability, and corrosion resistance; on the other hand, they also have more active sites than carbon nanotubes and more diverse structural designs than graphene, larger specific surface area and more flexible than graphite. Moreover, carbon-based nanofibers can be used as templates or carriers to realize the design of delicate nanostructures. Carbon-based nanofibers have shown great potential in energy storage, catalysis, sensing, biomedicine, MA, EMI shielding, etc.5Fig. 1(b) shows that the publication number of articles on carbon-based nanofibers remains high and stable in various fields. More importantly, carbon-based nanofibers have received more attention in the field of EM functional materials (the insert in Fig. 1(b)). With the structural advantages of carbon-based nanofibers, their microstructures can be tailored to prepare EM functional materials with superior absorption or shielding performance. In particular, Green EMI shielding can be achieved by designing 2D films or 3D porous architectures, which was first proposed by Cao's group.6 Green EMI shielding means absorption is dominant in EMI shielding, and the second EM pollution can be inhibited. Many peers followed this theory and reported green shielding materials.7–9 Furthermore, EM devices can be designed using the EM response of EM materials to achieve multifunctional applications (Fig. 1(a)).10–21 According to different dimensions of the nanofiber structure, carbon-based nanofibers can be divided into one-dimensional (1D) nanofibers, two-dimensional (2D) films, and assembled three-dimensional (3D) architectures such as foams and aerogels. The works on 1D nanofibers are the most in the current research, while the works on 2D and 3D structures are also very important and cannot be ignored (Fig. 1(c)).
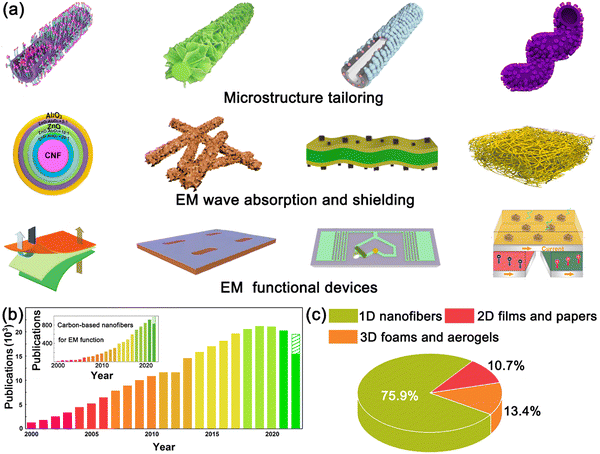 |
| Fig. 1 (a) Schematic illustration for microstructure tailoring of carbon-based nanofibers, carbon-based nanofibers for EM wave and shielding, and carbon-based nanofibers for EM functional devices. Reproduced from ref. 10–21 with permission. Copyright 2019, Wiley-VCH; Copyright 2018, Wiley-VCH; Copyright 2021, ACS; Copyright 2021, ACS; Copyright 2018, Springer; Copyright 2022, Elsevier; Copyright 2022, Elsevier; Copyright 2020, Elsevier; Copyright 2017, RSC; Copyright 2022, RSC; Copyright 2022, Elsevier; Copyright 2021, Elsevier. (b) Publication numbers of carbon-based nanofibers; The insert is the publication numbers of carbon-based nanofibers for EM functional materials. (Source: Web of Science) (c) publication proportion of carbon-based nanofibers with different dimensions for EM functional materials in 2021 (Source: Web of Science). | |
Structural tailoring of carbon-based nanofibers is the key to regulating their EM response. Structural design strategies of carbon-based nanofibers including surface modification, the introduction of internal structure, porous structure, and hollow structure, as well as compositing with other advanced low dimensional materials have been proven to improve EM performance. However, many studies have not clearly explained the influence mechanism of material structure on properties. Cao's Group proposed the theory of dielectric “genes” and magnetic “genes” for the first time to reveal the relationship between material microstructure and EM properties.22–24 Dielectric “genes” include conduction “genes” and polarization “genes”, wherein the former are electron migrating and hopping, and the latter are dipoles caused by defects, polar groups, and interfaces. Magnetic “genes” are the valence electrons located in the 3d orbital and can generate magnetic resonance and eddy current by different spin motions of valence electrons. Based on the theory of dielectric “genes” and magnetic “genes”, the material structure can be tailored purposefully to achieve the expected EM performance. This theory has been recognized and promoted by peers. Liu et al. synthesized carbon foam with an interpenetrating carbon network by planting polarization loss “gene”, which showed superior reflection loss (RL) of −72.8 dB and broad effective absorption bandwidth (EAB) of 6.2 GHz.25 Wu's group designed metal–organic frameworks (MOFs) to achieve an RL of −62.5 dB and EAB of 6.6 GHz by selective coding dielectric “genes”.26 The theory of dielectric “genes” and magnetic “genes” will be a powerful tool to prepare carbon-based EM functional nanofibers.
In this paper, the fabrication methods of carbon-based nanofibers are summarized, the corresponding synthesis mechanism is dissected in detail, and the influence of microstructure on EM attenuation and energy dissipation is discussed. Then, we highlight the tailoring strategies of carbon-based nanofibers with different structures, including 1D nanofibers, 2D films, and 3D foams for EM functional materials and devices. Finally, the existing challenges and future development opportunities of carbon-based nanofibers are deeply evaluated.
2. Fabrication of carbon-based nanofibers
Up to now, CNFs have been prepared by various methods such as electrospinning, chemical vapor deposition (CVD), polymerization, template method, and wet spinning. The diversiform fabrication methods endow CNFs with different morphologies, structures, and characteristics, and also enrich their applications. In this section, the fabrication methods and related synthesis mechanisms are summarized and analyzed.
2.1 Electrospinning
In recent decades, electrospinning technology has become more and more widely used in the preparation of nanofibers. It has been favored by researchers because of its simple equipment, controllable operation process, and low cost. In addition, using electrospinning technology a variety of nanofibers, including organic nanofibers, inorganic nanofibers and composite nanofibers can be prepared. As early as 1600, William Gilbert put forward the concept of electrospinning.27 He found that under the action of an electric field, the water droplet would become a cone. In 1934, Formhals first reported a series of patents related to electrospinning technology.28 After this, Taylor studied the influence of electric field force and surface tension on droplets in the electrospinning process and revealed the change of droplet model under an electric field.29 In particular, when the electric field strength increases to a certain critical value, the droplet will change from a sphere to a cone (Taylor cone) and eject a charged jet. Thanks to the emergence of nanoscale characterization technologies and equipment, electrospinning has made great progress since the 1900s. Electrospinning nanofibers have attracted the attention of researchers and have been applicated in catalysis, sensing, energy storage, photoelectric, filtering, medical engineering, etc. More importantly, through the regulation of process parameters and structural tailoring, multifarious nanofibers with different morphology, structure, composition, size, arrangement, distribution, and porosity have been synthesized, which further flourished their application in various fields.
The electrospinning technology device is composed of four parts: a syringe pump, syringe needle, high-voltage power supply, and collector (Fig. 2(a)).30 The syringe pump can store the solution and give the syringe forward propulsion. The syringe needle is used to spray the solution. The high-voltage power supply can build a strong electric field. The collector is used to receive the ejected nanofibers. During the electrospinning process, the pendant droplets are extruded from the syringe needle and tend to form spheres because of surface tension. Then, under the influence of an electric field, the surface charges of droplets with the same sign can generate forward tractive force, which can overcome the surface tension and make the spherical droplets change into Taylor cones. Under the traction of the electric field force, the Taylor cone ejects a charged jet (Fig. 2(b)).31 The jet passes through straight-line propagation and an unstable spiral swing stage and then forms a fiber. The fiber is further elongated while the solvent is evaporated and a thinner nanofiber is obtained (Fig. 2(c)).31 Finally, the nanofiber deposits on a conductive collector, which is a plate or a roller. The nanofiber size is greatly affected by the process parameters of electrospinning such as voltage, solution viscosity, the relative molecular weight of polymers, surface tension, conductivity, the driving speed of syringe pump, evaporation, the distance between needle and receiver, and temperature. For example, Yu's group revealed that the large surface tension of the solution can lead to the instability of fiber size.32 Koski et al. found that as the relative molecular weight of PVA increases, the nanofibers change from beaded to cylindrical, and then to flat ribbons.33 Katti and coworkers reported that as the voltage increases from 8 kV to 9 kV, the diameter of the poly(lactide-co-glycolide) nanofibers decreases from 900 nm to 450 nm.34
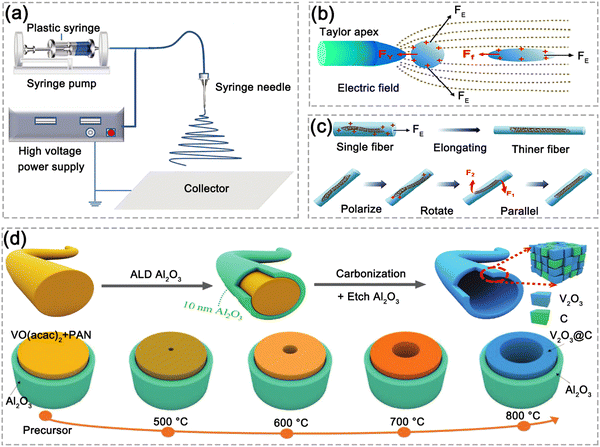 |
| Fig. 2 (a) Schematic diagram of electrospinning equipment. Reproduced from ref. 30 with permission. Copyright 2020, De Gruyter. (b) The electric field near the needle. (c) Evolution of the fiber in the process of moving forward. Reproduced from ref. 31 with permission. Copyright 2020, Wiley-VCH. (d) The decomposition process of carbon-based fibers during carbonization. Reproduced from ref. 36 with permission. Copyright 2020, Wiley-VCH. | |
CNFs derived from electrospinning precursor fibers are usually obtained after the carbonization process. Multiple structures of electrospinning precursor fibers such as beaded, banded, porous, hollow, and skin-core, can be retained after carbonization, which endows CNFs with structural diversity. The main components of electrospinning precursor fibers are usually polymers. During carbonization, non-carbon elements escape as gases and most of the C elements remain to form a carbon matrix. In addition, many other components, e.g., inorganic salts, carbon materials, perovskites, and metal particles can be mixed into polymer precursor solutions to synthesize composite nanofibers. For example, Guo et al. prepared hollow bead-like Fe3C/N-doped CNFs through electrospinning and thermal treatment.35 The Fe3C nanoparticles are generated from the reduction of ferric salt. Another advantage of electrospinning fiber is that the surface of the fiber is easy to be functionalized, and can be coated or modified by CVD, atomic layer deposition (ALD), electrodeposition, electroless plating, hydrothermal and other means, further improving the performance and application value of the nanofibers. Mao et al. reported oxide/carbon hollow composite nanofibers by electrospinning and the ALD method (Fig. 2(d)).36 The thin Al2O3 layer (∼10 nm) deposited on the outside of polyacrylonitrile (PAN) nanofibers by the ALD process plays a critical role in confining the pyrolysis of the precursor, which is essential to form hollow structures. During pyrolysis, the stable Al2O3 layer is used as an enclosed space, in which PAN is carbonized to carbon. At the same time, VO(acac)2 decomposes and diffuses to the outer Al2O3 layer. Continuous decomposition and diffusion result in a hollow structure inside the nanofibers. After Al2O3 is removed with an alkaline solution, hollow V2O3@C nanofibers are obtained (Fig. 2(d)).36 Further, they explored the nanofiber structure at different carbonation temperatures (500–800 °C). With the increase in temperature, the hollow holes in the nanofiber center will enlarge, which means the precursor expands gradually during decomposition under the constraint of the Al2O3 layer.
A wide range of applicable polymers, various structures, simple regulation means, and functionalized surfaces make electrospinning technology the most popular method for preparing carbon-based nanofibers. Various components and low-dimensional nanomaterials can be easily introduced into the matrix of CNFs obtained by electrospinning, providing a variety of mechanisms for the attenuation of EM waves. Diversified improved electrospinning processes, such as oriented electrospinning, coaxial electrospinning, and multi-needle electrospinning also provide new ideas for the structural design of EM functional carbon-based nanofibers. However, there are still some key problems hindering the development of electrospinning technology. Firstly, organic solvents are usually used to prepare precursor nanofibers, which are harmful to the human body and environment, so it is urgent to find environment-friendly solvents. Secondly, some studies found that inorganic nanofibers with diameters of tens of nanometers or below easily cause lung inflammation.27 Although the electrospinning nanofibers are relatively long and difficult to inhale into the lungs, the research on the inhalation safety of electrospinning nanofibers is very few, so their safety cannot be guaranteed. Finally, the market demand and output of electrospinning nanofibers do not match. The preparation of most electrospinning fibers is still on the laboratory scale, which is difficult to achieve industrial production. To reach mass production, the production process and equipment need to be improved and innovated.
2.2 CVD technology
CVD is a potential method for the continuous batch production of multiple kinds of deposits. A traditional CVD process can be divided into three steps: (I) diffusion of reaction gases. (II) Deposition of reaction gas on the substrate surface. (III) Chemical reactions occur on the surface of the substrate. During the chemical reaction, the gaseous substances can form solid substances on the surface. Gaseous substances are one or several compounds or simple substances. The gas phase by-products generated during the reaction are separated out, while the non-volatile solid products remain on the surface of the substrate. CVD technology has many advantages; for example, the reaction can be carried out under normal pressure or low vacuum conditions, and a variety of metal or non-metallic substances can be prepared; the product has good compactness and high crystallinity; the deposition layer with strong adhesiveness and good diffraction can deposit complex structures; the equipment is simple and operation is convenient; the structure of the final product can be manipulated by adjusting substrate, gases, flow rate, catalysts, temperature and pressure in the CVD process. According to different experimental conditions, CVD can be subdivided into low-pressure CVD, high-pressure CVD, substrate-supported catalyst CVD, plasma-enhanced CVD, floating catalyst CVD, and photo CVD, etc.Fig. 3(a) shows a typical process of floating catalyst CVD.37 Different from the traditional CVD technology, the catalyst precursor (ferrocene) is placed into the reaction zone together with the growth promoter (sulfur) and carbon source through a gas carrier transmission and then decomposed into floating nanoparticles at a high temperature, which can act as the catalyst. With the decomposition of the carbon source and the promotion of sulfur, CNTs gradually grow.
