DOI:
10.1039/D2QM01147F
(Review Article)
Mater. Chem. Front., 2023,
7, 789-805
The nonhalides in perovskite solar cells
Received
8th November 2022
, Accepted 30th December 2022
First published on 4th January 2023
Abstract
Recently, halide perovskite solar cells (PSCs) have accomplished the most remarkable progress in emerging photovoltaic technology. However, their poor stability hinders the route to commercialization. Notably, the high chemical activity of halides such as I− and Br− and the formed halide-related vacancy defects lead to the instability of PSCs. In spite of the importance of X-site anions, more and more works have been devoted to the polyatomic pseudohalides and superhalogens in perovskites. In this context, this review will focus on the basic understanding of the structural and photoelectric properties of pseudohalides and superhalogens. We comprehensively summarize the current research progress of SCN−, HCCO−, Ac−, BF4−, BH4−, and PF6− anions to realize high structurally stable perovskites and related solar cells. Based on these observations, some perspectives on future efforts toward achieving highly stable PSCs are proposed and discussed.
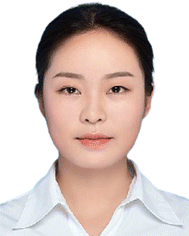
Shurong Wang
| Shurong Wang is currently pursuing her PhD under the supervision of Prof. Feng Hao at the School of Materials and Energy, University of Electronic Science and Technology of China. She received her BS in Applied Chemistry (2017) and ME in Environmental Engineering (2020) from Nanjing University of Information Science and Technology. Her current research focuses on component engineering and defect engineering for lead-free perovskite solar cells. |
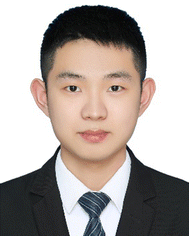
Cheng Wu
| Cheng Wu received his Master's degree from Jiangsu University in 2021. Now he pursuing his PhD supervised by Prof. Feng Hao at the University of Electronic Science and Technology of China. His current research interest mainly focuses on developing highly efficient organic interlayer for lead-free perovskite solar cells. |
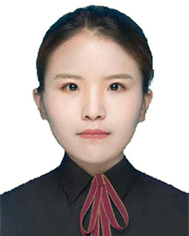
Huanhuan Yao
| Huanhuan Yao is currently pursuing her PhD under the supervision of Prof. Feng Hao at the School of Materials and Energy, University of Electronic Science and Technology of China. She received her BS in physics (2019) from Northwest Normal University and ME in materials science and engineering (2022) from Lanzhou University. Her current research focuses on lead-free perovskite solar cells. |
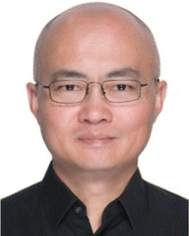
Liming Ding
| Liming Ding achieved his PhD degree from the University of Science and Technology of China. He started his research on OSCs and PLEDs in Olle Inganäs Lab in 1998. Then, he worked with Frank Karasz and Tom Russell at PSE, UMASS Amherst. He joined Konarka as a Senior Scientist in 2008. In 2010, he joined the National Center for Nanoscience and Technology as a Full Professor. His research interests include perovskite solar cells, organic solar cells and photodetectors. |
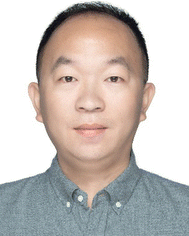
Feng Hao
| Feng Hao received his PhD from Tsinghua University in 2012. Then he moved to Northwestern University as a postdoc in the Department of Chemistry. He has been a full professor at the School of Materials and Energy, University of Electronic Science and Technology of China since 2017. His research focuses on perovskite solar cells and photonic materials. |
1. Introduction
Perovskite solar cells (PSCs) have driven much interest in the development of photovoltaic devices since they first emerged in 2009.1,2 The hybrid organic–inorganic halide perovskites act as a classical solar absorber material. Their great merits such as high carrier mobility, high absorption coefficient, low carrier recombination rate, low exciton binding energy, high defect tolerance, and tunable band-gap properties enabled an outstanding performance within a decade.3–6 The certified power conversion efficiency (PCE) has been pushed to 25.7% with superior long-term operational stability.7
In general, the halide perovskite has a ABX3 scaffold, where the A site denotes a monovalent cation (such as CH3NH3+ (MA+), CH(NH2)2+ (FA+), and Cs+), the B site denotes a divalent metal cation (such as Pb2+ and Sn2+), and the X site denotes a monovalent anion (such as I−, Cl−, and Br−).8 The corner-shared [BX6]4− octahedra form a cuboctahedral cavity to accommodate the A site that satisfies the three-dimensional (3D) perovskite structure. The electronic and optical properties of the halide perovskite show a great relationship to the composition and structural variations. The exploration of the possible range of A-site cations has been widely investigated. The alternative A-site cations to yield the 3D perovskite lattice only are Cs+, FA+, and MA+. Other large organic spacer cations (such as PEA+ (C6H5(CH2)2NH3+), BA+ (CH3(CH2)3NH3+), or EDA2+ ((CH2)2(NH3)22+)) could be incorporated into perovskites to form a low-dimensional structure like the RP (Ruddlesden–Popper) phase with a formula of
or the DJ (Dion–Jacobson) phase with a A′An−1BnX3n+1 framework. However, a preferred vertical orientation of a low-dimensional perovskite is hard to achieve owing to its different quantum well thickness with different n values. The small n-value perovskite is more likely to orient parallel to the substrate, and the high n-value perovskite tends to achieve a vertical orientation.9
In addition, the Pb2+ of the B-site cation has high toxicity, which is very concerning in terms of environmental and human health concerns. Other metal cations with similar electron configurations in the IVA/VA group, such as Sn2+, Ge2+, Sb3+, Bi3+, Ag+, Cu+, and Au+, still show poor performance.10,11 Meanwhile, I−, Cl−, and Br− are the most widely used X-site anions, which react with BX2 to form the ABX3 perovskite materials. However, the halide anion of I− shows high chemical activity and the corresponding I− vacancy defects could form easily. The existing I− vacancy defects have a negative impact on the device stability owing to the unwanted increase in ion conductivity.12 Partial substitution of I− by Br− could tune the band gap and improve the structural stability. As for Cl−, the Cl− incorporation could affect the nucleation and crystallization of the perovskite film, although it is too small to incorporate into a perovskite lattice.13
Other efforts have also been reported to form stable perovskites such as SCN− (thiocyanate), Ac−, HCOO−, BF4− (tetrafluoroborate), and PF6− (hexafluorophosphate).2,14–17 Yan et al. delivered efficient and stable PSCs by using Pb(SCN)2 in a perovskite precursor.18 This SCN−-modulated perovskite film is a high-quality perovskite film with increased grain size. Other studies with the HCCO− anion have also been investigated. Estroff and Abate et al. enabled an excellent coverage and large crystal domains with the use of MAHCOO.19,20 Grätzel et al. used FAHCOO to suppress the anion-vacancy defects and non-radiative recombination in FAPbI3 perovskites.2 The BF4− anion plays a key role in improving charge extraction in the interface and reducing the voltage deficiency.17,21 An appropriate amount of PF6− addition could stabilize the Pb–X–Pb octahedral frame in perovskites, which is beneficial for the less non-radiative recombination and enlarged carrier lifetime.21 Notably, these nonhalides have shown various functions in realizing superiorly stable and high-performance PSCs. However, the effects of anions beyond I−, Cl−, and Br− on perovskites have yet to be fundamentally understood.
In this review, we first describe the basic understanding of the structural and photoelectric properties in nonhalides including pseudohalides and superhalogens. Then we comprehensively summarize the current research progress of SCN−, HCCO−, Ac−, BF4−, BH4−, and PF6− anions to realize highly structurally stable perovskite materials and devices. Their functions in perovskites are systematically summarized, including the strong interaction with perovskites, nucleation and growth, phase stability, efficiency, stability enhancement, and defect passivation. Finally, based on these observations, we propose our perspectives on the future efforts towards achieving highly stable PSCs and devices.
2. Overview of nonhalide anions
To obtain a typical 3D perovskite crystal, the crystallographic stability is determined by Goldschmidt's tolerance criteria (t) and octahedral factor (μ):22 | 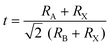 | (1) |
|  | (2) |
where RA, RB, and RX are the ion radii of A, B, and X site ions. To form a stable perovskite crystal with a 3D framework, the value of t and μ must be in the range of 0.81 < t < 1.11, and 0.44 < μ < 0.90, respectively.23,24
The X-site anions are not limited to the three halides (I−, Cl−, and Br−), alternatively, it can be defined as negative monovalent anions or polyatomic anions with −1 charge. The ion radius shows a decreasing trend: RCl− (181 pm) < RBr− (196 pm) < RI− (220 pm). There are two types of nonhalides in perovskites (Fig. 1a). On the one hand, the stable pseudohalides (e.g. SCN−, Ac−, and HCOO−) have gained much attention. SCN− has a similar ion radius to I− (217 pm for SCN−).25 The linear Lewis structure of SCN− showed stable resonance, rendering a strong Lewis acid-base interaction with Pb2+ or Sn2+ when considering the long pairs of electrons from N and S atoms.26,27 HCOO− has a stronger bonding with FA+ than BF4−, I−, Br−, and Cl− owing to the hydrogen bonding.2 The most deleterious defects of I− vacancies can be eliminated with HCOO−. On the other hand, superhalogens such as BH4−, PF6−, and BF4− have a higher electronegative and electron affinity than halides.28,29 The use of superhalogens greatly enhances the structural stability via partial substitution of halides. Therefore, the recent emergence of pseudohalides or superhalogens can significantly mitigate the defect-related instability in PSCs.