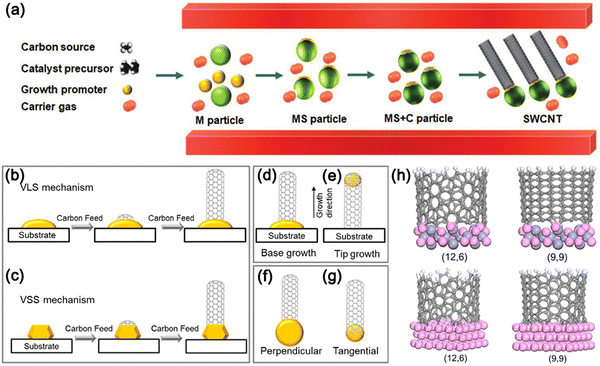 |
| Fig. 3 (a) Reaction process of single-walled CNTs prepared by floating catalyst CVD method. Reproduced from ref. 37 with permission. Copyright 2022, Wiley-VCH. (b)–(g) Various mechanisms of catalyst particles catalyzing the growth of CNTs. (b) VLS mechanism. (c) VSS mechanism. (d) Base growth mechanism. (e) Tip growth mechanism. (f) Perpendicular mechanism. (g) Tangential mechanism. Reproduced from ref. 38 with permission. Copyright 2020, ACS. (h) Different chiral single-walled CNTs catalyzed by Co7W6 and Co catalyst particles. Reproduced from ref. 40 with permission. Copyright 2016, ACS. | |
In order to prepare CNTs or CNFs, metals such as Fe, Co, Ni, and Cu are usually used as catalysts, and hydrocarbon gases as carbon sources. According to different catalyst particles and experimental conditions, researchers have explored various growth mechanisms of CNTs and CNFs (Fig. 3(b)–(g)): CNTs in high temperatures (>800 °C) are generally grown through vapor–liquid–solid (VLS) mechanism because catalyst nanoparticles are in the liquid state in this temperature range (Fig. 3(b)); CNTs can also be grown at a lower temperature through the vapor–solid–solid (VSS) mechanism (Fig. 3(c)); the catalyst particles are usually loaded on substrates or supports. According to the strong or weak interaction between metal and substrate, CNTs will grow in the base growth mode or tip growth mode, respectively (Fig. 3(d) and (e)); based on the different configurations of CNT wall on a catalyst particle, tangential and perpendicular modes are proposed, which is caused by the size difference between CNTs and the catalyst particles (Fig. 3(f) and (g)).38
By controlling the experimental conditions, CNTs with different structures and arrangements such as single-walled CNTs, multi-walled CNTs, helical CNTs, or CNFs can be obtained. These unique structures endow materials with special structures and applications. Yao et al. used carbon fibers as a substrate to grow multi-walled CNTs at low temperatures.39 The CNTs improved the mechanical properties of the carbon/epoxy composites, and the interfacial shear strength and interlaminar shear strength can increase by 30.7% and 32.3%, respectively, because the new interphase formed by the CNT layer improved the stress transfer between the carbon fibers and the adjacent epoxy. Li's group found that the structure of the catalyst can affect the chirality-specific growth of single-walled CNTs (Fig. 3(h)).40 Compared with Co catalysts with low differentiation among single-walled CNTs, Co7W6 catalysts can discriminate single-walled CNTs with different chiralities because the formation energies between various chiral CNTs and catalysts are quite different. Tang et al. explored the dependence of helical CNT structure on the catalyst particle size.41 Small particle size is conducive to improving the purity of helical CNTs. In addition, the helical CNTs show ferromagnetism and high magnetization. Peng's group fabricated vertically-aligned CNT arrays by the CVD process for the first time.42 The aligned films showed excellent MA performance. The absorption frequency peak could be tuned through the variable intersectional angles of the films.
Although CVD technology has proved to be an effective method for synthesizing CNTs and CNFs, and has developed rapidly owing to the advanced equipment and profound mechanism, its shortcomings cannot be ignored. CNTs and CNFs prepared by CVD are generally used as EM functional materials together with the substrate materials because the EM loss mechanism of individual CNTs or CNFs is too single and its conductivity is too high. In addition, it is difficult to load other nanomaterials on the surface of CNTs and CNFs, so the combination of the substrate can provide total benefits of their advantages. CVD needs a high reaction temperature, which limits the selection of some substrates; CVD has a low deposition rate and a thin deposition layer, and it is difficult to deposit locally; gases involved in the reaction and gas by-products after the reaction may have certain toxicity; although CVD can be used to prepare centimeter scale CNTs and CNFs, the length is still not as long as the nanofibers prepared by electrospinning, which limits their application in flexible films or three-dimensional (3D) porous foam structures. These deficiencies and challenges need to be further overcome by researchers.
2.3 Polymerization
The carbon-based nanofibers derived from polymerization nanofibers further flourish this family. The polymerization method has aroused the interest of researchers due to the fast reaction speed, mild reaction conditions, simple process flow, and low requirements for the purity of the reaction monomer, etc. Polymers such as polypyrrole, polyaniline (PANI), and poly(3,4-ethylenedioxythiophene) (PEDOT) have been reported in the synthesis of nanofibers. Polypyrrole nanofibers can be synthesized via chemical polymerization, in which the pyrrole monomer can be oxidized by oxidants during polymerization. Elhassan et al. chose methyl orange as a structure-guiding additive and FeCl3 as an oxidant to prepare polypyrrole nanofibers (Fig. 4(a)).43 Methyl orange with rigid planar hydrophobic structures and hydrophilic end groups tends to aggregate in an aqueous solution. Subsequently, as a precipitant, FeCl3 forms a complex with methyl orange molecules to produce fibrous precipitation. The polypyrrole monomer is oxidized and polymerized on the fibrous precipitation surface, while FeCl3 is reduced to degrade the complex, finally obtaining hollow polypyrrole nanotubes. After microwave calcination, functionalization, the second polymerization of polypyrrole, and microwave calcination, the sea cucumber-like hollow structured CNFs were successfully synthesized. Jiao et al. mixed the pyrrole monomer and ZIFs in a FeCl3 solution. The monomer could fully enter the pores of ZIFs and be transformed into PPy cores under the oxidation of Fe3+, then the PPy cores grew into PPy nanofibers through the nanochannels in ZIFs.44 In addition to iron salts, persulfate can also be used as an oxidant. Sun and Xie et al. used ammonium persulphate as oxidants to obtain PPy nanofibers, which contained no metal ions, have strong oxidation capacity and are convenient for the subsequent treatment.45,46
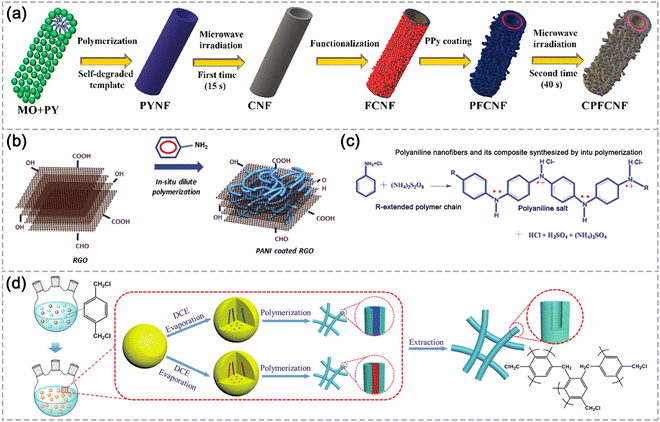 |
| Fig. 4 (a) Schematic diagram of carbon-based nanofibers prepared by polymerization of polypyrrole. Reproduced from ref. 43 with permission. Copyright 2020, Elsevier. (b) In situ polymerization of PANI nanofibers. Reproduced from ref. 48 with permission. Copyright 2018, Elsevier. (c) The formation process of PANI from monomers to nanofibers. Reproduced from ref. 49 with permission. Copyright 2016, RSC. (d) The synthesis process of tubular polymer nanofibers by Friedel–Crafts alkylation in a confined self-condensation system. Reproduced from ref. 54 with permission. Copyright 2019, RSC. | |
PEDOT nanofibers and PANI nanofibers can be synthesized by in situ polymerization. Zhang and Hazarika synthesized PEDOT nanofibers and PANI nanofibers with reduced graphene oxide (RGO) sheets as the substrate, respectively.47 The defects and groups on the RGO sheets’ surface can promote the growth of polymer nanofibers, which can improve the MA properties of RGO sheets (Fig. 4(b)).48Fig. 4(c) shows the formation process of PANI nanofibers from monomers to nanofibers, where the sulphuric acid can act as protonic acid to provide the PH value required for the reaction and be doped into the PANI framework to endow it a certain conductivity.49 Wang et al. grafted ferrite nanoparticles on the PANI nanofibers during in situ emulsion polymerization, which could avoid particle agglomeration and improve EMI shielding performance.50
Friedel–Crafts alkylation reaction can be used to prepare the hyper-crosslinked polymers.51–53 In 2019, Zhang's group reported the method of preparing tubular polymer nanofibers (TPFs) by Friedel–Crafts alkylation in a confined self-condensation system.54 Different from the traditional solution polymerization system for preparing the hyper-crosslinked polymers, the hyper-crosslinking reaction they designed was confined to a single oil phase droplet, which was formed by the collision and coalescence of two droplets. Fig. 4(d) shows the proposed mechanism for the formation of TPFs. 1,2-Dichloroethane (DCE) and polydimethylsiloxane (PDMS) formed a dual oil phase suspension system, polymer monomer alpha,alpha′-dichloro-p-xylene (DCX) and catalyst FeCl3 existed in DCE respectively.54 After the two kinds of DCE droplets collide and merge, the polymerization reaction begins to take place. With the evaporation of DCE, DCX or FeCl3 may crystallize with the volatilization of DCE and act as a template during the polymerization of DCX to PDCX. The TPFs were obtained after the templates were removed by extraction. This work has important reference significance for the preparation of tubular nanofibers and core–shell structural nanofibers for MA materials.55–58 Wang et al. carbonized FeCl3/PDCX nanofibers to prepare tubular magnetic CNFs, where the Fe nanoparticles existed in the tubes.55 The composition, structure, and EM response at different carbonization temperatures were detected. Kang et al. using TPFs, prepared from the confined self-condensation method, and tetrabutyl titanate as raw materials to obtain tubular CNFs/TiO2 hybrids via the hydrolysis and pyrolysis process.59 The pore structure produced during pyrolysis and multiple interfaces could improve the absorbing performance of the material.
Conductive polymer nanofibers obtained by polymerization can be directly used in EM functional materials due to their good conductivity loss. However, non-conductive polymer nanofibers usually need to be converted into carbon-based nanofibers through carbonization to improve dielectric loss, which increases the complexity of the process. On the other hand, although multiple chemical reactions have brought infinite vitality to polymer-derived carbon-based nanofibers, most of the synthesis is still on the laboratory scale, which greatly limits its application potential. Besides, the post-treatment of waste liquid containing acids and metal ions is also an issue to be solved.
2.4 Others
2.4.1 Biomass template method.
Biomass nanofibers have a wide range of sources including sugarcane bagasse, corncob, wood, bamboo, and reeds, which are low-cost, environmentally friendly, and convenient sources. They can be used as templates to prepare carbon-based fibers by means of direct calcination or surface coating. Shi's group took natural collagen nanofibers as a template to prepare hierarchical CNF bundles with closely distributed Fe3O4 nanoparticles through heat treatment.60 Yu's group synthesized CNF@MnO2 composites derived from bacterial cellulose, which showed potential as an asymmetric supercapacitor.61 The structure of the biomass template has an important impact on the structure of the product. By changing different template types or processes, nanofibers, membranes, or foam with various structures can be obtained.
2.4.2 Forcespinning.
Forcespinning technology is a method of preparing nanofibers developed in the past ten years. Different from electrospinning, forcespinning uses centrifugal force instead of electrostatic force to fabricate nanofibers.62 This method can process solutions and melted materials and can produce a variety of polymers nanofibers such as poly-ethylene oxide, PVP, polystyrene, polypropylene, and polylactic acid, and other kinds of materials such as ceramic, metallic or composites nanofibers in large quantities.63 Cremar and coworkers reported CNFs derived from polyvinyl alcohol fibers through forcespinning and calcination, which showed excellent EMI shielding performance and mechanical integrity.64 However, at present, the preparation of EM functional carbon-based nanofibers by forcespinning is still very rare, and further development and exploration are needed.
2.4.3 Blow spinning.
Blow spinning technology is applicable to solution spinning. At the gas–liquid interface, the shear force generated by the flowing gas will refine the liquid droplets extruded from the needle and make the jet move forward. In the process of advancing, the jet continuously evaporates under the action of gas and finally forms nanofibers. Ju et al. fabricated PVA-based porous CNFs by electro-blown spinning.65 PVA was calcined to carbon and PTFE was sacrificed during carbonization. The porous structures can be tuned by changing the loading of PTFE. Wu's group reported a needleless Kármán vortex solution blow spinning system to realize the mass production of nanofibers.66 Kinds of nanofibers such as carbon, ceramics, and polymers were produced on a large scale. This work proves the possibility of preparing high-quality and high-yield nanofibers by blow spinning. At present, the uniformity of carbon-based nanofibers prepared by blow spinning is relatively poor, which may have a great impact on the stability of EM properties. The relevant preparation process needs further improvement.
2.4.4 Wet spinning.
Wet spinning is mainly used to prepare polymer fibers. There are already a variety of polymer fibers prepared by wet spinning, such as cellulose nanofibers,67,68 aramid nanofibers,69 polyvinylidene fluoride nanofibers,70 and PANI fibers.71 Li et al. synthesized PAN/polymethyl methacrylate (PMMA) blend fibers via wet spinning, the CNFs were obtained as the PMMA matrix was removed and the PAN component was transformed to carbon during the carbonization process.72 Wet spinning involves the simple operation and a high production rate, but the diameter of the prepared fibers is usually large, which limits the production of nanofibers.
3. Mechanism of EM wave attenuation
Carbon-based nanofibers can generate EM response and transform EM energy into heat under an alternating EM field, as a result, the EM waves can be attenuated. The response includes electron transport, dipole polarization, magnetic moments, and eddy current, as well as multiple scattering, which are related to the structure and composition of the carbon-based nanofibers. Since Cao's group proposed the theory of dielectric “genes”,22 researchers have a deeper cognition of how to regulate the EM response by tailoring the microstructure of materials. In this section, the EM attenuation mechanism of carbon-based nanofibers is discussed and analyzed.
3.1 Electron transport
Carbon-based nanofibers have certain conductivity because each carbon atom in the graphite carbon can release free electrons. In addition, the rich network structure formed between nanofibers as conductive channels can promote the transport of electrons. The conduction loss generated by electron transport will attenuate EM waves into thermal energy. Cao's group creatively proposed electron-hopping (EHP) and aggregation-induced charge transport (AICT) models to reveal the electron transmission behavior in carbon fibers and multi-walled CNTs (Fig. 5(a) and (b)).73–75 In a continuous fiber structure, electrons can migrate. When encountering defects or interface barriers, electrons can hop. These two models together determine the conduction loss.
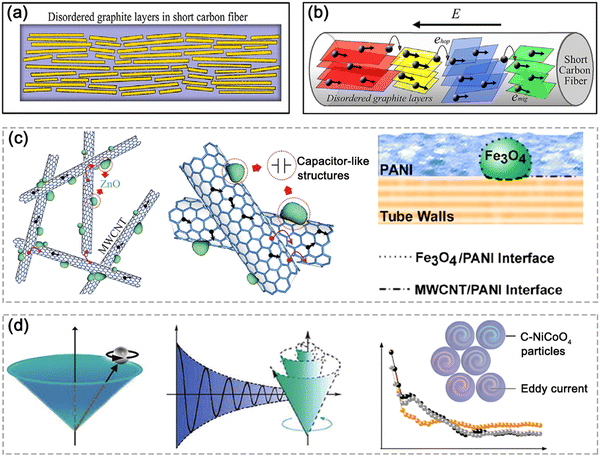 |
| Fig. 5 (a) Structure of carbon fiber. (b) The electron transport behavior in carbon fiber. Reproduced from ref. 73 with permission. Copyright 2010, Elsevier. (c) Schematic diagram of a conductive network, capacitor-like structure, and interfaces. Reproduced from ref. 76 and 77 with permission. Copyright 2014, RSC; Copyright 2012, ACS. (d) Schematic diagram of electron spin, magnetic resonance, and eddy current. Reproduced from ref. 78 and 79 with permission. Copyright 2019, Wiley-VCH; Copyright 2021, Elsevier. | |
3.2 Dipole polarization
These are various defects, polar groups, and heterogeneous interfaces on the surface or inside of nanofibers, which can create dipoles. Under the alternating EM field, the rotation of dipoles lagging behind the change in the EM field will cause polarization relaxation. Cao's group put forward capacitor-like structures to express interfacial polarization for the first time (Fig. 5(c)).76,77 The asymmetric distribution of charges on both sides of the heterogeneous interface is similar to the capacitor structure, which can generate dipole polarization.
3.3 Magnetic moments and eddy current
For MA and EMI shielding, magnetic components in the form of nanoparticles, nanoneedles, nanosheets, and cladding are usually selected in carbon-based nanofibers. The magnetic responses occurring in different frequency bands mainly include domain wall resonance and relaxation, natural resonance, exchange resonance as well as eddy current. Researchers have reported a series of low-dimensional magnets to reveal the influence of morphology on the magnetic domain distribution. For example, the helical domains existed in Fe nanoparticles,80 the strip domains can be detected in Fe3GeTe2 slice,81 and vortex domains can be observed in Co nanoparticles.10 The magnetic domain variations and spin rotations will generate a magnetic loss. The magnetic components can produce magnetic resonance, including natural resonance and exchange resonance (Fig. 5(d)).78,79 Because of the high anisotropy of low-dimensional magnets, they tend to have natural resonance at higher frequencies. In addition, multiple exchange resonances can occur when the magnetic particle size is smaller than the critical size. The magnetic eddy current can also be produced by magnetic components under an alternating EM field, which can be measured by the eddy current coefficient: μ′′(μ′)−2f−1.82 When the coefficient is constant with the changed frequency, the eddy current is dominant in the frequency range.
3.4 Multiple scattering and reflection
Carbon-based nanofibers possess a high aspect ratio, large specific surface area, and more internal space. These multiple surfaces and internal spaces can produce multiple scatter EM waves and attenuate them. Moreover, carbon-based nanofibers can be assembled in many forms, e.g., monolayer film, multilayer film, foam, and aerogel (Fig. 6). EM waves show different propagation behavior in different structures. For monolayer film, the incident EM waves will be reflected, absorbed, and transmitted (Fig. 6(a)).83 The propagation behavior of EM waves in multilayers will be more complicated. Ren's group designed sandwich structural films, in which the upper and lower are absorption layers, and the middle is a reflection layer (Fig. 6(b)).21 The excellent absorption-dominant EMI shielding performance is achieved due to the absorb-reflected-reabsorb process. Ju et al. prepared multiple films, and EM waves can show multiple reflections in the internal gaps between the films (Fig. 6(c)).84 For foams and aerogels, apart from polarization relaxation occurring in microstructures (Fig. 6(d)) and microcurrent loss between nanofibers (Fig. 6(e)), the multi-scattering of EM waves in the cavity of aerogel cannot be ignored (Fig. 6(f)).85 The cavity walls will repeatedly scatter EM waves to enhance the EM dissipation capacity.
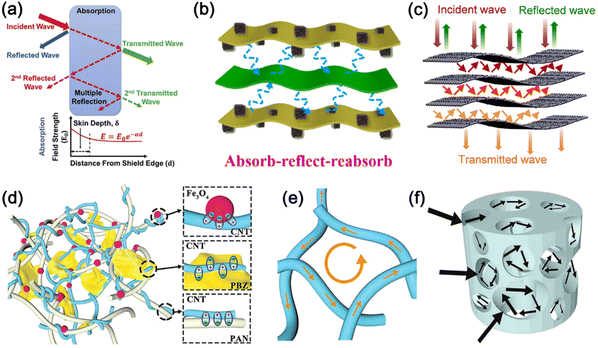 |
| Fig. 6 (a) The reflection, absorption, and transmission of incident EM waves in a single-layer film. Reproduced from ref. 83 with permission. Copyright 2022, Elsevier. (b) The absorption–reflection–reabsorption process of EM waves in a sandwich structural film. Reproduced from ref. 21 with permission. Copyright 2022, Elsevier. (c) Multiple scattering and reflection of EM waves in a multilayer film. Reproduced from ref. 84 with permission. Copyright 2022, Elsevier. (d) The interfacial polarization, (e) microcurrent, and (f) multiple scattering and reflection in an aerogel. Reproduced from ref. 85 with permission. Copyright 2019, Wiley-VCH. | |
3.5 MA and EMI shielding performance
Under the action of various mechanisms of EM wave attenuation, materials will show MA or EMI shielding performance. The MA performance can be measured by RL value, which can be calculated by the following equation:86 | 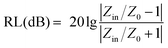 | (1) |
where Z0 and Zin are free space and input impedance. These two can be expressed by: | 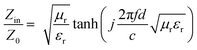 | (2) |
where f is frequency, c is the speed of light, d is the absorber thickness, respectively.
EMI shielding performance can be measured by shielding effectiveness (SE), which can be expressed by:87
| 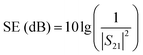 | (3) |
| 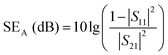 | (4) |
| 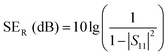 | (5) |
| SE (dB) = SEA + SER | (6) |
where
S11/
S21 represents the reflection/transmission coefficient.
A,
R, and
T are absorption, reflection, and transmission coefficients, respectively. These three can be calculated from
S parameters:
In order to avoid EM pollution caused by secondary reflection, and emphasize the contribution of absorption in the shielding mechanism, the conceptions of green EMI shielding and green index (gs) were proposed by Cao's group for the first time.6 The gs can be expressed by:
| 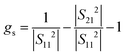 | (10) |
when the
gs > 1, absorption will play a leading role in EMI shielding. The higher
gs value, the greener the EMI shielding materials. Green EMI shielding can be divided into three types: (1) poor green shielding performance (SE > 10 dB and
gs < 1); (2) effective green shielding performance (10 dB < SE < 20 dB and
gs > 1); (3) high-efficiency green shielding performance (SE > 20 dB and
gs >1).
4. Tailoring strategies of carbon-based nanofibers for MA and EMI shielding
CNFs with a single component may not be able to meet the effective attenuation of EM waves. After the discussion in Section 3, it can be concluded that the dielectric “genes” and magnetic “genes” can be regulated to tailor the nanofiber structure to achieve its “selective expression”, that is, controllable EM attenuation performance. In this section, the tailoring strategies of carbon-based nanofibers for MA and EMI shielding are dissected. Considering the different structures of nanofibers, we will expand this part in the order of fiber dimensions.
4.1 Structural tailoring of 1D nanofibers
In the field of EM wave absorption and EMI shielding, most of the current works on carbon-based nanofibers are being carried out around the 1D structures. Structural tailoring on the surface or inside of nanofibers is an effective way to achieve superior EM functions.
4.1.1 Surface structure design.
The functionalized surface of the carbon-based nanofibers makes various modification processes possible, including ALD, hydrothermal reaction, CVD, electroplating, electroless plating, electrostatic self-assembling, magnetron sputtering, in situ polymerization, and dipping. These kinds of methods give more possibilities to the structure of the carbon-based nanofibers and provide more EM loss mechanisms. The composition and structure of pure CNFs are too single, which provides a single EM attenuation mechanism. After the surface structure design, with the implantation of low-dimensional nanomaterials, the surface structure of the nanofiber becomes complex. Compared with CNFs with smooth surfaces, the low-dimensional nanomaterials on nanofiber surfaces can show multiple-scatter EM waves. In addition, the combination of carbon fiber and other components promotes the formation of heterogeneous interfaces, introducing more dielectric or magnetic “genes”, and promoting the effective integration of various attenuation mechanisms.
Coating the nanofiber surface is a simple way to control the EM properties of CNFs, which can reduce the high conductivity of CNFs, increase heterointerfaces, and optimize impedance matching. Qin's group successively deposited the Al2O3 layer and Fe3O4 layer on the surface of carbon nanocoils by the ALD process, which brings polarization relaxation and magnetic response to carbon nanocoils (Fig. 7(a) and (b)).88 Although the pristine carbon nanocoils can provide strong conduction loss, they have no MA ability because the too-high complex permittivity leads to impedance mismatch. The outer Al2O3 layer and Fe3O4 layer bring polarization relaxation and magnetic response. In addition, the thickness of the deposition layers can be precisely controlled on the nanometer scale by the varying cycle numbers. At the same time, the permittivity and permeability are also tuned with the changed thickness of the deposition layers (Fig. 7(c) and (d)). As a result, the MA performance is optimized. Fe3O4/Al2O3/carbon nanocoils nanostructures with 24 nm Fe3O4 layer show a minimum reflection loss of −40.3 dB (Fig. 7(e)). The multilayer core–shell structures and dielectric-magnetic multiple loss mechanisms are the critical factors to absorb EM waves.
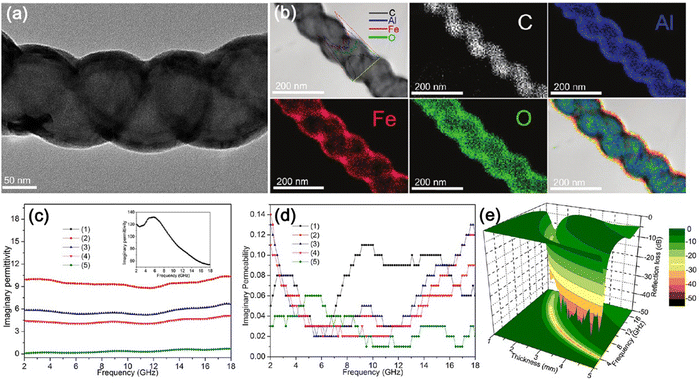 |
| Fig. 7 (a) The TEM image of Fe3O4/Al2O3/carbon nanocoils. (b) The elemental mapping images. (c) The imaginary permittivity and (d) imaginary permeability of Fe3O4/Al2O3/carbon nanocoils. (e) The 3D RL plots versus thickness and frequency. Reproduced from ref. 88 with permission. Copyright 2012, ACS. | |
The introduction of low dimensional nanostructures such as zero-dimensional (0D) nanoparticles and 2D nanosheets on the surface of carbon-based nanofibers are helpful in implanting dielectric and magnetic “genes”, as well enhancing multiple scattering. The EM response can be accurately controlled on the microstructures. Various low-dimensional materials enrich the structure of carbon-based nanofibers, and the dielectric and magnetic “genes” can be selectively assembled. The conductive networks, dipoles, and magnetic response can work together under the action of an EM field to dissipate EM waves. Yu's group fabricated graphene nanofibers by the CVD method, where the 2D graphene sheets grew vertically on the nanofiber surface.11 The product inherits the high conductivity (1.2 × 105 S m−1) advantage of CNFs, while the tightly-arranged graphene sheets enhance multiple scattering and polarization effects. The EMI SE is 56 dB and SSE/t (dB cm2 g−1), SE/(d × t) can reach 60
932 dB cm2 g−1. Xu et al. prepared MnOx nanoflakes-coated CNFs through a hydrothermal process.89 The composite nanofibers show significantly enhanced MA performance than pure CNFs and most single-phase manganese oxides. The MnOx nanoflakes promote multiple scattering of EM waves, provide magnetic loss and interfacial polarization, and optimize impedance matching. Liao et al. coated MoS2 on MgFe2O4/MgO/C fibers surface through the hydrothermal method.15 Compared with the MgFe2O4/MgO/C fibers with low EM waves loss capacity and poor impedance matching, the MgFe2O4/MgO/C@MoS2 composites showed enhanced MA performance. The minimum RL value could reach −56.94 dB and EAB is 3.9 GHz with a thickness of 2.7 mm. The introduction of 2D MoS2 improved dielectric loss ability and ameliorated impedance matching. The heterointerfaces formed between MoS2 and MgFe2O4/MgO/C fibers promoted interface polarization, the defects on MoS2 induced dipole polarization, and MoS2 improved the dielectric-magnetic synergy effect between magnetic MgFe2O4.