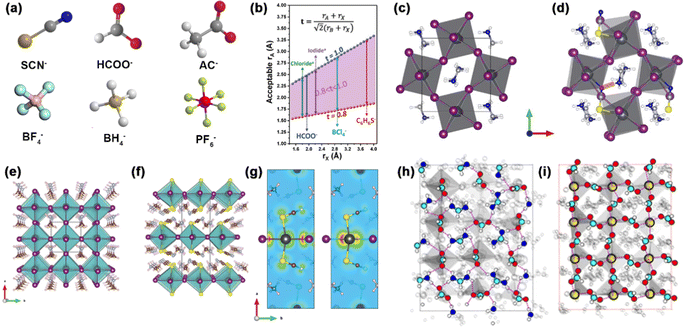 |
| Fig. 1 (a) The structure of nonhalides in perovskites. (b) The range of ion radii of A-site and X-site ions that satisfy 0.81 < t < 1.11. Reproduced with permission.30 Copyright 2019, Wiley-VCH Publications. Crystal structure of (c) MAPbI3 and (d) MAPb(SCN)I3−x. Reproduced with permission.37 Copyright 2015, Springer Nature. Crystal structure of (e) MAPbI3 and (f) MA2Pb(SCN)2I2. (g) Charge density isosurface of VBM (left) and CBM (right) in MA2Pb(SCN)2I2. Reproduced with permission.38 Copyright 2015, American Chemical Society. Top view of surface atoms in (h) the FA+-terminated surface and (i) Pb2+-terminated surface. Reproduced with permission.2 Copyright 2020, Springer Nature. | |
2.1 Pseudohalides
The effective ion radii of polyatomic anions usually satisfy the following criteria:30 | 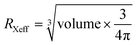 | (3) |
It is reliable to apply a general comparison of the relative ion size of the pseudohalides. To satisfy Goldschmidt's tolerance criteria, the range of A-site cations increased with the size of X-site anions (Fig. 1b). The maximum value of spherical X anions is RX = 2.42 RB (μ ≥ 0.414) to stabilize the [BX6]4− octahedral. Therefore, the X-site anions with a size up to 287 pm can form a stable [BX6]4− octahedral, which allows the size of A sites up to 288 pm in lead perovskites. For nonspherical X anions, the effective RX may be greater than 2.42 RB, which depends on how the ligands of the X site coordinate to the B site.30 The pseudohalides mainly contain N3− (azides),31 CN− (cyanides),32 N(CN)2− (dicyanamides),33 SCN−,29 HCOO−, 2Ac−,34 and I3− (triiodides).35 Beside the Pb- and Sn-based perovskites, the perovskite-like lattice can accommodate other B-site cations, such as [(CH3)4N]Mn(N3)3, Ni(NH3)(CN2)·C6H6, [NH3(CH2)7NH3]2Au2I6(I3)2, MAMn(HCOO)3, etc.31,32,35,36 The polyatomic HCOO− anion has a similar size to Br− and a planar structure.
SCN− has been extensively investigated for improving the stability of perovskites. Yan et al. calculated the crystal structure of MAPbIx(SCN)3−x perovskites by density functional theory (DFT) calculation (Fig. 1c and d).37 The incorporation of the SCN− group delivered a thermodynamically stable lattice with a pseudo-orthorhombic framework.37 Ganose et al. also verified the thermodynamically stable structure in 2D hybrid perovskites (Fig. 1e and f).38 The 2D MA2Pb(SCN)2I2 layered perovskite has a space group of Pnm21, where the Pb atom octahedrally coordinated to two apical (or trans) S-bonded SCN− anions and four axial I− anions. Meanwhile, the MA+ molecule was sandwiched between the layers, forming an orthorhombic pattern like the K2NiF4 compound. SCN− has a great role in bonding, where the valence band maximum (VBM) and conduction band minimum (CBM) is determined by Pb 6p-I 5p and Pb 6p orbitals, respectively (Fig. 1g). There is also a small contribution of S 3p and N 2p in the SCN− in VBM. HCOO− was rarely reported in the inorganic–organic hybrid perovskites. Instead, it can coordinate with Mn2+ cations and form a MAMn(HCOO)3 structure. It plays a significant role in eliminating halide vacancy defects and alleviates the adverse ionic conductivity of perovskites (Fig. 1h and i).2
2.2 Superhalogens
Superhalogens have a higher electron affinity than halides, such as BH4−, BF4−, and PF6−.39 Ogale et al. first applied them to solid-state dye-sensitized solar cells as a component of the X site by using MABF4.40 BF4− and PF6− could partially substitute I− to form stable organic–inorganic perovskite superlattices, which provide a large variety of possibility to expand the possible perovskite formulations.41,42 The addition of BF4− in FAPbI3 could enhance the formation energy of FA+-related vacancies to improve the structural stability.42 BF4− has a higher electronegativity than the F−, implying that the bonding between BF4− and its neighboring Pb atom would be more ionic. Li et al. calculated the VBM and CBM of the minimum-energy superlattice structures of (FAPbI3)3/CsPbI2BF4, (MAPbI3)3/CsPbI2BF4, (FAPbI3)3/MAPbI2BF4, and (CsPbI3)3/MAPbI2BF4 (Fig. 2a–d and e–h).43 The charge density in (MAPbI3)3/CsPbI2BF4, (FAPbI3)3/MAPbI2BF4 illustrated that the VBM and CBM states were distinctly separated in space and had no slap. While the charge density of VBM and CBM value in (FAPbI3)3/CsPbI2BF4 and (CsPbI3)3/MAPbI2BF4 had a slight overlap on the Pb atoms and was far away from the CsPbI2BF4 and MAPbI2BF4 layers. They also calculated the layers of FAPbI3, MAPbI3, and CsPbI3 from one to three along the direction of the base vector a. The complete separation of charge density in VBM and CBM states is beneficial to the electrons/holes collection owing to the effective electrons/holes restriction in different atomic layers. Increasing the number of layers could solve the incomplete separation of the charge density of the VBM and CBM states.
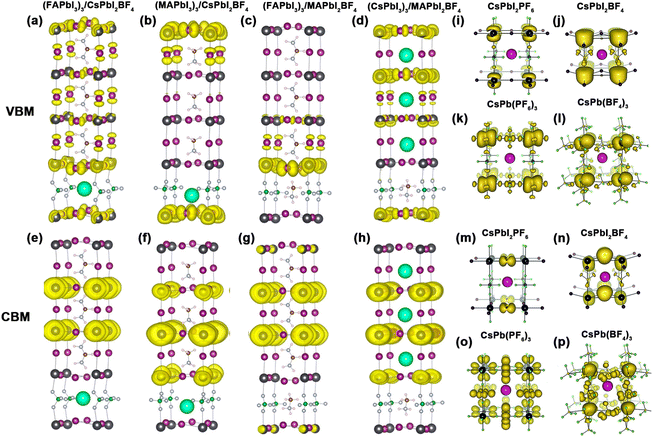 |
| Fig. 2 Charge density in the (a–d) VBM and (e–h) CBM states of (FAPbI3)3/CsPbI2BF4, (MAPbI3)3/CsPbI2BF4, (FAPbI3)3/MAPbI2BF4, and (CsPbI3)3/MAPbI2BF4, respectively. Reproduced with permission.43 Copyright 2020, American Chemical Society. Charge density in the (i–l) VBM and (m–p) CBM states of CsPbI2PF6, CsPbI2BF4, CsPb(PF6)3, and CsPb(BF4)3, respectively. Reproduced with permission.41 Copyright 2015, The Royal Society of Chemistry. | |
For perovskite superlattices, the electrons are distributed in the potential well and holes in the potential barrier. For instance, the superlattice with BF4− is a type-II superlattice.43 The great spatial separation of electrons/holes with superhalogens has a significant effect on carrier transport and collection. In addition, Hendon et al. simulated the crystal structure with VBM and CBM states of CsPbI3−x(PF6)x and CsPbI3−x(BF4)x perovskites as shown in Fig. 2d–p.41 Compared to the octahedral CsPbI3 perovskite (pm3m), CsPb(PF6)3 maintained a cubic lattice by the linear coordination through the axial termini. CsPb(BF4)3 had Cs symmetry with C1m1 space group. The B–F bond in CsPb(BF4)3 was not inline owing to the linear coordination occurring by two edges of the tetrahedron. As for BH4−, it consists of two elements and has one extra electron satisfying the electronic shell closure.44 In addition to the suppression of I− vacancy defects, BH4− has a strong dihydrogen interaction with MA+. The existence of BH4− could substitute the I− anion or compensate for the I− vacancy defects.45,46
3. SCN−
3.1 Acting as an X-site anion in halide perovskites
Bilaji et al. first used a pseudohalide salt of Pb(SCN)2 and Pb(NO3)2 with MAI vapors through a sequential vapor-assisted solution process47 to achieve the ion exchange of SCN− and I−, or NO3− and I−, respectively. Yan et al. used Pb(SCN)2 precursor and prepared a high-quality MAPbI3−xSCNx perovskite film.37 The S and N atoms with long pairs of electrons in SCN− have strong interaction with Pb atoms, which in turn stabilized the frame structure of MAPbI(SCN)2. Jiang et al. compared the X-ray diffraction (XRD) patterns of MAPbI3 and MAPbI(SCN)2 (Fig. 3a).48 The characteristic peaks at 14°, 20°, 29°, 32°, and 41° for the MAPbI(SCN)2 film could be indexed to the formed perovskite structure. They designed moisture-tolerance tests via the reflection spectrum in Fig. 3b and c. There is no obvious variation in the film color of the MAPbI(SCN)2 film for 0–1.5 h. Moreover, the black MAPbI(SCN)2 film still maintained the original morphology for months in air with 40% relative humidity. After exposure to air with 95% humidity, the MAPbI(SCN)2 remained the stable perovskite structure with an Eg of 1.532 eV. The resulting PSC device with MAPbI(SCN)2 delivered a PCE of 8.3%. Yan et al. indicated that adding Pb(SCN)2 below 1.0 mmol% did not change the Eg value of FA0.8Cs0.2Pb(I0.7Br0.3)3 perovskites.49 Lou et al. also illustrated that the pure CsPb(SCN)3 displayed the highest Eg compared with CsPbBr3 and CsPbI3 perovskites.50 The up-shifted VBM was responsible for the increase of Eg. The strong interaction between SCN− and Pb2+ (like Pb–S, Pb–N, and Pb–C bonds) lead to a rather high-energy anti-bonding orbital when compared to CsPbBr3 and CsPbI3.