4.1.2 Internal structure design.
The structural advantage of nanofiber is not only reflected in the structural design of the surface, but also in the structural construction of its interior. Porous nanofibers, hollow nanofibers, and core–shell structure nanofibers have been prepared through internal structure design. The internal hollow or porous structure can reduce the density, increase the inner space, and can be used as a container to contain or load other components.90 The core–shell structure introduces more material components into the nanofiber and increases the heterointerfaces and defects. Structural design inside the nanofibers can better retain their 1D structure and maintain a good conductive network. In addition, with the nanofiber matrix as the template, the reaction takes place inside, and the resulting dielectric and magnetic “genes” can be well protected by the carbon shell. For example, transition metal magnetic nanoparticles can be protected from water and oxygen corrosion.
Coaxial electrospinning, as an improved electrospinning technology, is favored by researchers because it is easy to prepare core–shell nanofibers. Jin and coworkers synthesized hollow carbon-based tubes through the coaxial electrospinning and solvothermal process (Fig. 8).12 In the first step, they prepared nanofibers with skin-core structure via coaxial electrospinning, in which the core is TiO2/silicone oil, and the skin is Co(Ac)2/PAN components. The silicone oil was removed to form hollow tubular structures by carbonization. After solvothermal and second carbonization, TiO2@Co/C@Co/Ni carbon tubes were obtained (Fig. 8(a)–(d)). On the one hand, the multilayer structure facilitates both the incidence and dissipation of EM waves, because the impedance matching and EM attenuation ability could be controlled with the layer from outside to inside. On the other hand, various components provided multiple loss mechanisms. The gradient heterostructures could generate interfacial polarization loss, and the 1D magnetic network could enhance magnetic loss. The integration of various mechanisms, together with the exquisite structure design, determines the outstanding MA performance (RL = −54 dB, EAB = 6 GHz) (Fig. 8(e)–(g)). This work proved the possibility of precise tailoring of carbon-based nanofibers as a template to achieve efficient electromagnetic responses.
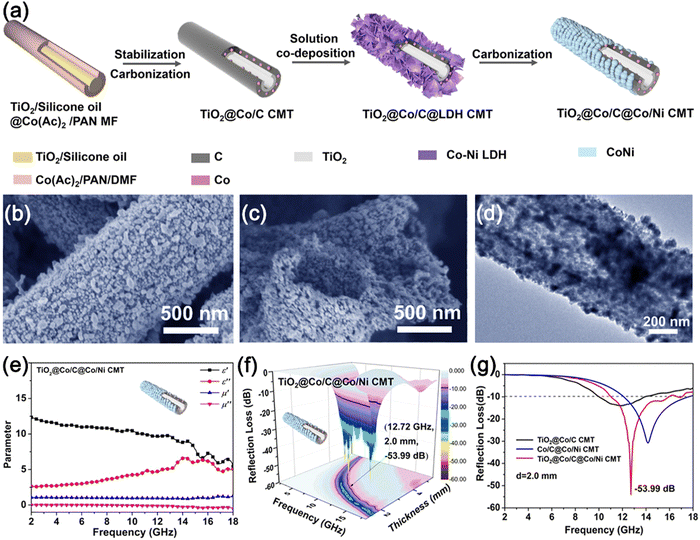 |
| Fig. 8 (a) The synthesis process of TiO2@Co/C@Co/Ni carbon tubes. (b) and (c) SEM images and (d) a TEM image of TiO2@Co/C@Co/Ni carbon tubes. (e) EM parameters. (f) 3D RL plots and (g) 2D RL curves. Reproduced from ref. 12 with permission. Copyright 2021, ACS. | |
Creating hollow structures inside the fiber to accommodate other nanomaterials is also a workable proposition for designing hybrid carbon-based nanofibers. Zhang's group constructed magnetic hybrid CNFs with core–shell structures via three steps.91 In the first step, tubular FeCl3/PDCX nanofibers were prepared in a confined self-condensation system. After carbonization, FeCl3/PDCX nanofibers were degraded to tubular CNFs with Fe nanoparticles remaining inside. The tubular structure of CNFs becomes the carrier for containing Fe nanoparticles. Finally, the TiO2 layer was formed on the outer carbon layer through the hydrolysis process and Fe/Fe3O4@C@TiO2 nanofibers with three-layer core–shell structures were obtained. The inter Fe/Fe3O4 layer provides magnetic loss, a large number of interfaces, and defects. The TiO2 layer with different loadings can manipulate the EM parameters, which can act as a transition layer to decrease the reflection of EM waves. The three-layer structure gives the material excellent MA performance, its minimum RL value is −45 dB with a thickness of 1.6 mm.
4.2 Structure design of 2D films and papers
Carbon-based nanofibers can be assembled into films and papers in a variety of ways including vacuum-assisted filtration, hot pressing, and spraying. Firstly, the films or papers retain the original nanofiber network structure completely, which ensures their certain conductivity. Secondly, Thanks to the large area and rich network structure of the films or papers, other advanced low-dimensional materials, and exquisite structural design can be easily introduced in carbon-based nanofibers, which give more dielectric “genes” and magnetic “genes” to films. Based on the intrinsic EM response characteristics of different components, the films and papers can achieve efficient EM attenuation due to the integration of multiple mechanisms. Multilayer structures made of films have gained researchers' attention due to their excellent EM wave attenuation capability. There is space for multiple scattering of EM waves between every two layers. More importantly, multilayer structures with different EM characteristics for each layer can effectively adjust the incidence, reflection, and absorption behavior of EM waves because the absorption and reflection intensity of EM waves in each layer are different, thus achieving efficient EMI shielding or MA properties. By adjusting the order of layers or changing the EM response characteristics of one layer, the attenuation behavior of EM waves can be controlled. This provides more abundant regulation means for the EM wave attenuation of films.
Wan et al. reported an ultrathin-densified CNT film by a purification and densification process.92 The impurities in the pristine CNTs were removed, the CNT film was densified, and a strong CNT–CNT attraction was formed. These factors led to ultrahigh conductivity (>9 × 105 S m−1), superior EMI SE (101 dB), and excellent mechanical strength. In addition, the CNT film showed reliability in strong acid/alkali, high temperature, and high humidity environments.
Although the single-layer film with high conductivity can achieve excellent EMI shielding performance, it mainly depends on high-intensity reflection, which will cause strong secondary EM pollution in the environment. The design of multilayer films helps to solve this problem and realize green EMI shielding dominated by absorption because of the multiple transmission behaviors of EM waves between multilayer structures. Chen et al. fabricated aligned carbon networks derived from cotton pads through pyrolysis (Fig. 9(a)–(c)).93 The EMI-shielding performance at different rotation angles was explored (Fig. 9(d) and (e)). The double-layer carbon networks with a spacing of 9.46 mm show approving EMI SE (>30.7 dB) and ultralow reflection coefficient (R ∼ 0.1) within the range of 0–180° of rotation angles (Fig. 9(f)). Each layer itself has a certain absorption capacity for EM waves. Additionally, the EM waves can be reflected and transmitted at the upper layer, then the transmitted EM waves will continue to move forward and be reflected by the lower layer (Fig. 9(g)). The second reflected EM waves have the same polarization plane as the EM waves reflected by the upper layer. However, there is a 180° phase difference between the two since the distance between the two layers is equal to a quarter wavelength (Fig. 9(h)). This leads to effective interference with EM waves in the double-layer structure. The simulation results calculated from an equivalent circuit (Fig. 9(i)) also verified the accuracy of the experimental results.
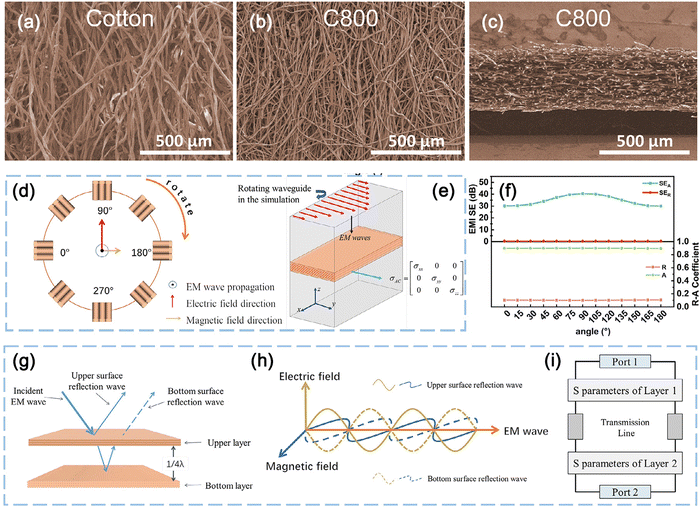 |
| Fig. 9 (a) and (b) SEM images of carbon networks. (c) The cross-section view of carbon networks. (d) The schematic diagram for measuring EMI shielding performance of carbon networks with variable angles. (e) Full-wave simulation model of carbon networks. (f) The EMI SE, absorption coefficient (A), and reflection coefficient (R) of double-layer carbon networks at different angles. (g) EMI shielding mechanism of low reflection. (h) The phase variation of waves which are reflected by two layers. (i) The equivalent circuit model. Reproduced from ref. 93 with permission. Copyright 2022, Elsevier. | |
4.3 Assembly of 3D nano-architecture
3D foams have been one of the most popular EM attenuation materials. The 3D porous structure assembled by carbon-based nanofibers has preeminent porosity, large specific surface area, small density, high conductivity, and good flexibility. Compared with multilayer structures, the foam structure has more internal area, more porous structures, and more dielectric and magnetic “genes” implantation sites. A large number of porous structures are one of the characteristics of foams. The porous structures provide large internal space and abundant internal walls, which increase the transmission path of EM waves and the chance of multiple scattering. The porous surface promotes the incidence of EM waves and improves the impedance matching between the material surface and air. The interconnected network structure ensures a certain conductivity. At the same time, various nanostructures can be loaded on a great number of network structures and inner walls, which help to increase the interfaces, defects, and magnetic responses. The effective introduction of dielectric “genes” and magnetic “genes”, combined with the special structural advantages of foams, can achieve strong EM attenuation. In essence, foam is an ideal high EM absorption structure with some conduction loss, strong polarization relaxation, matched magnetic loss, good impedance matching, and high attenuation ability. In addition, the flexibility of many foams is conducive to their application in flexible wearables and some special environments. The remarkable structural advantages of the foam structure make it possible to apply it to EM functional materials and wearable electronic devices.
Foams and aerogels composed of carbon-based nanofibers are usually prepared by the freeze-drying method, which can not only maintain the structural characteristics of nanofibers but also form a porous foam structure. Fei et al. used bacterial cellulose and zeolitic imidazolate frameworks-67 (ZIF-67) to synthesize Co/C particle-embedded CNF aerogels through freeze-drying and calcination (Fig. 10(a)).16 The aerogels are lightweight, in which the Co/C particles still retain the polyhedron structure of the ZIF-67 precursor, and the carbon fiber network structure connects each particle (Fig. 10(b)–(e)). The 3D cross-linked network composed of CNFs acts as a framework, and the Co/C particles act as active sites of “genes” to produce dielectric and magnetic “genes”. The conductive network, porous structures, plenty of interfaces, magnetic Co particles, and EM synergistic effect are in favor of the efficient absorption of EM waves (Fig. 10(f)). Aerogels exhibit superior EMI green shielding, and the EMI SE can reach 56 dB with A coefficients over 0.79, i.e., the gs are ∼3.76 (Fig. 10(g) and (h)). This work shows the new inspiration for CNF aerogels or foams to design advanced EM-functional materials and devices.
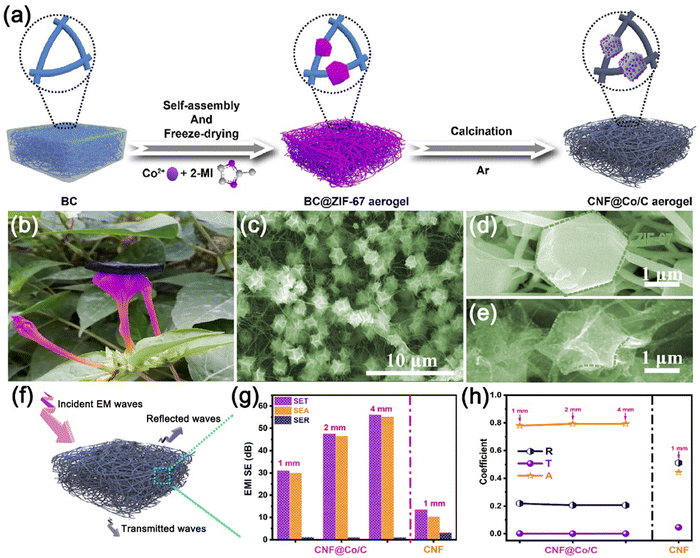 |
| Fig. 10 (a) The preparation process of the CNF@Co/C aerogel. (b) Photograph of the CNF@Co/C aerogel on a petal. (c) SEM image of the CNF@Co/C aerogel. SEM image of (d) bacterial cellulose@ZIF-67 and (e) CNF@Co/C. (f) The EMI shielding mechanism. (g) EMI SE of the CNF@Co/C aerogel. (h) A, R, and T coefficients of the CNF@Co/C aerogel at different thicknesses. Reproduced from ref. 16 with permission. Copyright 2020, Elsevier. | |
Shui et al. reported multifunctional CNF–SiC nanowire aerogel by using bacterial cellulose as scaffolds, which showed a high yield, excellent mechanical strength and stability, superior MA performance, and low thermal conductivity.94 The improper electrical conductivity of the pure CNF aerogel leads to interfacial impedance mismatch and unsatisfactory EM dissipation ability. In CNF–SiC aerogel structures, conduction “genes” and polarization “genes” are selectively expressed. The heterostructure formed by CNF and SiC generates interface polarizations, the existence of CNF ensures the conductivity of the composites, and the introduction of SiC nanowire ameliorates the impedance matching, hence, the MA performance was improved. The minimum RL value can reach −53.3 dB and the thermal conductivity was only 0.046 W m−1 K−1 at 200 °C.