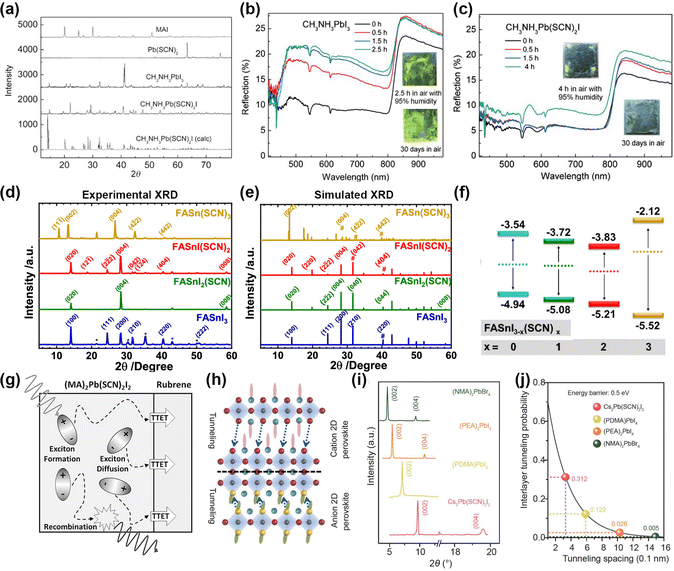 |
| Fig. 3 (a) XRD patterns of MAPbI3 and MAPbI2(SCN) perovskite films. (b and c) Reflection spectrum with stability tests of MAPbI3 and MAPbI2(SCN) in the air with 95% humidity. Reproduced with permission.48 Copyright 2015, Wiley-VCH Publications. (d and e) Experimental and simulated XRD patterns of FASnI3−x(SCN)x perovskite films. (f) Energy level diagrams of FASnI3−x(SCN)x perovskites. Reproduced with permission.52 Copyright 2020, American Chemical Society. (g) Exciton diffusion and triplet-triplet energy transfer at the 2D perovskites/rubrene interface. Reproduced with permission.55 Copyright 2017, Wiley-VCH Publication. (h) The lattice structure of Cs2PbI2(SCN)2 perovskites. (i) XRD patterns of four types of 2D perovskites. (j) Relationship between charge channeling probability and interlayer spacing. Reproduced with permission.57 Copyright 2021, Springer Nature. | |
Meanwhile, SCN− has a strong ionic interaction with Sn2+.51 Owing to the π-conjugated electron system, the incorporation of SCN− showed great enhancement in photoelectron transfer.52,53 Diau et al. observed the enhanced structural stability in FASnI3−x(SCN)x perovskites and a maximum was reached in FASnI(SCN)2.52 Indeed, SCN− was deemed as a bridging ligand to construct a stable octahedra framework due to its ambident nucleophilicity. The XRD patterns in Fig. 3d and e showed three main perovskite peaks around 14°, 28°, and 41° in FASnI3−x(SCN)x perovskites. However, the diffraction peaks of FASn(SCN)3 shifted to smaller angles with the increase of lattice spacing. Similar to the other mixed-halide substitution,54 adding SCN− in FASnI3−x(SCN)x films showed a decreased VBM value when increasing x from 0 to 3 (Fig. 3f). Besides, FASnI3−x(SCN)x has enhanced oxidation resistance, which is attributed to the enhanced hydrophobicity and strong interaction with Sn2+.
The 2D (MA)2Pb(SCN)2I2 perovskites exhibit a photoactive triplet state.55 After spin-coating rubrene, a small organic molecule, the obvious triplet energy transfer from (MA)2Pb(SCN)2I2 to rubrene was observed (Fig. 3g). This phenomenon exhibited unique photophysical behavior with triplet state phosphorescence. Therefore, 2D perovskites with SCN− have great potential in the use of triplet sensitizer. Moreover, 2D Cs2PbI2(SCN)2 shows high thermal stability.56,57 Meng et al. explored the photoelectric properties of Cs2PbI2(SCN)2 perovskites.57 The charge transport across the inorganic layers is determined by the quantum tunneling effect. The space between inorganic layers showed negligible contributions to VBM and CBM. It was observed that Cs2PbI2(SCN)2 had a crystal orientation along the [001] direction like (NMA)2PbBr4, (PEA)PbI4, and (PDMA)PbI4. However, the (002) and (004) peaks shifted to larger angles with decreasing size of the cation or anion (Fig. 3i). Meanwhile, the interlayer tunneling probability of the Cs2PbI2(SCN)2 perovskite reached 0.312, which was ten times higher than those of (NMA)2PbBr4 and (PDMA)PbI4 (Fig. 3j). Large tunneling probability and small interlayer spacing contributed to fast charge transport with high conductivity. Therefore, 2D Cs2PbI2(SCN)2 perovskites have super small interlayer spacing and interlayer nano channels, which will promote the charge transport.
Ning et al. employed PEAI and Sn(SCN)2 to form 2D PEA2Sn2I4−xSCNx perovskites as an intermediate template for the growth of 3D CsSnBrI2−xSCN perovskites.58 This approach enabled the preferred out-of-plane growth of a 2D–3D mixed structure with homogeneous inclusion of SCN− in the 3D CsSnBrI2−xSCN perovskite structure, further contributing to the suppressed defect density and retarded Sn2+ oxidation. The pseudohalide-modulated 2D perovskites also show great potential in tuning the electronic properties of perovskites. Liu et al. adopt the 2D Sn perovskites with pseudohalide to adjust the electronic and excitonic properties.59 The 2D BA2SnI2SCN2 and BA2SnI4 perovskites had the smallest exciton binding energy and effective mass compared with the Pb- and Br-based 2D perovskites. The strong exciton effect in pseudohalide-substituted 2D tin perovskites could enhance PL performance as well as photocurrent when applied in PSCs according to the J–V curve.
3.2 Acting as additives in halide perovskites
3.2.1 Amine-type additives.
GUASCN (guanidinium thiocyanate).
The strong binding affinity of SCN− could recover the oxidized sites and coordinate with undercoordinated sites to passivate the defects, which cannot be reached by classical halides.27,60,61 The strong hydrogen bonding between GUA+ and SCN− further contributes to the additional stabilization of perovskites. Pham et al. elucidated the grain growth mechanism with GUASCN:62 | MAPbI3 + GUASCN → GUAI (s) + PbI2 (s) + MA (g) + HSCN (g)↑ | (4) |
With the surface treatment of GUASCN, the HSCN gas was expelled from the film rapidly and the formed GUAI, PbI2, or the ((GUAI)x(PbI2)y) compound accumulated at GB as a secondary phase. The MA gas enhanced the de-nucleation rate and solubility of unwanted small MAPbI3 crystals, thus facilitating grain coarsening. Zhu et al. proved that adding 7 mol% GUASCN can substantially improve the carrier lifetime up to 1 μs in Sn–Pb perovskite films.14,63 The calculated surface recombination velocity (S) and carrier diffusion coefficient (D) from the transient reflection (TR) spectrum in Fig. 4a and b illustrated that the fitted S increased from 10–100 cm s−1 to 1.0 × 102 cm s−1. GUASCN is a common additive to reduce the pinholes and small grains in hybrid halide perovskites. Zhang et al. claimed that GUASCN could inhibit the 1D solvate intermediate phase and form high-quality quasi-2D films.64 The following reaction revealed the potential mechanism: | MAPbI3·DMF + yGUASCN → y/xPbI2−xSCNx + (1 − y/x)PbI2 + MAI + yGUAI + DMF | (5) |
The decomposition products of GUAI and PbI2−xSCNx enlarged the grain size and enhanced the crystalline quality of thin films. The fitted rapid rise time (τ) by ultrafast transient absorption (TA) spectroscopy in Fig. 4c and d indicated that the perovskite film with GUASCN had faster τ of 4.43 ps, 4.78 ps, and 107.90 ps for n = 3, 4, and 5, respectively, where the control films had τ of 0.15 ps (n = 2), 6.78 ps (n = 3), 8.86 ps (n = 4), and 13.21 ps (n = 5), respectively. Therefore, GUASCN significantly improved the energy/charge transfer efficiency due to a suppressed recombination center and effective energy/charge transfer. A similar approach was also reported in colloidal quantum dot (CQD) photovoltaics.65 The bidentate nature of SCN− leads to improved electronic coupling in the CQD matrix.2,66,67 GUASCN has nearly zero dipole moment, thus benefiting charge transport. By surface reconstruction, GUASCN played a key role in surface passivation via epitaxial surface bridges with CQD. The oxidized species were eliminated with GUASCN by substituting Pb–O to the Pb–SCN bond (Fig. 4e). The upshifted C
N stretch in Fig. 4f proved the surface reconstruction between the SCN− and CQD surface.
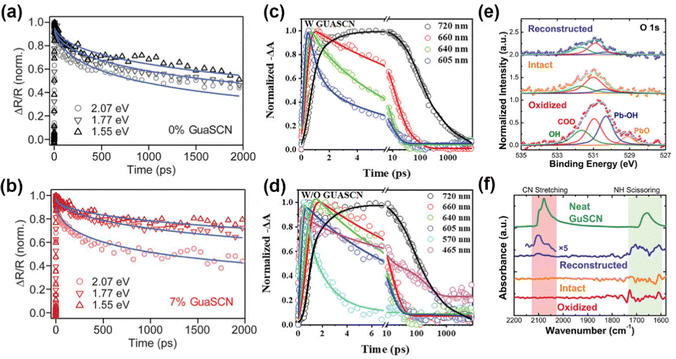 |
| Fig. 4 (a and b) TA spectra of 0% and 7% GUASCN addition under different pump photon energies. Reproduced with permission.14 Copyright 2019, American Association for the Advancement of Science. (c and d) TA kinetics of quasi-2D perovskites without/with GUASCN. Reproduced with permission.64 Copyright 2022, Wiley-VCH Publication. (e) XPS peaks of O 1s. (f) FTIR spectra of the CQD solids and neat GUASCN. Reproduced with permission.65 Copyright 2022, American Chemical Society. | |
NH4SCN.
The pseudohalide SCN− anion could drive the secondary formation of thin films as the intermediate catalyst, therefore ensuring the composition purity both in the original films and final polycrystals. The SCN− group in NH4SCN was not incorporated into the perovskite lattice in the final-state perovskite films. Jen et al. proposed the recrystallization process with NH4SCN:68 | MAPbI3 + SCN− → PbI64− + MA+·SCN− (dipping) | (6) |
| MA+·SCN− + NH4+ → MA+ + HSCN (g)↑ + NH3 (g)↑ (annealing) | (7) |
Therefore, the undissolved polycrystals could recrystallized and form new MAPbI3 crystal. The SCN− anions in NH4SCN can strongly interact with Pb2+/Sn2+ and the organic cations, where NH4+ cations could interact with [PbI6]4− or [SnI6]4−.68–70 Chou et al. revealed the role of in 2D tin perovskites by time-of-flight secondary ion mass spectrometry (ToF-SIMS) to detect the location of SCN− anions (Fig. 5a and b).71 The positive and negative signals of Sn, I, SCN, and TEA (2-thiophene-ethylammonium) were located at the same region, which indicated the SCN can coordinate with Sn2+. Zhang et al. investigated the NH4SCN concentration in the orientation and crystallinity of 2D (PEA)2(MA)n−1PbnI3n+1 films (Fig. 5c–e).72 The 2D perovskites in the absence of NH4SCN showed random orientation and weak preferential orientation, while the film with 2% SCN− had sharp and discrete Bragg spots and a considerable increase in orientation.
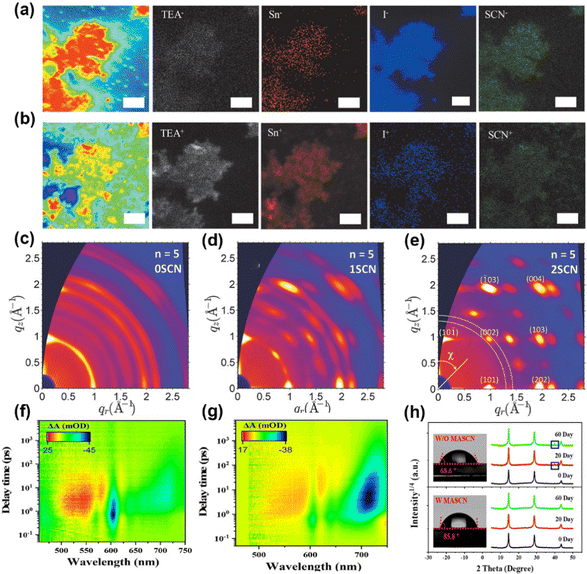 |
| Fig. 5 ToF-SIMS mapping of 2D perovskites with 7% NH4SCN: (a) negative signals of TEA−, Sn−, I−, and SCN−; (b) positive signals of TEA+, Sn+, I+, and SCN+. Reproduced with permission.71 Copyright 2020, Wiley-VCH Publication. (c–e) 2D GIWAX patterns of 2D perovskite films with various amounts of NH4SCN. Reproduced with permission.72 Copyright 2018, Wiley-VCH Publication. TA patterns of (PA)2(MA)4Pb5I16 film (f) without and (g) with MASCN. (h) XRD images and contact angle tests with or without MASCN. Reproduced with permission.75 Copyright 2022, American Chemical Society. | |
MASCN and FASCN.
MASCN can significantly reduce the reaction activity via the strong interaction with Sn2+/Pb2+.73–75 For instance, Lian et al. proved that MASCN can retard the reaction between FAI and Sn2+ and also suppress the Sn2+ oxidation.74 Zhang et al. added 5% MASCN into (PA)2(MA)4Pb5I16 (PA is n-pentylammonium) perovskite precursor to regulate the phase distribution.75 The TA patterns in Fig. 5f at various delay times indicated that the peaks at 570 nm, 606 nm, 640 nm, 666 nm, and 720 nm attributed to n = 2, n = 3, n =4, n = 5, and 3D-like phases in (PA)2(MA)4Pb5I16 film, respectively. The n = 2 phase disappeared and the 3D-like phase enhanced remarkably in MASCN-modified films (Fig. 5g), further proving that the perovskite film with SCN− obtained a high n-value phase and large grain. Moreover, the improved water contact implied great hydrophobicity of perovskite films in Fig. 5h. The XRD patterns in pseudohalide-induced perovskite films had barely changed 2D phases after storing for 60 days. Different from the previous report, Grätzel et al. used MASCN and FASCN vapor to achieve phase transition and obtain stable black-phase FAPbI3 perovskites.76 The formed corner-sharing Pb–I–SCN structures at the interface could maintain kinetically stable perovskites. The vapor-treated FAPbI3 devices maintained 94.4% PCE of the original value after 500 h testing at the maximum power point (MPP).
PEASCN and TEASCN.
Compared to pure PEAI treatment, the PEASCN source conformed to a more crystalline film.77 The PEASCN sample can reach a carrier lifetime of over 2 μs and carrier mobility over 40 cm−2 V−1 s−1. Khadka et al. introduced PEASCN into pure FASnI3 perovskites to achieve defect suppression in the bulk and interface.78 These polyatomic pseudohalides delivered a high PCE of 9.65% with a Voc of 667 mV in the corresponding devices. Therefore, the PEASCN not only acts as a 2D additive but also as a crystallization regulator. Ning et al. synthesized a new pseudohalide molecule TEASCN (TEA is 2-thiopheneethylamine) to construct a bilayer quasi-2D structure on the Sn–Pb perovskite surface.79 The TEASCN-introduced films enabled a high n-value (n = 2) phase while the TEAI-introduced films formed a low n-value phase of n = 1.
3.2.2 Metal-type additives.
KSCN and NaSCN are the most commonly used additives in perovskites.80–84 Zhang et al. used the two additives to improve the microstructure perovskite structure and crystallinity of thin films.18 The NaSCN treatment could suppress the Br− vacancies and decreased the surface defect density, thus further increasing the device stability.82 Besides the effect of SCN−, K+ could also passivate defects. Xu et al. found that KSCN can enlarge perovskite grains to an average diameter of 11 μm.81 This attempt enabled the carrier diffusion length to around 25 μm in slot-die-coated perovskite films. Additionally, Cao et al. used KSCN as an interlayer between PEDOT:PSS and FA0.75MA0.25SnI2Br perovskites to better match the energy alignment and optimize carrier transport in the interface.83 The resulting tin perovskite device delivered a high PCE of over 11% with a Voc of 840 mV. On the one hand, K+ diffused into the lattice and passivate defects in the GB. On the other hand, SCN− anions coordinate with Sn2+ and restrain Sn2+ oxidation.
4. HCOO− and Ac−
Pseudohalides HCOO− and Ac− anions could affect the crystallization and optoelectronic properties of perovskites. Adding a small amount of HCOO− and Ac− anions in precursors could achieve compact surface coverage and improve the crystallinity of perovskite thin films.85 Interestingly, formic acid (FAH) is identified as one of the degradation products of DMF.86 Introducing additional FAH in the precursor enables the reduction of the hydrolysis of DMF. A 70–80 mV increased Voc was achieved with 7.5 vol% FAH. Increasing the acidity would raise the concentration of all solutes and decrease the strength of solvents in the precursor, thus inducing supersaturation and crystal growth.87 Meng et al. observed dense nuclei with the snowflake-like crystal of FAH-induced perovskite film within 3.5 min as shown in Fig. 6a and b.88 The control film showed almost no nucleation after the spin-coating process. Meanwhile, FAH is an ideal redox potential and has no negative effects in nucleation and crystal growth. Liu et al. pointed out the following reactions of FAH effect in precursor:89 | HCCO− + 2I0 → 2I− + H+ + CO2 (g)↑ | (9) |
| HCCO− + I3− → 3I− + H+ + CO2 (g)↑ | (10) |
HCOO− in FAH can react with adverse I0 and form I−, H+, and CO2 gas. The generated H+ further inhibited the deprotonation of FA+. In addition, the FAMACs films with 2 mol% FAH showed higher photoluminescence intensity (Fig. 6c) and longer lifetime (Fig. 6d). Han et al. further reported that FAH enhanced the crystallinity of Sn-based perovskites and exhibited high reproducibility and PCE in the corresponding devices.90
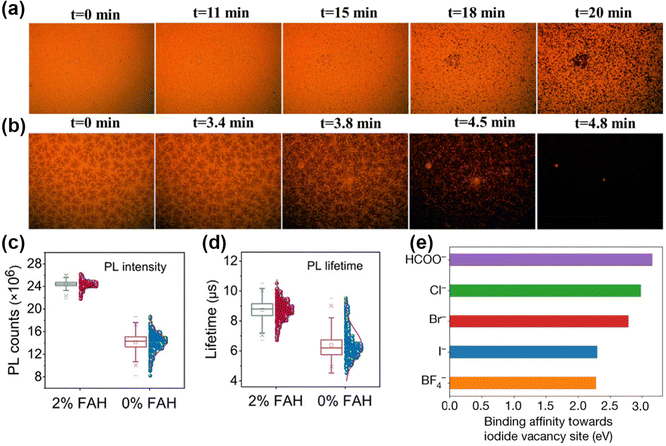 |
| Fig. 6 (a and b) In situ microscopy patterns with 0 M and 0.764 M FAH-treated perovskite films. Reproduced with permission.88 Copyright, 2020, Elsevier Publication. (c and d) PL intensities and lifetimes of perovskite films with or without FAH treatment. Reproduced with permission.89 Copyright 2021, American Association for the Advancement of Science. (e) The interaction strengths of different anions with I−-vacancy defects at the perovskite surface. Reproduced with permission.2 Copyright 2021, Springer Nature. | |
Estroff et al. first used MAFA (methylammonium formate), an ionic liquid (IL), in MAPbI3 perovskites.19 In addition to the advantages of HCOO−, the property of ILs also played a key role in film growth due to their outstanding thermal stability.16,91 Abate et al. indicated that the crystallization and growth process with MAFA went through the following steps:20 (i) the coordination of Pb2+ and HCOO− in precursor; (ii) the HCOO-Pb bond replaced by I-Pb bond when annealing at 100 °C; (iii) complete replacement by I-Pb bond and crystal growth. The MAFA was presented at the surface in the final form. By utilizing the pseudohalide anion, Jeong et al. attained a certified PCE of 25.2% and remarkable operational stability over 450 h.2 HCOO− anions showed a strong affinity with Pb2+ and can form a stable bonding network at the Pb2+-terminated surface. As exhibited in Fig. 6e, the highest binding energy with I− vacancies in HCOO− also proved the strong defect passivation of anion vacancies via strong binding affinity.