5. Carbon-based nanofibers for EM devices
Various crystal structures and electronic characteristics of the carbon-based nanofibers are key factors affecting the EM response and energy conversion. The combination of carbon-based nanofibers and low-dimensional nanomaterials enriches the dielectric “genes” and magnetic “genes”, and the multiple EM response mechanisms promoting the MA or EMI shielding ability of nanofibers. Furthermore, thanks to their 1D structure with a large aspect ratio, the integration and assembly of nanofibers make it possible for materials to construct nanoarchitectures such as multilayer films and porous foams. These nanoarchitectures are easy to be processed into various shapes, with good flexibility, certain strain capacity, multiple EM response characteristics, and excellent environmental stability. These advantages contribute to the application of EM devices in various special or extreme environments such as waterproof, inflaming retarding, piezoresistive sensing, stress sensing, electronic communication, microwave driver, triboelectric generator, infrared stealth, and thermal management.
Carbon-based nanofibers have proven their potential applications in the field of electronic communications. Song et al. designed a flexible radio-frequency antenna electrode with a CNT film (Fig. 11).95 The CNT film was prepared by direct spinning, which can be readily cut into various shapes by laser (Fig. 11(a)). The CNTs are intertwined with each other to help improve the conductivity, mechanical strength, and flexibility (Fig. 11(b)). The monopole patch radio-frequency antenna was fabricated with CNT film as the patterned electrode and copper plate as a ground plane (Fig. 11(c)). By changing the dimensions of the pattern, the minimum return loss could reach −37.8 dB at 1.95 GHz. The resonant frequency could be controlled by controllable dimensions, which means this antenna can be applicated in the Bluetooth and Wi-Fi frequency range. In addition, the CNT film can completely wrap the ball without any wrinkles, showing good flexibility (the insert in Fig. 11(d)). The CNT-based antenna exhibited an outstanding radiation efficiency (85.6%), high average (−0.82 dBi) and peak gain (4.97 dBi) (Fig. 11(e)). Compared with highly-conductive metal films, CNT films not only have the advantages of the former but also possess the characteristics of light weight, corrosion resistance, low price, good flexibility, etc., showing stronger competitiveness on flexible radio-frequency antenna electrodes.
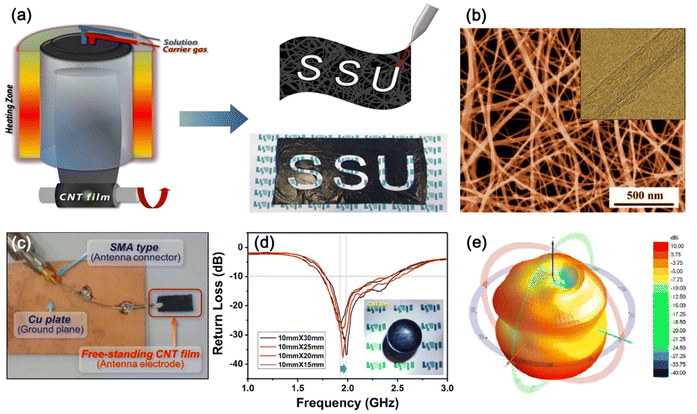 |
| Fig. 11 (a) The preparation process of CNT film and the digital image of the CNT film engraved by a laser. (b) An SEM image of the CNT film (the insert is a TEM image of a single CNT). (c) CNT-based monopole patch RF antenna. (d) The return loss of CNT-based antennas with different dimensions. (e) The 3D radiation plots of CNT-based RF antennas. Reproduced from ref. 95 with permission. Copyright 2022, Elsevier. | |
EM wearable devices show fascinating properties due to their flexibility, convenience, and portability, which have great application potential in the field of electronic communication, healthcare, aerospace, and astronautics. Song et al. reported a wearable EM waves absorption cloth in situ by employing carbon materials into a nonwoven matrix, in which the RGO-supported CNT architecture was assembled on non-woven skeletons (Fig. 12).17 The wearable cloth showed excellent flexibility, that it could be straightened, twisted, or processed into various shapes (Fig. 12(a)–(d)). Its MA performance measured by the arch method exhibited a broad EAB of 8.3–14.5 GHz with a thickness of 4 mm (Fig. 12(e)–(g)). The absorption performance remained relatively stable at different angles. The wearable cloth can be bent freely and return to its original shape after stress removal (Fig. 12(h)). The dimension and EM response of the cloth changed little after 200 cycles of repeated bending, which means it has outstanding durability. In this cloth, the RGO-supported CNT architecture offered sufficient dielectric loss and scattering path of EM waves. Moreover, the modification of CNT on the interface of RGO could tailor the impedance conditions to satisfy the ideal matching regions for generating a quarter-wavelength loss. This work provides meaningful inspiration for the design and innovation of wearable EM devices.
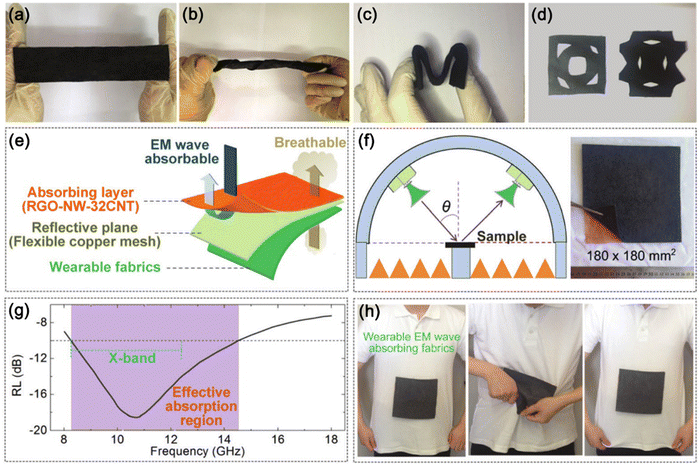 |
| Fig. 12 (a)–(d) Photographs of rGO–NW–CNT architecture with different shapes. (e) Structure of wearable cloth. (f) The measurements of wearable cloth by the arch method. (g) The RL values of wearable cloth. (h) The mechanical deformation and recovery of wearable cloth. Reproduced from ref. 17 with permission. Copyright 2017, RSC. | |
6. Overview and outlook
The various preparation methods, tailoring means, and assembly strategies of carbon-based nanofibers have greatly enriched their applications in the field of EM functional materials, especially in EM multifunctional materials and EM devices. Carbon-based nanofibers show great potential in wireless communication, electronic components, and wearable devices. The future opportunities and challenges of EM functional carbon-based nanofibers are detailed below.
6.1 New fabrication methods
At present, the workmanship of preparing carbon-based nanofibers by electrospinning, CVD, polymerization, and other methods is increasingly maturing, and the relevant mechanisms of synthesis are explored in detail. Carbon-based nanofibers prepared by various technologies show excellent EM attenuation performance, as shown in Tables 1 and 2. With the amelioration of the process and the update of equipment, the size, structure, composition, and arrangement of carbon-based nanofibers can be precisely tailored through experimental parameters. Different from traditional electrospinning, coaxial electrospinning can easily prepare skin-core structural nanofibers, which has recently attracted researchers’ attention. By exploring the influence of catalyst particles on the mechanism of the growth of single-walled CNTs, researchers can control the chirality of CNTs. Through an in-depth study of the polymerization mechanism, hollow or solid polymer nanofibers have been prepared. In addition, combined with other subsequent processes, such as carbonization, ALD, and hydrothermal reactions, crystal engineering, and pore engineering can be implemented. This provides more opportunities to regulate the EM response of carbon-based nanofibers.
Table 1 Summary of carbon-based nanofibers for MA over reported works
Preparation |
Materials |
Type |
Ratio (wt%) |
Thickness (mm) |
RL (dB) |
EAB (GHz) |
Ref. |
Electrospinning |
Fe3C/N-doped CNFs |
Nanofiber |
30 |
1.5 |
−54.5 |
2.2 |
100
|
Electrospinning |
NiFe@CNF |
Nanofiber |
30 |
1.4 |
−49.5 |
4.72 |
101
|
Electrospinning |
C–NiCo2O4 |
Nanofiber |
50 |
1.9 |
−52.7 |
5.2 |
79
|
Electrospinning |
TiO2/C |
Nanofiber |
15 |
2.0 |
−74.5 |
6.7 |
102
|
Electrospinning |
Ni@C@Co |
Nanofiber |
15 |
4.0 |
−45.9 |
5.9 |
103
|
CVD |
Carbon fiber/SiC/Si3N4 |
Film |
— |
2.0 |
−20.3 |
2.3 |
104
|
CVD |
FeCo–C/CNTs |
Nanotube |
20 |
2.0 |
−79.2 |
6.3 |
105
|
CVD |
Fe3C/CNT |
Nanotube |
— |
— |
−21.5 |
5.7 |
106
|
CVD |
CNT/carbon fiber |
Nanotube |
68 |
2.45 |
−56.1 |
3.6 |
107
|
CVD |
CNT/MXene |
Nanotube |
40 |
2.5 |
−52.3 |
2.16 |
108
|
Polymerization |
CNF/Co/nanoporous carbon |
Nanofiber |
8 |
2.0 |
−54.1 |
6.2 |
56
|
Polymerization |
Fe/Fe3O4@TCNFs@TiO2 |
Nanofiber |
15 |
1.6 |
−44.8 |
3.7 |
91
|
Polymerization |
Tubular CNF/Fe |
Nanofiber |
— |
2.2 |
−47.3 |
6.5 |
55
|
Biomass template |
Fe3O4/CNF |
Nanofiber |
80 |
2.0 |
−36 |
5.4 |
60
|
Biomass template |
CNT/carbon |
Foam |
20 |
2.11 |
−55.4 |
2.35 |
109
|
Table 2 Summary of carbon-based nanofibers for EMI shielding over reported works
Preparation |
Materials |
Type |
Thickness |
SE |
Band |
Ref. |
Electrospinning |
TaC/Fe3C–Fe carbon |
Film |
180 μm |
46.4 |
8.2–12.4 |
110
|
Electrospinning |
CNF/silicone |
Film |
0.6 mm |
∼100 |
8.2–12.4 |
111
|
Electrospinning |
Co/C |
Film |
250 μm |
49 |
8.2–12.4 |
112
|
Electrospinning |
TiN/Co@carbon |
Film |
180 μm |
93 |
8.2–12.4 |
84
|
Electrospinning |
ZrO2@carbon |
Film |
0.72 mm |
94 |
12–18 |
113
|
CVD |
CNTs/CNFs@C |
Foam |
5 mm |
∼27 |
18–26.5 |
114
|
CVD |
CoFe2O4/MWCNT/graphene |
Film |
3.5 mm |
49.6 |
8.2–12.4 |
115
|
CVD |
C–Fe–CNTs |
Film |
— |
47.4 |
18–26.5 |
116
|
CVD |
C@SiO2–CNT/PDMS |
Foam |
2 mm |
61.3 |
8.2–12.4 |
117
|
CVD |
CNT |
Foam |
1 mm |
84 |
8.2–12.4 |
118
|
CVD |
CNT/Fe3C/carbon fiber |
Film |
2 mm |
80 |
8.2–12.4 |
119
|
Biomass template |
TiC/carbon |
Foam |
2 mm |
72.9 |
8.2–12.4 |
120
|
Biomass template |
Carbon |
Foam |
2 mm |
93.1 |
8.2–12.4 |
121
|
Biomass template |
CNF@Co/C |
Foam |
4 mm |
56.1 |
8.2–12.4 |
16
|
Biomass template |
Carbon |
Film |
— |
49.3 |
8.2–12.4 |
93
|
With the upgrading of synthesis technology and related equipment, other methods for preparing carbon-based nanofibers are also in full bloom, such as blow spinning, forcespinning, and biomass template methods, which have the potential to achieve large-scale production. Compared with the above methods, these methods have high production efficiency and low requirements for equipment and will have a bright development prospect in the future.
However, the pollution of waste gas and liquid caused in the production process is still a problem to be solved, which is harmful to the production environment and operators. In addition, the manufacturing cost of nanofibers is high due to the high energy consumption processes such as high-temperature treatment and inert gas protection. These two factors restrict large-scale production.
6.2 New tailoring strategies and mechanisms
How to regulate the EM response is the key problem for improving the EM attenuation performance. The proposal of many important physical models and formulas such as the EHP model and AICT model for electron transport, equivalent-circuit model and capacitor-like model for dipole polarization, the synergy, and competition between electron transport and dipole polarization, four principles (interface scattering, microcurrent attenuation, microantenna radiation, and dielectric relaxation) for EM energy conversion, have provided guidance to design EM functional materials. Many works have followed these mechanisms to design materials under control.
The proposition of the dielectric “genes” theory enables researchers to better understand the essence of the EM response. The polarization “genes” can be tailored through defects, polar groups, and interfaces, while the conduction “genes” can be tailored through the conductive network formed by electrons migrating and hopping. By regulating dielectric “genes”, the EM response of materials can be manipulated. Moreover, the magnetic “genes” can also be tailored, which is generated by the spin motion of valence electrons located in the d orbital. The magnetic “genes” will cause natural resonance, exchange resonance, and eddy current loss, i.e., magnetic loss. Magnetic components can be easily implanted in carbon-based nanofibers via various ways to provide magnetic loss and improve impedance matching. The EM response characteristics of nanofibers can be determined through the selective expression of dielectric “genes” and magnetic “genes”.
Although these mechanisms have become powerful tools for us to design EM functional materials, they still need to be innovated. The scientific nature of the EM response should be further revealed from the perspective of physics and mathematics. The contribution of multiple scattering and reflection, dielectric-magnetic synergy, magnetic resonance, and magnetic eddy current need to be quantified and calculated.