Similar to HCOO−, volatile Ac− anions also can form an intermediate with perovskite and modulate the crystallization process.34,92 Zhou et al. proposed a novel strategy to suppress adverse I2 to reduce the energy and voltage loss.92 FAAc (formamidine acetate) as a “residual free” weak alkaline can reduce the defect density at a deep level and in particular manipulate the stoichiometry at A sites in perovskite precursors. The characteristic peak of I2 at 360 nm showed a decreased tendency with the increasing amount of FAAc (Fig. 7a). As for the alkalinity, FAAc has a pKa of around 7.64, whereas other alkaline additives such as NaAc (sodium acetate) and NaHCO3 have a pKa of 9.24 and 10.33, respectively. Therefore, a large range of FAAc can regulate the absorbance of I2 (Fig. 7b), which indicated that the weak alkaline had a great potential to regulate the α-phase perovskites on a large scale. Additionally, the volatile Ac− facilitated grain growth with enlarged grain size and film quality. Lv et al. further used MAAc in the Sn–Pb perovskite precursor.93 The strong hydrogen bonding between MAI and MAAc can not only stabilize the perovskite lattice but also increase the viscosity and solubility of the solute in the solution.
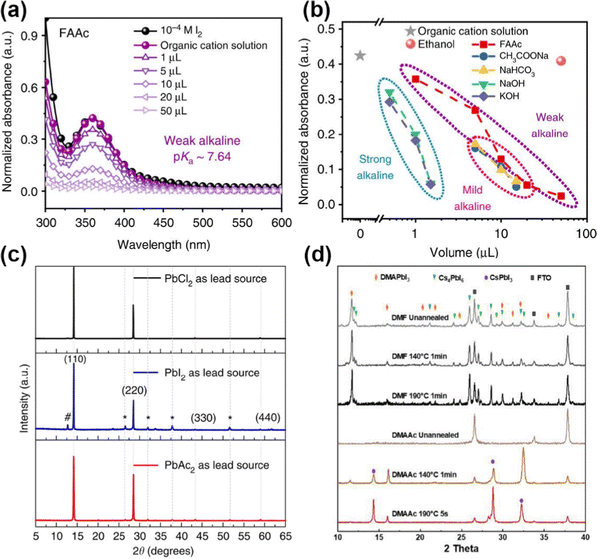 |
| Fig. 7 (a) UV-Vis absorption of perovskite film with different amounts of FAAc. (b) Normalized absorbance with different additives. Reproduced with permission.92 Copyright 2019, Springer Nature. (c) XRD patterns of perovskite films with PbCl2, PbI2, and PbAc2 sources. Reproduced with permission.15 Copyright 2015, Springer Nature. (d) XRD patterns of CsPbI3-DMAAc precursor with different annealing times. Reproduced with permission.94 Copyright 2022, Wiley-VCH Publication. | |
PbAc2 as a pseudohalide lead source has also been attempted to improve the perovskite growth and film morphology.15 Compared to PbCl2 and PbI2 as displayed in Fig. 7c, the perovskite film from PbAc2 revealed better crystallinity. The transition process can be described as:
| PbX2 + 3MAI → MAPbI3 + 2MAX (X = Cl, I, Ac) | (11) |
The thermally unstable MAAc tends to evaporate more rapidly than MACl and MAI under annealing. The nucleation density with PbAc
2 was higher than others and the film showed full coverage on the substrate. Meng
et al. eliminated the Cs
4PbI
6 intermediate phase by DMAAc (dimethylamine acetate) and stabilized the lattice structure of DMAPbI
3.
94 As shown in
Fig. 7d, after annealing at 140 °C for 1 min, some DMAPbI
3 crystalline phase appeared in the absence of the Cs
4PbI
6 phase. After annealing at 190 °C for 5 s, there was no Cs
4PbI
6 phase either. Thus, the incorporation of DMAAc can shorten the crystal growth time. When annealing further, the coexisting of DMAPbI
2Ac and DMAPbI
3 affords a dynamically stable DMAPb(I, Ac)
3 as follows:
| PbI2 + DMAAc → DMAPbI2Ac | (12) |
| DMAPbI3 + DMAPbI2Ac → DMAPb(I,Ac)3 | (13) |
| DMAPb(I,Ac)3 + Cs+ + I− → CaPbI3 + DMA (g)↑ + HAc + HI (g)↑ | (14) |
Therefore, DMAAc can accelerate the phase transformation from intermediate to thermally stable CsPbI
3 phase. Additionally, Huang
et al. achieved a high
Voc up to 1.31 V and superior air stability for 4680 h with
n-butylamine acetate (BAAc).
95 The hydrogen bonding of N–H⋯O
![[double bond, length as m-dash]](https://www.rsc.org/images/entities/char_e001.gif)
C–O
− in BAAc has a stronger shielding effect than N–H⋯I
− in BAI. The vertically aligned grains can be crystallized from the uniformly distributed intermediate.
5. BF4− and BH4−
An interesting strategy to introduce F− in perovskite with BF4− anion has been investigated.28,96–98 BF4− contributes to large perturbation to the electronic structure. Considering the similar ion radii of I− and BF4−, Han et al. investigated MAPbI3−x(BF4)x perovskites with different BF4− concentrations.28 All MAPbI3−x(BF4)x perovskites had the same tetragonal crystal structure like the MAPbI3 perovskites. The introduction of BF4− leads to an obvious change in frequency-dependent electrical conductivity. In the same vein, Nagane et al. studied the mechanism of BF4− modulation via solid-state NMR (ssNMR) at the atomic level.99 It was reported that BF4− anion was not incorporated into the perovskite lattice, but reacted with MAI like a scavenger. As shown in Fig. 8a, the F− signal of BF4− was located at −147.8 ppm with a broadened signal after mixing equimolar amounts of MAI and MABF4, indicating the formed MAI-MABF4 cocrystal. Additionally, 10 mol% excess MAI was added with BF4−and the F− signal was the characteristic peak of MAI-MABF4 cocrystals. The δ peak at 43.9 ppm in the 11B spectrum had no change in Fig. 8b, further proving that the BF4− was not incorporated into the perovskite lattice.
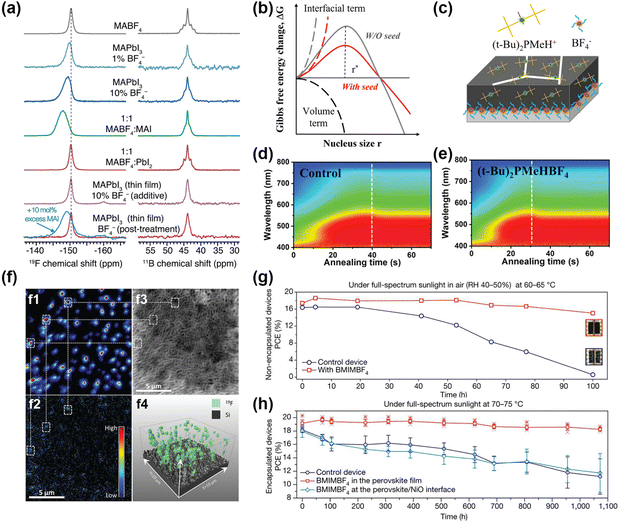 |
| Fig. 8 (a) The 19F (left) and 11B NMR (right) spectra of MABF4, MAPbI3, MAPbI3 : 1% MABF4, MAPbI3 : 10%MABF4, MABF4: MAI, MABF4:PbI2, and the MAPbI3 film treated with 10% MABF4, respectively. Reproduced with permission.99 Copyright 2021, Wiley-VCH Publication. (b) Gibbs free energies of perovskite nucleation with or without (t-Bu)2PMeHBF4. (c) Growth modulation pattern by (t-Bu)2PMeHBF4. (d and e) In situ UV-Vis absorption spectra with or without (t-Bu)2PMeHBF4. Reproduced with permission.101 Copyright 2022, Wiley-VCH Publication. (f): (f1) 19F− and (f2) 11B16O2− anion maps for the perovskite films with BMPBF4 additives. (f3) Secondary electron map for the sputtered surface morphology. (f4) 3D maps of F− signals. Reproduced with permission.102 Copyright 2020, American Association for the Advancement of Science. Thermal stability with (g) non-encapsulated devices and (h) encapsulated devices with or without BMIMBF4 additives. Reproduced with permission.21 Copyright 2019, Springer Nature. | |
Additionally, imidazolium tetrafluoroborate (IMBF4) containing imidazolium cation and BF4− anion to passivate the Sn–Pb perovskite was achieved by Kim et al.12 The morphological effect and charge carrier dynamics with IMBF4 verified the role of BF4− in optoelectronic properties. Meanwhile, the functional π-conjugated QAPy+ (1,1′-((7,14-dioxo-7,14-dihydroquinolino[2,3-b]acridine-5,12-diyl)bis(octane-8,1-diyl))bis(pyridin-1-ium)) cooperating with BF4− lead to a higher endothermic decomposition temperature, therefore exhibiting better stability compared to the control PSCs device.97 The QAPyBF4 not only suppressed the defects of organic cation vacancies but also improved the interface contact and the adhesion of the buried interface. A signature PCE of 23.1% and Voc up to 1.2 V was achieved with markedly improved thermal stability by the buried QAPyBF4 interface. To demonstrate the crystallization process with BF4−, Zhang et al. applied (t-Bu)2PMeHBF4 ((t-Bu)2PMeH+ is di-tert-butyl(methyl)phosphonium) and analyzed the free energy in the surface/bulk by classical theory (Fig. 8b and c).100 With the assistance of (t-Bu)2PMeHBF4, the PbI2–(t-Bu)2PMeBF4 intermediate was prenucleated as the nucleation center and greatly reduced the interface energy. Thus, the nuclei of perovskite films can occur at a lower saturation due to the low nucleation barrier, further forming small and contact nuclei during the solvent evaporation process (Fig. 8b). Besides the faster nucleation process in the precursor, a faster crystallization process in the thin films was also observed as shown in the in-situ absorption spectra in Fig. 8d and e. The absorption signal of (t-Bu)2PMeHBF4-modified film reached the peak value 10s earlier than the control film.
Snaith et al. claimed that BMPBF4 (BMP+ is 1-butyl-1-methylpiperidinium) could suppress the deep trap states significantly.21 To understand the distribution of BMPBF4 in the perovskites, they utilized the nano SIMS (nanoscale secondary ion mass spectrometry) and analyzed the 19F− and 11B16O2− signals as displayed in Fig. 8f. The 19F− signals were consistent with 11B16O2− signals. F− anions were distributed over the surveyed volume in the perovskite layers to play the role of defect passivation. Snaith et al. also incorporated BMIMBF4 (BMIM+ is 1-butyl-3-methylimidazolium) into perovskite layers and achieved superior long-term thermal stability both in non-encapsulated and encapsulated devices (Fig. 8g and h).21 The BMIM+ was not only located in the bulk perovskites but also accumulated at the buried interface. However, the BF4− was only located at the buried interface. Both the BMIM+ and BF4− suppressed the ion migration in the perovskite films in return.
Compared to halide anions, BH4− is composed of two elements and needed one extra electron for electronic shell closure.46,103,104 The strong dihydrogen interaction between ionic hydrogen in BH4− and the proton hydrogen in MAI can prevent the decomposition of MAI. BH4− has an ion radius of 202 pm, which is similar to halides and can partially substitute these heavy halides such as I−, Br−, or Cl−.41,46 A stable MAPbIx(BH4)3−x was reported by Xu et al.45 The superhalogen BH4− can release anion vacancies and reduce defects such as halide vacancies or organic cation vacancies by partial substitution of I−. Sanchez-Diaz et al. reported the addition of NaBH4 into Sn perovskites for achieving the oxidation resistance from Sn2+ to Sn4+ during the solution processing, which lead to a PCE of 10.61% with superior operational stability over 1300 h in an N2 atmosphere.105 The FASnI3 perovskite film emerged with a compact and pinhole-free morphology with NaBH4 incorporation. In this respect, this new type of superhalogen anion provides a novel design in complex perovskites.
6. PF6−
For the F-type superhalogen PF6−, it has an ion radius of 225 pm and also can be incorporated into a perovskite lattice.41,106 Tao et al. achieved a PCE of 27.35% in 4T (four terminal) perovskite-Si tandem devices by F-type PF6− with a perovskite framework of FA0.65MA0.20Cs0.15Pb(I0.8Br0.2)3−x(PF6)x.106 When partial substitution of I−/Br− by PF6− occurred, the perovskite showed lattice expansion. Meanwhile, there was a released tensile strain with PF6− addition. Therefore, a high-quality perovskite film formed with strain relaxation, which had a positive effect on charge transport and passivation of defect density. Moreover, Hao et al. adopted BMIMPF6 to enhance the phase stability of CsPbI2Br and delivered a PCE of 16.2% with a Voc of 1.28 V.16 The CsPbI2Br with BMIMPF6 had a lower formation energy than the pure α-CsPbI2Br as shown in Fig. 9a, therefore stabilizing the α-CsPbI2Br perovskite. The average Br-I-Br angle (Fig. 9c and d) with BMIMPF6 increased from 78.1° to 88.3°, thus contributing to the enhanced phase stability of α-CsPbI2Br perovskite by restraining the distortion of [BX6]4− octahedrons. With KPF6-assisted crystallization, Bu et al. achieved a high PCE of 23.35% in MA-free PSCs.107 The interface between perovskite and Spiro-OMeTAD was intersected without KPF6 treatment, whereas it had no obvious change after KPF6 treatment as shown in cross-sectional SEM images (Fig. 9d and e). Meanwhile, PF6− stayed on the surface and passivated the grain boundaries and the signals of K+ and F− throughout the perovskite films (Fig. 9f). As a result, the device with KPF6 showed negligible ion migration after thermal aging.
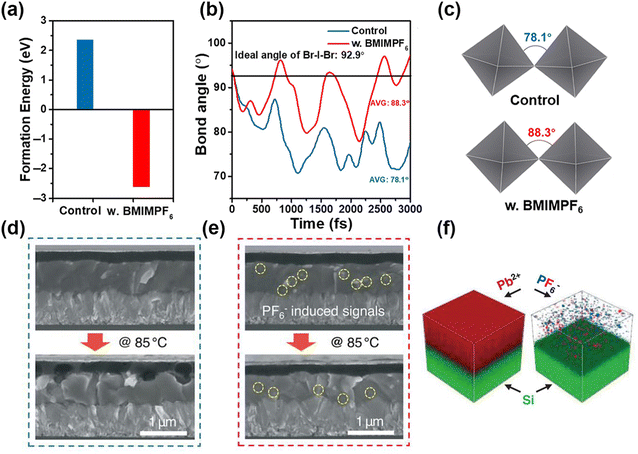 |
| Fig. 9 (a) Formation energy of α-CsPbI2Br with or without BMIMPF6 treatment. (b) Time-dependent bond angle of Br–I–Br angle and (c) the corresponding crystal schematic. Reproduced with permission.16 Copyright 2021, Wiley-VCH Publication. Cross-sectional SEM patterns of perovskite/Spiro-OMeTAD interface (d) without and (e) with KPF6 treatment. (f) TOF-SIMS pattern of Pb, P, and F ions in perovskites after KPF6 addition. Reproduced with permission.107 Copyright 2021, Springer Nature. | |
7. Conclusions and outlooks
The nonhalides act as emerging candidates for tuning the optoelectronic properties of perovskites and their applications in photovoltaics and beyond. The ambient light and thermal stability were greatly enhanced through this strategy. Meanwhile, they can tune the nucleation and crystal growth process and modulate the film growth process. Their positive effects on morphology, stability, electrical conductivity, carrier density, and charge transport properties provide another perspective for the future selection of the X-site anions. To achieve high-efficiency and stable PSCs with this strategy, more fundamental research studies need to be carried out.
(i) More theoretical and experimental studies should determine whether pseudohalides or superhalogens can be incorporated into a perovskite lattice. The propensity of the position in the bulk perovskite lattice or the interface/surface can offer an in depth understanding of halide management including the halide composition and halide defect regulation.
(ii) Due to the high volatile properties of SCN−, the chemical inhomogeneity by local evaporation may be caused by unusual planar defects like edge dislocation or the bending of interlayers. The development of an elaborate vapor treatment method with SCN− can improve the phase transition and the crystallization kinetics.
(iii) Pseudohalides contribute to enlarged grains and few boundaries with high n-value distribution for low-dimensional perovskites. The role of organic cations in such a system cannot be ignored. On the one hand, functionalized organic cations containing both electron-rich atoms and electron-donating chains show a strong passivation effect on the net positive charge in perovskites. On the other hand, the characteristics of the cationic component should also be investigated.
(iv) The passivation effect of HCOO− and Ac− is mostly reflected in the halide vacancies. Meanwhile, they have a strong interaction with B-site cations to stabilize the perovskite lattice structure. The hydrogen bonding network with organic cations further enables the unique role of HCOO− and Ac− in passivating anion-vacancy defects. Though volatile FAH and HAc have no residues in perovskite films after annealing,85,90 long-term passivation from the precursors to thin films at high temperature is missed. Therefore, ionic liquids can be designed with various functional organic cations with pseudohalide anions in this regard. Their special properties with non-volatility and low toxicity will benefit the realization of efficient and stable PSCs.
(v) The passivation with BF4− or PF6− can mainly be embodied in blocking the invasion of H2O and O2, thereby facilitating moisture stability. Designing the functional hydrophilic groups with BF4− or PF6− to meet the appropriate polarity, conductivity, melting point, viscosity, and chirality is an important issue in the development of more efficient and stable PSCs soon.
Conflicts of interest
There are no conflicts to declare.
Acknowledgements
This work was financially supported by the National Key Research & Development Program of China (2022YFE0118400), the National Natural Science Foundation of China NSFC (51702038), the Science & Technology Department of Sichuan Province (2020YFG0061), and the Recruitment Program for Young Professionals. L. Ding thanks the National Key Research and Development Program of China (2017YFA0206600) and the National Natural Science Foundation of China (51773045, 21772030, 51922032, 21961160720) for financial support.
References
- A. Kojima, K. Teshima, Y. Shirai and T. Miyasaka, Organometal Halide Perovskites as Visible-Light Sensitizers for Photovoltaic Cells, J. Am. Chem. Soc., 2009, 131, 6050–6051 CrossRef CAS PubMed
.