6.3 New materials and structures
In recent years, the development and utilization of various new materials have provided a variety of options for carbon-based nanofibers to compounds. Since MXene entered this field in 2016, it has rapidly become a star material among EM functional materials.96 MXene possesses 2D nanostructure, metallic conductivity, abundant surface functionalities, and negatively charged surfaces, which are the advantages of combining with carbon-based nanofibers to prepare EM functional materials. MOF-based materials have been sought after due to their distinctive crystal structure and electronic state. MOFs with various nanostructures embedded in carbon-based nanofibers bring plentiful interfaces and pore structures. The dielectric “genes” and magnetic “genes” are planted and the EM response can be clipped. Furthermore, other low-dimension advanced materials such as magnetic quantum dots, MoS2, and g-C3N4 have proven their worth in carbon-based nanofibers.
As a carrier or a template, carbon-based nanofibers can accommodate multiple micro/nano-structures e.g. core–shell structure, skin-core structure, porous structure, and hollow structure, which increase heterointerfaces, defects, and groups. Meanwhile, the multiple scattering and reflection can be also intensified. More importantly, carbon-based nanofibers can be prepared into 2D films or assembled into 3D architectures. These structures can regulate the propagation behavior of EM waves and achieve efficient attenuation.
Nevertheless, the assembly mechanism of materials is not clear, and the relationship between microstructure and performance remains to be explored. The design philosophy, modification, regulation, and performance of new materials need to be discovered continuously.
6.4 New functions and devices
Materials with single performance are difficult to be used in harsh or complex environments. It is an inevitable trend for EM materials to develop into multifunctional materials. Many works have been performed in this direction, such as flexibility, hydrophobicity, flame retardancy, transparency, piezoresistive sensing, stress sensing, Joule heating, corrosion resistance, electronic communication, triboelectric nanogenerator, and microwave actuation. This shows an attractive prospect for the Internet of Things system, wireless communication, and wearable devices.
Integration of multiple functions promotes the development of EM devices. EM devices can realize multiple functions through the EM response, but also have high sensitivity, good stability, wide frequency, and competent durability. Ferromagnetic alloys have been reported to prepare multi-degree-of-freedom robots, which can be driven by microwaves at 2.47 GHz;97 MXene antennas show high-power radiation, wide frequency ranges, excellent flexibility, and scalability, which is promising for integrated radio-frequency devices.98 Graphene-based smart contact lenses show excellent EMI shielding performance and dehydration protection properties.99 CNF-based wireless sensor tag has been designed to detect H2S gas, which has a large detection range, a long operating range, and excellent cycle stability.19 Carbon-based nanofibers with multiple EM responses, high conductivity, good flexibility, various crystal structure, sophisticated internal structure, favorable storability, and stability, have an infinite and broad development prospect in EM devices.
Most studies have focused on the frequency range of 2–18 GHz. However, the operating frequency of many electronic components and devices in the actual environment is not within this range. Therefore, it is necessary to explore the EM response and performance of EM devices in other frequencies including MHz, GHz, THz, and infrared bands. Besides that, the assembly principle of EM devices, and the close relationship between material microstructure and device performance are also challenges for future research.
7. Conclusions
In summary, we reviewed the research progress on carbon-based nanofibers for EM functional materials and devices. The fabrication methods were summarized, and the relevant synthesis mechanisms were analyzed in detail. In addition, the design strategies of different nanofiber structures for MA and EMI shielding were dissected, and the relationship between microstructure and EM response was revealed. More importantly, we highlighted the potential applications of carbon-based nanofibers in multifunctional EM devices, including patch antennas, microwave drivers, smart lenses, sensors, and wearable devices. Finally, we rationally evaluated the current opportunities and problems and wisely predicted the challenges and trends, which can point out the direction for future research.
Conflicts of interest
The authors declare no conflicts of interest.
Acknowledgements
Financial support from the National Natural Science Foundation of China (Grant No. 52273257, 52177014, 51977009, and 11774027) is gratefully acknowledged.
References
- J. C. Shu, S. Q. Zhu, W. Q. Cao and M. S. Cao, Hierarchical C/Co3O4 nanoarray on a nickel substrate integrating electromagnetic and thermal shielding, Mater. Chem. Front., 2021, 5, 6553 RSC.
- Y. D. Xu, Z. Q. Lin, Y. Q. Yang, H. J. Duan, G. Z. Zhao, Y. Q. Liu, Y. G. Hu, R. Sun and C. P. Wong, Integration of efficient microwave absorption and shielding in a multistage composite foam with progressive conductivity modular design, Mater. Horiz., 2022, 9, 708–719 RSC.
- S. Y. Zhou, G. H. Zhang, Z. Q. Nie, H. Z. Liu, H. H. Yu, Y. X. Liu, K. X. Bi, W. P. Geng, H. G. Duan and X. J. Chou, Recent advances in 3D printed structures for electromagnetic wave absorbing and shielding, Mater. Chem. Front., 2022, 6, 1736–1751 RSC.
- J. Y. Wang, M. Yang and D. Wang, Progress and perspectives of hollow multishelled structures, Chin. J. Chem., 2022, 40, 1190–1203 CrossRef CAS.
- X. X. Zhou, Y. Wang, C. C. Gong, B. Liu and G. Wei, Production, structural design, functional control, and broad applications of carbon nanofiber-based nanomaterials: a comprehensive review, Chem. Eng. J., 2020, 402, 126189 CrossRef CAS.
- X. X. Wang, J. C. Shu, W. Q. Cao, M. Zhang, J. Yuan and M. S. Cao, Eco-mimetic nanoarchitecture for green EMI shielding, Chem. Eng. J., 2019, 369, 1068–1077 CrossRef CAS.
- D. Q. Zhang, T. T. Liu, J. C. Shu, S. Liang, X. X. Wang, J. Y. Cheng, H. Wang and M. S. Cao, Self-assembly construction of WS2–rGO architecture with green EMI shielding, ACS Appl. Mater. Interfaces, 2019, 11, 26807–26816 CrossRef CAS PubMed.
- A. Puthiyedath Narayanan, K. N. Narayanan Unni and K. Peethambharan Surendran, Aerogels of V2O5 nanowires reinforced by polyaniline for electromagnetic interference shielding, Chem. Eng. J., 2021, 408, 127239 CrossRef CAS.
- K. Sushmita, D. Ghosh, S. Nilawar and S. Bose, Absorption dominated directional electromagnetic interference shielding through asymmetry in a multilayered construct with an exceptionally high green index, ACS Appl. Mater. Interfaces, 2022, 14, 49140–49157 CrossRef CAS PubMed.
- Z. C. Wu, K. Pei, L. S. Xing, X. F. Yu, W. B. You and R. C. Che, Enhanced microwave absorption performance from magnetic coupling of magnetic nanoparticles suspended within hierarchically tubular composite, Adv. Funct. Mater., 2019, 29, 1901448 CrossRef.
- J. Zeng, X. X. Ji, Y. H. Ma, Z. X. Zhang, S. G. Wang, Z. H. Ren, C. Y. Zhi and J. Yu, 3D graphene fibers grown by thermal chemical vapor deposition, Adv. Mater., 2018, 30, 1705380 CrossRef PubMed.
- C. Jin, Z. C. Wu, R. X. Zhang, X. Qian, H. L. Xu and R. C. Che, 1D electromagnetic-gradient hierarchical carbon microtube via coaxial electrospinning design for enhanced microwave absorption, ACS Appl. Mater. Interfaces, 2021, 13, 15939–15949 CrossRef CAS PubMed.
- X. Tian, Y. Wang, F. X. Peng, F. Huang, W. Tian, S. Lou, X. Jian, J. Y. Li and Z. W. Zhou, Defect-enhanced electromagnetic wave absorption property of hierarchical graphite capsules@helical carbon nanotube hybrid nanocomposites, ACS Appl. Mater. Interfaces, 2021, 13, 28710–28720 CrossRef CAS PubMed.
- S. C. Zhao, L. L. Yan, X. D. Tian, Y. Q. Liu, C. Q. Chen, Y. Q. Li, J. K. Zhang, Y. Song and Y. Qin, Flexible design of gradient multilayer nanofilms coated on carbon nanofibers by atomic layer deposition for enhanced microwave absorption performance, Nano Res., 2018, 11, 530–541 CrossRef CAS.
- Z. J. Liao, M. L. Ma, Y. X. Bi, Z. Y. Tong, K. L. Chung, Z. J. Li, Y. Ma, B. L. Gao, Z. K. Cao, R. R. Sun and X. Zhong, Mos2 decorated on one-dimensional MgFe2O4/MgO/c composites for high-performance microwave absorption, J. Colloid Interface Sci., 2022, 606, 709–718 CrossRef CAS PubMed.
- Y. Fei, M. Liang, T. Zhou, Y. Chen and H. W. Zou, Unique carbon nanofiber@ Co/C aerogel derived bacterial cellulose embedded zeolitic imidazolate frameworks for high-performance electromagnetic interference shielding, Carbon, 2020, 167, 575–584 CrossRef CAS.
- W. L. Song, L. Z. Fan, Z. L. Hou, K. L. Zhang, Y. B. Ma and M. S. Cao, A wearable microwave absorption cloth, J. Mater. Chem. C, 2017, 5, 2432–2441 RSC.
- Y. Wang, X. Zhang, X. J. Zhang, T. Zhou, Z. J. Cui and K. Zhang, A novel terahertz metasurface based on a single-walled carbon nanotube film for sensing application, J. Mater. Chem. A, 2022, 1, 1780–1787 RSC.
- S. G. Kim, T. V. Tran and J. S. Lee, Iron oxide-immobilized porous carbon nanofiber-based radio frequency identification (RFID) tag sensor for detecting hydrogen sulfide, J. Ind. Eng. Chem., 2022, 112, 423–429 CrossRef CAS.
- J. C. Shu, X. Y. Huang and M. S. Cao, Assembling 3D flower-like Co3O4-mwcnt architecture for optimizing low-frequency microwave absorption, Carbon, 2021, 174, 638–646 CrossRef CAS.
- Z. Z. Guo, P. G. Ren, J. Wang, J. H. Tang, F. D. Zhang, Z. Zong, Z. Y. Chen, Y. L. Jin and F. Ren, Multifunctional sandwich-structured magnetic-electric composite films with Joule heating capacities toward absorption-dominant electromagnetic interference shielding, Composites, Part B, 2022, 236, 109836 CrossRef CAS.
- M. S. Cao, X. X. Wang, W. Q. Cao, X. Y. Fang, B. Wen and J. Yuan, Thermally driven transport and relaxation switching self-powered electromagnetic energy conversion, Small, 2018, 14, 1800987 CrossRef.
- M. S. Cao, J. C. Shu, B. Wen, X. X. Wang and W. Q. Cao, Genetic dielectric genes inside 2D carbon-based materials with tunable electromagnetic function at elevated temperature, Small Struct., 2021, 2, 2100104 CrossRef CAS.
- X. X. Wang, M. Zhang, J. C. Shu, B. Wen, W. Q. Cao and M. S. Cao, Thermally-tailoring dielectric “genes” in graphene-based heterostructure to manipulate electromagnetic response, Carbon, 2021, 184, 136–145 CrossRef CAS.
- R. Qin, A. P. Ou, Y. L. Li, H. Deng, Y. Liu and X. Y. Liu, Noticeably enhanced microwave absorption performance via constructing molecular-level interpenetrating carbon network heterostructure, Carbon, 2021, 183, 858–871 CrossRef CAS.
- J. Q. Tao, L. L. Xu, H. S. Jin, Y. S. Gu, J. T. Zhou, Z. J. Yao, X. W. Tao, P. Chen, D. H. Wang, Z. Li and H. J. Wu, Selective coding dielectric genes based on proton tailoring to improve microwave absorption of MOFs, Adv. Powder Mater., 2023, 2, 100091 CrossRef.
- J. J. Xue, T. Wu, Y. Q. Dai and Y. N. Xia, Electrospinning and electrospun nanofibers: methods, materials, and applications, Chem. Rev., 2019, 119, 5298–5415 CrossRef CAS PubMed.
-
A. Formhals, Process and apparatus for preparing artificial threads, US Pat., 1975504, 1934 Search PubMed.
- G. Taylor, electrically driven jets, Proc. R. Soc. London, Ser. A, 1969, 313, 453–475 Search PubMed.
- X. L. Yang, J. W. Wang, H. T. Guo, L. Liu, W. H. Xu and G. G. Duan, Structural design toward functional materials by electrospinning: a review, E-Polymers, 2020, 20, 682–712 CrossRef CAS.
- W. P. Cai, Y. Y. Zhang, Y. T. Jia and J. H. Yan, Flexible heteroatom-doped porous carbon nanofiber cages for electrode scaffolds, Carbon Energy, 2020, 2, 472–481 CrossRef CAS.
- Q. Zheng, M. J. Yu, W. Wang, Y. J. Wang, Z. Zhang, H. P. Zhou, Y. Y. Dai, C. G. Wang and Y. Xu, Enhanced microwave absorption performance of Fe/C nanofibers by adjusting the magnetic particle size using different electrospinning solvents, Ceram. Int., 2020, 46, 28603–28612 CrossRef CAS.
- A. Koski, K. Yim and S. Shivkumar, Effect of molecular weight on fibrous PVA produced by electrospinning, Mater. Lett., 2004, 58, 493–497 CrossRef CAS.
- D. S. Katti, K. W. Robinson, F. K. Ko and C. T. Laurencin, Bioresorbable nanofiber-based systems for wound healing and drug delivery: optimization of fabrication parameters, J. Biomed. Mater. Res., 2004, 70B, 286–296 CrossRef CAS PubMed.
- R. D. Guo, D. Su, F. Chen, Y. Z. Cheng, X. Wang, R. Z. Gong and H. Luo, Hollow beaded Fe3C/N-doped carbon fibers toward broadband microwave absorption, ACS Appl. Mater. Interfaces, 2022, 14, 3084–3094 CrossRef CAS PubMed.
- Z. F. Mao, H. W. Wang, D. L. Chao, R. Wang, B. B. He, Y. S. Gong and H. J. Fan, Al2O3-assisted confinement synthesis of oxide/carbon hollow composite nanofibers and application in metal-ion capacitors, Small, 2020, 16, 2001950 CrossRef CAS PubMed.
- P. X. Hou, F. Zhang, L. L. Zhang, C. Liu and H. M. Cheng, Synthesis of carbon nanotubes by floating catalyst chemical vapor deposition and their applications, Adv. Funct. Mater., 2022, 32, 2108541 CrossRef CAS.
- F. Yang, M. Wang, D. Q. Zhang, J. Yang, M. Zheng and Y. Li, Chirality pure carbon nanotubes: growth, sorting, and characterization, Chem. Rev., 2020, 120, 2693–2758 CrossRef CAS PubMed.
- Z. Q. Yao, C. G. Wang, J. J. Qin, S. S. Su, Y. X. Wang, Q. F. Wang, M. J. Yu and H. Z. Wei, Interfacial improvement of carbon fiber/epoxy composites using one-step method for grafting carbon nanotubes on the fibers at ultra-low temperatures, Carbon, 2020, 164, 133–142 CrossRef CAS.
- F. Yang, X. Wang, M. H. Li, X. Y. Liu, X. L. Zhao, D. Q. Zhang, Y. Zhang, J. Yang and Y. Li, Templated synthesis of single-walled carbon nanotubes with specific structure, Acc. Chem. Res., 2016, 49, 606–615 CrossRef CAS PubMed.
- N. J. Tang, J. F. Wen, Y. Zhang, F. X. Liu, K. J. Lin and Y. W. Du, Helical carbon nanotubes: catalytic particle size-dependent growth and magnetic properties, ACS Nano, 2010, 4, 241–250 CrossRef CAS PubMed.
- H. Sun, R. C. Che, X. You, Y. S. Jiang, Z. B. Yang, J. Deng, L. B. Qiu and H. S. Peng, Cross-stacking aligned carbon-nanotube films to tune microwave absorption frequencies and increase absorption intensities, Adv. Mater., 2014, 26, 8120–8125 CrossRef CAS PubMed.
- A. Elhassan, I. Abdalla, J. Y. Yu, Z. L. Li and B. Ding, Microwave-assisted fabrication of sea cucumber-like hollow structured composite for high-performance electromagnetic wave absorption, Chem. Eng. J., 2020, 392, 123646 CrossRef CAS.
- Y. Z. Jiao, J. J. Li, A. M. Xie, F. Wu, K. Zhang, W. Dong and X. F. Zhu, Confined polymerization strategy to construct polypyrrole/zeolitic imidazolate frameworks (PPy/ZIFs) nanocomposites for tunable electrical conductivity and excellent electromagnetic absorption, Compos. Sci. Technol., 2019, 174, 232–240 CrossRef CAS.
- M. X. Sun, C. Xu, J. L. Li, L. Q. Xing, T. Zhou, F. Wu, Y. F. Shang and A. M. Xie, Protonic doping brings tuneable dielectric and electromagnetic attenuated properties for polypyrrole nanofibers, Chem. Eng. J., 2020, 381, 122615 CrossRef.
- A. M. Xie, F. Wu, W. C. Jiang, K. Zhang, M. X. Sun and M. Y. Wang, Chiral induced synthesis of helical polypyrrole (PPy) nano-structures: a lightweight and high-performance material against electromagnetic pollution, J. Mater. Chem. C, 2017, 5, 2175–2181 RSC.
- X. Zhang, Y. Huang and P. B. Liu, Enhanced electromagnetic wave absorption properties of poly(3,4-ethylenedioxythiophene) nanofiber-decorated graphene sheets by non-covalent interactions, Nano-Micro Lett., 2016, 8, 131–136 CrossRef PubMed.
- A. Hazarika, B. K. Deka, K. Kong, D. Y. Kim, Y. W. Nam, J. H. Choi, C. G. Kim, Y. B. Park and H. W. Park, Microwave absorption and mechanical performance of α-MnO2 nanostructures grown on woven kevlar fiber/reduced graphene oxide-polyaniline nanofiber array-reinforced polyester resin composites, Composites, Part B, 2018, 140, 123–132 CrossRef CAS.
- N. Joseph, J. Varghese and M. T. Sebastian, A facile formulation and excellent electromagnetic absorption of room temperature curable polyaniline nanofiber based inks, J. Mater. Chem. C, 2016, 4, 918–999 RSC.
- W. J. Wang, S. P. Gumfekar, Q. J. Jiao and B. X. Zhao, Ferrite-grafted polyaniline nanofibers as electromagnetic shielding materials, J. Mater. Chem. C, 2013, 1, 2851–2859 RSC.
- R. Castaldo, R. Avolio, M. Cocca, G. Gentile, M. E. Errico, M. Avella, C. Carfagna and V. Ambrogi, A versatile synthetic approach toward hyper-cross-linked styrene-based polymers and nanocomposites, Macromolecules, 2017, 50, 4132–4143 CrossRef CAS.
- M. Seo, S. Kim, J. Oh, S. Kim and M. A. Hillmyer, Hierarchically porous polymers from hyper-cross-linked block polymer precursors, J. Am. Chem. Soc., 2015, 137, 600–603 CrossRef CAS PubMed.
- Z. A. Qiao, S. H. Chai, K. Nelson, Z. H. Bi, J. H. Chen, S. M. Mahurin, X. Zhu and S. Dai, Polymeric molecular sieve membranes via in situ cross-linking of non-porous polymer membrane templates, Nat. Commun., 2014, 5, 3705 CrossRef PubMed.
- J. Q. Wang, Z. T. Yang, A. Mudasir, Z. H. Peng, Z. Q. Yu and B. L. Zhang, A novel synthetic method for tubular nanofibers, Polym. Chem., 2019, 10, 4239 RSC.
- J. Q. Wang, F. Wu, Y. H. Cui, J. J. Chen, A. B. Zhang, Q. Y. Zhang and B. L. Zhang, Facile synthesis of tubular magnetic carbon nanofibers by hypercrosslinked polymer design for microwave adsorption, J. Am. Ceram. Soc., 2020, 103, 5706–5720 CrossRef CAS.
- S. Kang, S. Y. Qiao, Y. T. Cao, Z. M. Hu, J. R. Yu and Y. Wang, High-efficiency microwave attenuation of magnetic carbon nanoparticle-decorated tubular carbon nanofibers composites at an ultralow filling content, Adv. Electron. Mater., 2021, 7, 2100121 CrossRef CAS.
- J. Q. Wang, Y. Huyan, Z. T. Yang, A. B. Zhang, Q. Y. Zhang and B. L. Zhang, Tubular carbon nanofibers: synthesis, characterization and applications in microwave absorption, Carbon, 2019, 152, 255–266 CrossRef CAS.
- J. Q. Wang, F. Wu, Z. T. Yang, T. Shah, A. B. Zhang, Q. Y. Zhang and B. L. Zhang, Preparation of CTCNFs/Co9S8 hybrid nanofibers with enhanced microwave absorption performance, Nanotechnology, 2020, 31, 225605 CrossRef CAS PubMed.
- S. Kang, S. Y. Qiao, Y. T. Cao, Z. M. Hu, J. R. Yu, Y. Wang and J. Zhu, Hyper-cross-linked polymers-derived porous tubular carbon nanofibers@TiO2 toward a wide-band and lightweight microwave absorbent at a low loading content, ACS Appl. Mater. Interfaces, 2020, 12, 46455–46465 CrossRef CAS PubMed.
- X. L. Wang, X. Huang, Z. R. Chen, X. P. Liao, C. Liu and B. Shi, Ferromagnetic hierarchical carbon nanofiber bundles derived from natural collagen fibers: truly lightweight and high-performance microwave absorption materials, J. Mater. Chem. C, 2015, 3, 10146–10153 RSC.
- L. F. Chen, Z. H. Huang, H. W. Liang, Q. F. Guan and S. H. Yu, Bacterial-cellulose-derived carbon nanofiber@MnO2 and nitrogen-doped carbon nanofiber electrode materials: an asymmetric supercapacitor with high energy and power density, Adv. Mater., 2013, 25, 4746–4752 CrossRef CAS PubMed.
- S. Padron, A. Fuentes, D. Caruntu and K. Lozano, Experimental study of nanofiber production through forcespinning, J. Appl. Phys., 2013, 113, 24318 CrossRef.
- K. Sarkar, C. Gomez, S. Zambrano, M. Ramirez, E. de Hoyos, H. Vasquez and K. Lozano, Electrospinning to forcespinningtm, Mater. Today, 2010, 13, 12–14 CrossRef CAS.
- L. D. Cremar, J. Acosta-Martinez, A. Villarreal, A. Salinas and K. Lozano, Mechanical and electrical characterization of carbon nanofibers produced from water soluble precursors, Mater. Today Commun., 2016, 7, 134–139 CrossRef CAS.
- J. G. Ju, W. M. Kang, N. P. Deng, L. Li, Y. X. Zhao, X. M. Ma, L. L. Fan and B. W. Cheng, Preparation and characterization of pva-based carbon nanofibers with honeycomb-like porous structure via electro-blown spinning method, Microporous Mesoporous Mater., 2017, 239, 416–425 CrossRef CAS.
- Z. W. Li, Z. W. Cui, L. H. Zhao, N. Hussain, Y. Z. Zhao, C. Yang, X. Y. Jiang, L. Li, J. N. Song, B. P. Zhang, Z. K. Cheng and H. Wu, High-throughput production of kilogram-scale nanofibers by karman vortex solution blow spinning, Sci. Adv., 2022, 8, eabn3690 CrossRef CAS PubMed.
- K. Abe and M. Utsumi, Wet spinning of cellulose nanofibers via gelation by alkaline treatment, Cellulose, 2020, 27, 10441–10446 CrossRef CAS.
- J. J. Yao, S. Y. Chen, Y. Chen, B. X. Wang, Q. B. Pei and H. P. Wang, Macrofibers with high mechanical performance based on aligned bacterial cellulose nanofibers, ACS Appl. Mater. Interfaces, 2017, 9, 20330–20339 CrossRef CAS PubMed.
- A. P. Wang, X. Zhang, F. Chen and Q. Fu, Aramid nanofiber framework supporting graphene nanoplate via wet-spinning for a high-performance filament, Carbon, 2021, 179, 655–665 CrossRef CAS.
- L. Francis, N. Ghaffour, A. S. Alsaadi, S. P. Nunes and G. L. Amy, PVDF hollow fiber and nanofiber membranes for fresh water reclamation using membrane distillation, J. Mater. Sci., 2014, 49, 2045–2053 CrossRef CAS.
- X. W. Shi, Y. L. Hu, F. Y. Fu, J. P. Zhou, Y. X. Wang, L. Y. Chen, H. M. Zhang, J. Li, X. H. Wang and L. N. Zhang, Construction of PANI–cellulose composite fibers with good antistatic properties, J. Mater. Chem. A, 2014, 2, 7669–7673 RSC.
- G. Li, T. S. Xie, S. L. Yang, J. H. Jin and J. M. Jiang, Microwave absorption enhancement of porous carbon fibers compared with carbon nanofibers, J. Phys. Chem. C, 2012, 116, 9196–9201 CrossRef CAS.
- M. S. Cao, W. L. Song, Z. L. Hou, B. Wen and J. Yuan, The effects of temperature and frequency on the dielectric properties, electromagnetic interference shielding and microwave-absorption of short carbon fiber/silica composites, Carbon, 2010, 48, 788–796 CrossRef CAS.
- W. L. Song, M. S. Cao, Z. L. Hou, X. Y. Fang, X. L. Shi and J. Yuan, High dielectric loss and its monotonic dependence of conducting-dominated multiwalled carbon nanotubes/silica nanocomposite on temperature
ranging from 373 to 873 K in x-band, Appl. Phys. Lett., 2009, 94, 233110 CrossRef.
- B. Wen, M. S. Cao, Z. L. Hou, W. L. Song, L. Zhang, M. M. Lu, H. B. Jin, X. Y. Fang, W. Z. Wang and J. Yuan, Temperature dependent microwave attenuation behavior for carbon-nanotube/silica composites, Carbon, 2013, 65, 124–139 CrossRef CAS.
- M. M. Lu, W. Q. Cao, H. L. Shi, X. Y. Fang, J. Yang, Z. L. Hou, H. B. Jin, W. Z. Wang, J. Yuan and M. S. Cao, Multi-wall carbon nanotubes decorated with ZnO nanocrystals: mild solution-process synthesis and highly efficient microwave absorption properties at elevated temperature, J. Mater. Chem. A, 2014, 2, 10540–10547 RSC.
- M. S. Cao, J. Yang, W. L. Song, D. Q. Zhang, B. Wen, H. B. Jin, Z. L. Hou and J. Yuan, Ferroferric oxide/multiwalled carbon nanotube vs polyaniline/ferroferric oxide/multiwalled carbon nanotube multiheterostructures for highly effective microwave absorption, ACS Appl. Mater. Interfaces, 2012, 4, 6949–6956 CrossRef CAS PubMed.
- M. S. Cao, X. X. Wang, M. Zhang, J. C. Shu, W. Q. Cao, H. J. Yang, X. Y. Fang and J. Yuan, Electromagnetic response and energy conversion for functions and devices in low-dimensional materials, Adv. Funct. Mater., 2019, 29, 1807398 CrossRef.
- C. Han, M. Zhang, W. Q. Cao and M. S. Cao, Electrospinning and in situ hierarchical thermal treatment to tailor C-NiCo2O4 nanofibers for tunable microwave absorption, Carbon, 2021, 171, 953–962 CrossRef CAS.
- Z. W. Liu, R. C. Che, Y. Wei, Y. P. Liu, A. A. Elzatahry, D. A. Dahyan and D. Y. Zhao, Broadening microwave absorption via a multi-domain structure, APL Mater., 2017, 5, 46104 CrossRef.