- J. Jeong, M. Kim, J. Seo, H. Lu, P. Ahlawat, A. Mishra, Y. Yang, M. A. Hope, F. T. Eickemeyer and M. Kim, Pseudo-Halide Anion Engineering for α-FAPbI3 Perovskite Solar Cells, Nature, 2021, 592, 381–385 CrossRef CAS PubMed
.
- S. Wang, L. Yan, W. Zhu, Z. Cao, L. Zhou, L. Ding and F. Hao, Suppressing the Formation of Tin Vacancy Yields Efficient Lead-Free Perovskite Solar Cells, Nano Energy, 2022, 99, 107416 CrossRef CAS
.
- F. Hao, C. C. Stoumpos, R. P. H. Chang and M. G. Kanatzidis, Anomalous Band Gap Behavior in Mixed Sn and Pb Perovskites Enables Broadening of Absorption Spectrum in Solar Cells, J. Am. Chem. Soc., 2014, 136, 8094–8099 CrossRef CAS PubMed
.
- J. Liu, S. Wang, W. Zhu, Z. Tang, L. Ding and F. Hao, Highly Symmetric Lewis Base Coordinated with Sn2+ for Reducing Voltage Loss and Retarding Oxidation in Tin-Halide Perovskite Solar Cells, Chem. Eng. J., 2022, 139975 Search PubMed
.
- S. Wang, A. Wang, F. Hao and L. Ding, Renaissance of tin halide perovskite solar cells, J. Semicond., 2021, 42, 030201 CrossRef CAS
.
- Y. Rong, Y. Hu, A. Mei, H. Tan, M. I. Saidaminov, S. I. Seok, M. D. McGehee, E. H. Sargent and H. Han, Challenges for Commercializing Perovskite Solar Cells, Science, 2018, 361, eaat8235 CrossRef PubMed
.
- S. Wang, A. Wang and F. Hao, Toward stable lead halide perovskite solar cells: A knob on the A/X sites components, iScience, 2022, 25, 103599 CrossRef CAS PubMed
.
- W. Fu, H. Chen and A. K. Y. Jen, Two-dimensional Perovskites for Photovoltaics, Mater. Today Nano, 2021, 14, 100117 CrossRef CAS
.
- C. Zhang, S. Wang, X. Li, M. Yuan, L. Turyanska and X. Yang, Core/Shell Perovskite Nanocrystals: Synthesis of Highly Efficient and Environmentally Stable FAPbBr3/CsPbBr3 for LED Applications, Adv. Funct. Mater., 2020, 30, 1910582 CrossRef CAS
.
- D. Cortecchia, H. A. Dewi, J. Yin, A. Bruno, S. Chen, T. Baikie, P. P. Boix, M. Grätzel, S. Mhaisalkar, C. Soci and N. Mathews, Lead-Free MA2CuClxBr4–x Hybrid Perovskites, Inorg. Chem., 2016, 55, 1044–1052 CrossRef CAS PubMed
.
- H. Kim, J. W. Lee, G. R. Han, S. K. Kim and J. H. Oh, Synergistic Effects of Cation and Anion in an Ionic Imidazolium Tetrafluoroborate Additive for Improving the Efficiency and Stability of Half-Mixed Pb–Sn Perovskite Solar Cells, Adv. Funct. Mater., 2021, 31, 2008801 CrossRef CAS
.
- Q. Chen, H. Zhou, Y. Fang, A. Z. Stieg, T.-B. Song, H.-H. Wang, X. Xu, Y. Liu, S. Lu and J. You, The Optoelectronic Role of Chlorine in CH3NH3PbI3 (Cl)-based Perovskite Solar Cells, Nat. Commun., 2015, 6, 1–9 CAS
.
- J. Tong, Z. Song, D. H. Kim, X. Chen, C. Chen, A. F. Palmstrom, P. F. Ndione, M. O. Reese, S. P. Dunfield and O. G. Reid, Carrier Lifetimes of >1 μs in Sn–Pb Perovskites enable Efficient All-Perovskite Tandem Solar Cells, Science, 2019, 364, 475–479 CrossRef CAS PubMed
.
- W. Zhang, M. Saliba, D. T. Moore, S. K. Pathak, M. T. Hörantner, T. Stergiopoulos, S. D. Stranks, G. E. Eperon, J. A. Alexander-Webber and A. Abate, Ultrasmooth Organic–Inorganic Perovskite Thin-Film Formation and Crystallization for Efficient Planar Heterojunction Solar Cells, Nat. Commun., 2015, 6, 1–10 CAS
.
- A. Wang, J. Wang, X. Niu, C. Zuo, F. Hao and L. Ding, Inhibiting Octahedral Tilting for Stable CsPbI2Br Solar Cells, InfoMat, 2022, 4, e12263 CAS
.
- M. Li, C. Zhao, Z. K. Wang, C. C. Zhang, H. K. Lee, A. Pockett, J. Barbé, W. C. Tsoi, Y. G. Yang and M. J. Carnie, Interface Modification by Ionic Liquid: a Promising Candidate for Indoor Light Harvesting and Stability Improvement of Planar Perovskite Solar Cells, Adv. Energy Mater., 2018, 8, 1801509 CrossRef
.
- Z. Zhang, Y. Zhou, Y. Cai, H. Liu, Q. Qin, X. Lu, X. Gao, L. Shui, S. Wu and J.-M. Liu, Efficient and Stable CH3NH3PbI3−x(SCN)x Planar Perovskite Solar Cells Fabricated in Ambient Air with Low-Temperature Process, J. Power Sources, 2018, 377, 52–58 CrossRef CAS
.
- D. T. Moore, K. W. Tan, H. Sai, K. P. Barteau, U. Wiesner and L. A. Estroff, Direct Crystallization Route to Methylammonium Lead Iodide Perovskite From an Ionic Liquid, Chem. Mater., 2015, 27, 3197–3199 CrossRef CAS
.
- J. Y. Seo, T. Matsui, J. Luo, J. P. Correa-Baena, F. Giordano, M. Saliba, K. Schenk, A. Ummadisingu, K. Domanski and M. Hadadian, Ionic Liquid Control Crystal Growth to Enhance Planar Perovskite Solar Cells Efficiency, Adv. Energy Mater., 2016, 6, 1600767 CrossRef
.
- S. Bai, P. Da, C. Li, Z. Wang, Z. Yuan, F. Fu, M. Kawecki, X. Liu, N. Sakai and J. T.-W. Wang, Planar Perovskite Solar Cells with Long-Term Stability using Ionic Liquid Additives, Nature, 2019, 571, 245–250 CrossRef CAS PubMed
.
- D. Luo, R. Su, W. Zhang, Q. Gong and R. Zhu, Minimizing non-radiative recombination losses in perovskite solar cells, Nat. Rev. Mater., 2020, 5, 44–60 CrossRef CAS
.
- Z. Cheng and J. Lin, Layered Organic–Inorganic Hybrid Perovskites: Structure, Optical Properties, Film Preparation, Patterning and Templating Engineering, CrystEngComm, 2010, 12, 2646–2662 RSC
.
- M. A. Green, A. Ho-Baillie and H. J. Snaith, The Emergence of Perovskite Solar Cells, Nat. Photonics, 2014, 8, 506–514 CrossRef CAS
.
- A. Halder, R. Chulliyil, A. S. Subbiah, T. Khan, S. Chattoraj, A. Chowdhury and S. K. Sarkar, Pseudohalide (SCN−)-doped MAPbI3 Perovskites: A Few Surprises, J. Phys. Chem. Lett., 2015, 6, 3483–3489 CrossRef CAS PubMed
.
- S. J. Kim, T. H. Lee, J.-M. Yang, J. W. Yang, Y. J. Lee, M.-J. Choi, S. A. Lee, J. M. Suh, K. J. Kwak, J. H. Baek, I. H. Im, D. E. Lee, J. Y. Kim, J. Kim, J. S. Han, S. Y. Kim, D. Lee, N.-G. Park and H. W. Jang, Vertically Aligned Two-Dimensional Halide Perovskites for Reliably Operable Artificial Synapses, Mater. Today, 2022, 52, 19–30 CrossRef CAS
.
- T. Wang, H. L. Loi, J. Cao, Z. Qin, Z. Guan, Y. Xu, H. Cheng, M. G. Li, C. S. Lee and X. Lu, High Open Circuit Voltage Over 1 V Achieved in Tin-Based Perovskite Solar Cells with a 2D/3D Vertical Heterojunction, Adv. Sci., 2022, 2200242 CrossRef CAS PubMed
.
- J. Chen, Y. Rong, A. Mei, Y. Xiong, T. Liu, Y. Sheng, P. Jiang, L. Hong, Y. Guan and X. Zhu, Hole-Conductor-Free Fully Printable Mesoscopic Solar Cell with Mixed-Anion Perovskite CH3NH3PbI(3−x)(BF4)x, Adv. Energy Mater., 2016, 6, 1502009 CrossRef
.
- H. Kim, J. Lim, M. Sohail and M. K. Nazeeruddin, Superhalogen Passivation for Efficient and Stable Perovskite Solar Cells, Sol. RRL, 2022, 2200013 CrossRef CAS
.
- B. Walker, G. H. Kim and J. Y. Kim, Pseudohalides in Lead-Based Perovskite Semiconductors, Adv. Mater., 2019, 31, 1807029 CrossRef PubMed
.
- F. A. Mautner, R. Cortés, L. Lezama and T. Rojo, [N(CH3)4][Mn(N3)3]: a Compound with a Distorted Perovskite Structure through Azido Ligands, Angew. Chem., Int. Ed. Engl., 1996, 35, 78–80 CrossRef
.
- H. Buser, D. Schwarzenbach, W. Petter and A. Ludi, The Crystal Structure of Prussian Blue: Fe4[Fe(CN)6]3·xH2O, Inorg. Chem., 1977, 16, 2704–2710 CrossRef CAS
.