- K. Pei, S. S. Liu, E. Z. Zhang, X. B. Zhao, L. T. Yang, L. F. Ai, Z. H. Li, F. X. Xiu and R. C. Che, Anomalous spin behavior in Fe3GeTe2 driven by current pulses, ACS Nano, 2020, 14, 9512–9520 CrossRef CAS PubMed.
- T. Y. Huang, M. Yang, J. Y. Wang, S. J. Zhang, J. Du and D. Wang, Preparation and microwave absorbing property of NiFe2O4/c composites with hollow multishelled structure, Chem. J. Chin. Univ., 2023, 44, 290–299 Search PubMed.
- S. A. Hashemi, A. Ghaffarkhah, E. Hosseini, S. Bahrani, P. Najmi, N. Omidifar, S. M. Mousavi, M. Amini, M. Ghaedi, S. Ramakrishna and M. Arjmand, Recent progress on hybrid fibrous electromagnetic shields: key protectors of living species against electromagnetic radiation, Matter, 2022, 5, 3807–3868 CrossRef CAS.
- Z. S. Ju, P. Li, X. N. Zhao, J. G. Ma, H. Y. Xu and Y. C. Liu, Flexible TiN/Co@carbon nanofiber mats for high-performance electromagnetic interference shielding and Joule heating applications, Carbon, 2022, 196, 612–620 CrossRef CAS.
- Y. Li, X. F. Liu, X. Y. Nie, W. W. Yang, Y. D. Wang, R. H. Yu and J. L. Shui, Multifunctional organic–inorganic hybrid aerogel for self-cleaning, heat-insulating, and highly efficient microwave absorbing material, Adv. Funct. Mater., 2019, 29, 1807624 CrossRef.
- M. Ma, Q. Zheng, Y. H. Zhu, L. Li and M. S. Cao, Confinedly implanting Fe3O4 nanoclusters on MoS2 nanosheets to tailor electromagnetic properties for excellent multi-bands microwave absorption, J. Materiomics, 2022, 8, 577–585 CrossRef.
- L. H. Yao, W. Q. Cao, J. G. Zhao, Q. Zheng, Y. C. Wang, S. Jiang, Q. L. Pan, J. Song, Y. Q. Zhu and M. S. Cao, Regulating bifunctional flower-like NiFe2O4/graphene for green EMI shielding and lithium ion storage, J. Mater. Sci. Technol., 2022, 127, 48–60 CrossRef.
- G. Z. Wang, Z. Gao, S. W. Tang, C. Q. Chen, F. F. Duan, S. C. Zhao, S. W. Lin, Y. H. Feng, L. Zhou and Y. Qin, Microwave absorption properties of carbon nanocoils coated with highly controlled magnetic materials by atomic layer deposition, ACS Nano, 2012, 6, 11009–11017 CrossRef CAS PubMed.
- X. F. Xu, S. H. Shi, G. P. Wan, C. C. Hao, Z. Y. He and G. Z. Wang, Uniformly coating MnOx nanoflakes onto carbon nanofibers as lightweight and wideband microwave absorbers with frequency-selective absorption, Mater. Des., 2019, 183, 108167 CrossRef CAS.
- Y. Z. Wei, D. C. Zhao, J. W. Wan and D. Wang, Electron-orbital-lattice interactions in hollow multishelled structures, Trends Chem., 2022, 4, 1021–1033 CrossRef CAS.
- J. Q. Wang, Y. H. Cui, F. Wu, T. Shah, M. Ahmad, A. B. Zhang, Q. Y. Zhang and B. L. Zhang, Core–shell structured Fe/Fe3O4@TCNFs@TiO2 magnetic hybrid nanofibers: preparation and electromagnetic parameters regulation for enhanced microwave absorption, Carbon, 2020, 165, 275–285 CrossRef CAS.
- Y. J. Wan, X. Y. Wang, X. M. Li, S. Y. Liao, Z. Q. Lin, Y. G. Hu, T. Zhao, X. L. Zeng, C. H. Li, S. H. Yu, P. L. Zhu, R. Sun and C. P. Wong, Ultrathin densified carbon nanotube film with “metal-like” conductivity, superior mechanical strength, and ultrahigh electromagnetic interference shielding effectiveness, ACS Nano, 2020, 14, 14134–14145 CrossRef CAS PubMed.
- J. L. Chen, D. Yi, X. C. Jia, G. Q. Wang, Z. P. Sun, L. H. Zhang, Y. F. Liu, B. Shen and W. G. Zheng, Biomass-based aligned carbon networks with double-layer construction for tunable electromagnetic shielding with ultra-low reflectivity, J. Mater. Sci. Technol., 2022, 103, 98–104 CrossRef.
- B. Du, D. Y. Zhang, J. J. Qian, M. Cai, C. He, P. Zhou and A. Z. Shui, Multifunctional carbon nanofiber-SiC nanowire aerogel films with superior microwave absorbing performance, Adv. Compos. Hybrid Mater., 2021, 4, 1281–1291 CrossRef CAS.
- H. Song, H. Jeon, D. Im, N. Cakmakci, K. Y. Shin and Y. Jeong, Free-standing carbon nanotube film for high efficiency monopole antenna, Carbon, 2022, 187, 22–28 CrossRef CAS.
- F. Shahzad, M. Alhabeb, C. B. Hatter, B. Anasori, S. M. Hong, C. M. Koo and Y. Gogotsi, Electromagnetic interference shielding with 2D transition metal carbides (MXenes), Science, 2016, 353, 1137–1140 CrossRef CAS PubMed.
- Y. Z. Li, J. Y. Wu, P. Z. Yang, L. Z. Song, J. Wang, Z. G. Xing and J. W. Zhao, Multi-degree-of-freedom robots powered and controlled by microwaves, Adv. Sci., 2022, 9, 2203305 CrossRef CAS PubMed.
- M. K. Han, Y. Q. Liu, R. Rakhmanov, C. Israel, M. A. S. Tajin, G. Friedman, V. Volman, A. Hoorfar, K. R. Dandekar and Y. Gogotsi, Solution-processed Ti3C2Tx MXene antennas for radio-frequency communication, Adv. Mater., 2021, 33, 2003225 CrossRef CAS PubMed.
- S. Lee, I. Jo, S. Kang, B. Jang, J. Moon, J. B. Park, S. Lee, S. Rho, Y. Kim and B. H. Hong, Smart contact lenses with graphene coating for electromagnetic interference shielding and dehydration
protection, ACS Nano, 2017, 11, 5318–5324 CrossRef CAS PubMed.
- Y. Sun, Y. J. Wang, H. J. Ma, Y. Zhou, H. N. Xing, W. Feng, J. Feng, Z. H. Shi, Y. Zong, X. H. Li and X. L. Zheng, Fe3C nanocrystals encapsulated in n-doped carbon nanofibers as high-efficient microwave absorbers with superior oxidation/corrosion resistance, Carbon, 2021, 178, 515–527 CrossRef CAS.
- T. Yu, J. Qiu, J. Liao, X. Wang, W. Chen, Y. Cheng, W. Wang and Y. Song, Topological transformation strategy for layered double hydroxide@carbon nanofibers as highly efficient electromagnetic wave absorber, J. Alloys Compd., 2021, 867, 159046 CrossRef CAS.
- J. Qiao, X. Zhang, C. Liu, L. F. Lyu, Z. Wang, L. L. Wu, W. Liu, F. L. Wang and J. R. Liu, Facile fabrication of Ni embedded TiO2/C core–shell ternary nanofibers with multicomponent functional synergy for efficient electromagnetic wave absorption, Composites, Part B, 2020, 200, 108343 CrossRef CAS.
- P. Z. Liu, T. D. Gao, W. J. He and P. B. Liu, Electrospinning of hierarchical carbon fibers with multi-dimensional magnetic configurations toward prominent microwave absorption, Carbon, 2023, 202, 244–253 CrossRef CAS.
- W. Zhou, Y. Li, L. Long, H. Luo and Y. C. Wang, High-temperature electromagnetic wave absorption properties of Cf/SiCNFs/Si3N4 composites, J. Am. Ceram. Soc., 2020, 103, 6822–6832 CrossRef CAS.
- D. T. Kuang, L. Z. Hou, S. L. Wang, H. Luo, L. W. Deng, J. L. Mead, H. Huang and M. Song, Large-scale synthesis and outstanding microwave absorption properties of carbon nanotubes coated by extremely small FeCo–C core–shell nanoparticles, Carbon, 2019, 153, 52–61 CrossRef CAS.
- H. Tang, X. Jian, B. Wu, S. Y. Liu, Z. C. Jiang, X. N. Chen, W. Q. Lv, W. D. He, W. Tian, Y. F. Wei, Y. Q. Gao, T. Chen and G. Li, Fe3C/helical carbon nanotube hybrid: facile synthesis and spin-induced enhancement in microwave-absorbing properties, Composites, Part B, 2016, 107, 51–58 CrossRef CAS.
- C. J. Wang, Y. X. Wang, H. T. Jiang, H. X. Tan and D. M. Liu, Continuous in situ growth of carbon nanotubes on carbon fibers at various temperatures for efficient electromagnetic wave absorption, Carbon, 2022, 200, 94–107 CrossRef CAS.
- Y. Yue, Y. X. Wang, X. D. Xu, C. J. Wang, Z. Q. Yao and D. M. Liu,
In situ growth of bamboo-shaped carbon nanotubes and helical carbon nanofibers on Ti3C2Tx MXene at ultra-low temperature for enhanced electromagnetic wave absorption properties, Ceram. Int., 2022, 48, 6338–6346 CrossRef CAS.
- X. Y. Zhao, D. M. Zhu, J. Z. Wu, R. D. Zhang, X. K. Lu, B. B. Fan, Y. Li and H. J. Wu, Environmentally friendly a multifunctional cellulose-based carbon foam for superior electromagnetic wave absorption performance, Compos. Commun., 2022, 35, 101320 CrossRef.
- H. T. Guo, M. H. Zheng, X. F. Ma, R. C. Cao, K. M. Liu, W. S. Yang, S. J. Jian, S. H. Jiang and G. G. Duan, Electrospun TaC/Fe3C–Fe carbon composite fabrics for high efficiency of electromagnetic interference shielding, Compos. Commun., 2022, 31, 101130 CrossRef.
- Z. W. Li, Z. J. Lin, M. S. Han, Y. B. Mu, P. P. Yu, Y. L. Zhang and J. Yu, Flexible electrospun carbon nanofibers/silicone composite films for electromagnetic interference shielding, electrothermal and photothermal applications, Chem. Eng. J., 2021, 420, 129826 CrossRef CAS.
- D. G. Gao, S. H. Guo, Y. Y. Zhou, B. Lyu, X. J. Li, P. Zhao and J. Z. Ma, Absorption-dominant, low-reflection multifunctional electromagnetic shielding material derived from hydrolysate of waste leather scraps, ACS Appl. Mater. Interfaces, 2022, 14, 38077–38089 CrossRef CAS PubMed.
- B. D. S. Deeraj, G. George, N. R. Dhineshbabu, S. Bose and K. Joseph, Electrospun ZrO2@carbon nanofiber mats and their epoxy composites as effective EMI shields in Ku band, Mater. Res. Bull., 2021, 144, 111477 CrossRef CAS.
- O. Pitkänen, J. Tolvanen, I. Szenti, Á. Kukovecz, J. Hannu, H. Jantunen and K. Kordas, Lightweight hierarchical carbon nanocomposites with highly efficient and tunable electromagnetic interference shielding properties, ACS Appl. Mater. Interfaces, 2019, 11, 19331–19338 CrossRef PubMed.
- M. Praveen, G. S. Karthikeya, R. H. Krishna, G. M. Mamatha, C. Manjunatha, A. Khosla and B. M. Nagabhushana, The role of magnetic nano CoFe2O4 and conductive mwcnt/graphene in LDPE-based composites for electromagnetic interference shielding in X-band, Diamond Relat. Mater., 2022, 130, 109501 CrossRef CAS.
- Q. Q. Men, S. Wang, Z. K. Yan, B. Zhao, L. Guan, G. Y. Chen, X. Q. Guo, R. Zhang and R. C. Che, Iron-encapsulated CNTs on carbon fiber with high-performance EMI shielding and electrocatalytic activity, Adv. Compos. Hybrid Mater., 2022, 5, 2429–2439 CrossRef CAS.
- B. Huang, J. Yue, B. Fan, X. Tang, Y. Liu and X. Huang, Constructing hierarchical structure via in situ growth of CNT in SiO2-coated carbon foam for high-performance EMI shielding application, Compos. Sci. Technol., 2022, 222, 109372 CrossRef CAS.
- Y. Y. Yu, Z. Chao, Q. Gong, C. W. Li, H. L. Fu, F. Lei, D. M. Hu and L. X. Zheng, Tailoring hierarchical carbon nanotube cellular structure for electromagnetic interference shielding in extreme conditions, Mater. Des., 2021, 206, 109783 CrossRef CAS.
- H. W. Wei, W. H. Zheng, Z. X. Jiang and Y. D. Huang, CNT coatings grown on the outer and inner surfaces of magnetic hollow carbon fibers with enhanced electromagnetic interference shielding performance, J. Mater. Chem. C, 2019, 7, 14375–14383 RSC.
- Z. Zong, P. G. Ren, Z. Z. Guo, J. Wang, Z. Y. Chen, Y. L. Jin and F. Ren, Three-dimensional macroporous hybrid carbon aerogel with heterogeneous structure derived from MXene/cellulose aerogel for absorption-dominant electromagnetic interference shielding and excellent thermal insulation performance, J. Colloid Interface Sci., 2022, 619, 96–105 CrossRef CAS PubMed.
- Z. H. Zhou, Y. Liang, H. D. Huang, L. Li, B. Yang, M. Z. Li, D. X. Yan, J. Lei and Z. M. Li, Structuring dense three-dimensional sheet-like skeleton networks in biomass-derived carbon aerogels for efficient electromagnetic interference shielding, Carbon, 2019, 152, 316–324 CrossRef CAS.
|
This journal is © the Partner Organisations 2023 |
Click here to see how this site uses Cookies. View our privacy policy here.