- M.-L. Tong, J. Ru, Y.-M. Wu, X.-M. Chen, H.-C. Chang, K. Mochizuki and S. Kitagawa, Cation-Templated Construction of Three-Dimensional α-Po Cubic-Type [M(dca)3]− Networks. Syntheses, Structures and Magnetic Properties of A [M(dca)3](dca= dicyanamide; for A= benzyltributylammonium, M= Mn2+, Co2+; for A= benzyltriethylammonium, M= Mn2+, Fe2+), New J. Chem., 2003, 27, 779–782 RSC
.
- L. Duan, H. Zhang, M. Liu, M. Grätzel and J. Luo, Phase-Pure γ-CsPbI3 for Efficient Inorganic Perovskite Solar Cells, ACS Energy Lett., 2022, 7, 2911–2918 CrossRef CAS
.
- L. M. Castro-Castro and A. M. Guloy, Organic-Based Layered Perovskites of Mixed-Valent Gold (i)/Gold (iii) Iodides, Angew. Chem., Int. Ed., 2003, 115, 2877–2880 CrossRef
.
- W. Li, Z. Wang, F. Deschler, S. Gao, R. H. Friend and A. K. Cheetham, Chemically diverse and multifunctional hybrid organic–inorganic perovskites, Nat. Rev. Mater., 2017, 2, 1–18 Search PubMed
.
- Q. Tai, P. You, H. Sang, Z. Liu, C. Hu, H. L. Chan and F. Yan, Efficient and Stable Perovskite Solar Cells Prepared in Ambient Air Irrespective of the Humidity, Nat. Commun., 2016, 7, 1–8 Search PubMed
.
- A. M. Ganose, C. N. Savory and D. O. Scanlon, (CH3NH3)2Pb(SCN)2I2: A More Stable Structural Motif for Hybrid Halide Photovoltaics?, J. Phys. Chem. Lett., 2015, 6, 4594–4598 CrossRef CAS PubMed
.
- G. L. Gutsev and A. I. Boldyrev, The Electronic Structure of Superhalogens and Superalkalies, Russ. Chem. Rev., 1987, 56, 519–531 CrossRef
.
- S. Nagane, U. Bansode, O. Game, S. Chhatre and S. Ogale, CH3NH3PbI(3−x)(BF4)x: molecular ion substituted hybrid perovskite, Chem. Commun., 2014, 50, 9741–9744 RSC
.
- C. H. Hendon, R. X. Yang, L. A. Burton and A. Walsh, Assessment of Polyanion (BF4+ and PF6−) Substitutions in Hybrid Halide Perovskites, J. Mater. Chem. A, 2015, 3, 9067–9070 RSC
.
- A. Yang, D. Li, X. Lai, H. Zhang and C. Liang, Superhalogen Boron Tetrafluoride Surface Modification Reduces the Formation of Organic Cation Vacancies on the Surface of Halide Perovskite Films, J. Phys. Chem. C, 2021, 125, 21223–21233 CrossRef CAS
.
- D. Li, D. Li, H. Zhang, A. Yang and C. Liang, High-Performance Photovoltaic Materials Based on the Superlattice Structures of Organic–Inorganic Halide Perovskite and Superhalogen Hybrid Perovskite, J. Phys. Chem. Lett., 2020, 11, 5282–5294 CrossRef CAS PubMed
.
- C. W. F. T. Pistorius, Melting and Polymorphism of LiBH4 to 45 kbar, Z. Phys. Chem., 1974, 88, 253–263 CrossRef CAS
.
- S. Xu, G. Liu, H. Zheng, X. Xu, L. Zhang, H. Xu, L. Zhu, F. Kong, Y. Li and X. Pan, Boosting Photovoltaic Performance and Stability of Super-Halogen-Substituted Perovskite Solar Cells by Simultaneous Methylammonium Immobilization and Vacancy Compensation, ACS Appl. Mater. Interfaces, 2020, 12, 8249–8259 CrossRef CAS PubMed
.
- P. Schouwink, M. B. Ley, A. Tissot, H. Hagemann, T. R. Jensen, Ľ. Smrčok and R. Černý, Structure And Properties of Complex Hydride Perovskite Materials, Nat. Commun., 2014, 5, 5706 CrossRef CAS PubMed
.
- G. Balaji, P. H. Joshi, H. A. Abbas, L. Zhang, R. Kottokkaran, M. Samiee, M. Noack and V. L. Dalal, CH3NH3PbI3 from Non-Iodide Lead Salts for Perovskite Solar Cells via the Formation of PbI2, Phys. Chem. Chem. Phys., 2015, 17, 10369–10372 RSC
.
- Q. Jiang, D. Rebollar, J. Gong, E. L. Piacentino, C. Zheng and T. Xu, Pseudohalide-Induced Moisture Tolerance in Perovskite CH3NH3Pb(SCN)2I Thin Films, Angew. Chem., Int. Ed., 2015, 127, 7727–7730 CrossRef
.
- Y. Yu, C. Wang, C. R. Grice, N. Shrestha, D. Zhao, W. Liao, L. Guan, R. A. Awni, W. Meng, A. J. Cimaroli, K. Zhu, R. J. Ellingson and Y. Yan, Synergistic Effects of Lead Thiocyanate Additive and Solvent Annealing on the Performance of Wide-Bandgap Perovskite Solar Cells, ACS Energy Lett., 2017, 2, 1177–1182 CrossRef CAS
.
- Y. Lou, Y. Niu, D. Yang, Q. Xu, Y. Hu, Y. Shen, J. Ming, J. Chen, L. Zhang and Y. Zhao, Rod-shaped Thiocyanate-induced Abnormal Band Gap Broadening in SCN− Doped CsPbBr3 Perovskite Nanocrystals, Nano Res., 2018, 11, 2715–2723 CrossRef CAS
.
- Z. Xu, M. Chen and S. F. Liu, Origin of Enhanced Stability in Thiocyanate Substituted α-FAPbI3 Analogues, Sci. China: Chem., 2019, 62, 866–874 CrossRef CAS
.
- M. Rameez, E. Y.-R. Lin, P. Raghunath, S. Narra, D. Song, M.-C. Lin, C.-H. Hung and E. W.-G. Diau, Development of Hybrid Pseudohalide Tin Perovskites for Highly Stable Carbon-Electrode Solar Cells, ACS Appl. Mater. Interfaces, 2020, 12, 21739–21747 CrossRef CAS PubMed
.
- X. Liu, K.-L. Huang, G.-M. Liang, M.-S. Wang and G.-C. Guo, Molecular Design of Luminescent Halogeno-thiocyano-d10 Metal Complexes with In Situ Formation of the Thiocyanate Ligand, CrystEngComm, 2009, 11, 1615–1620 RSC
.
- F. Hao, C. C. Stoumpos, D. H. Cao, R. P. Chang and M. G. Kanatzidis, Lead-Free Solid-State Organic–Inorganic Halide Perovskite Solar Cells, Nat. Photonics, 2014, 8, 489–494 CrossRef CAS
.
- R. Younts, H.-S. Duan, B. Gautam, B. Saparov, J. Liu, C. Mongin, F. N. Castellano, D. B. Mitzi and K. Gundogdu, Efficient Generation of Long-Lived Triplet Excitons in 2D Hybrid Perovskite, Adv. Mater., 2017, 29, 1604278 CrossRef PubMed
.
- Y. Numata, Y. Sanehira, R. Ishikawa, H. Shirai and T. Miyasaka, Thiocyanate Containing Two-Dimensional Cesium Lead Iodide Perovskite, Cs2PbI2(SCN)2: Characterization, Photovoltaic Application, and Degradation Mechanism, ACS Appl. Mater. Interfaces, 2018, 10, 42363–42371 CrossRef CAS PubMed
.
- Y. Li, J. Shi, H. Wu, Y. Luo, D. Li and Q. Meng, Ultra-small Interlayer Spacing and Nano Channels in Anionic Layered Perovskite Cs2Pb(SCN)2I2 Enable Efficient Photoelectric Conversion, Sci. China Mater., 2021, 64, 61–72 CrossRef CAS
.
- H. Li, X. Jiang, Q. Wei, Z. Zang, M. Ma, F. Wang, W. Zhou and Z. Ning, Low-Dimensional Inorganic Tin Perovskite Solar Cells Prepared by Templated Growth, Angew. Chem., 2021, 133, 16466–16472 CrossRef
.
- Z. Xu, M. Chen and S. F. Liu, Pseudohalide Induced Tunable Electronic and Excitonic Properties in Two-dimensional Single-layer Perovskite for Photovoltaics and Photoelectronic Applications, J. Energy Chem., 2019, 36, 106–113 CrossRef
.
- A. T. Fafarman, W.-k Koh, B. T. Diroll, D. K. Kim, D.-K. Ko, S. J. Oh, X. Ye, V. Doan-Nguyen, M. R. Crump, D. C. Reifsnyder, C. B. Murray and C. R. Kagan, Thiocyanate-Capped Nanocrystal Colloids: Vibrational Reporter of Surface Chemistry and Solution-Based Route to Enhanced Coupling in Nanocrystal Solids, J. Am. Chem. Soc., 2011, 133, 15753–15761 CrossRef CAS PubMed
.
- S. Wang, C. Wu, H. Yao and F. Hao, Dimensional Tailoring Endows Tin Halide Perovskite Solar Cells with High Efficiency and Stability, Mater. Lab., 2022, 220047 Search PubMed
.
- N. D. Pham, V. T. Tiong, D. Yao, W. Martens, A. Guerrero, J. Bisquert and H. Wang, Guanidinium Thiocyanate Selective Ostwald Ripening Induced Large Grain for High Performance Perovskite Solar Cells, Nano Energy, 2017, 41, 476–487 CrossRef CAS
.
- J. Tong, Q. Jiang, A. J. Ferguson, A. F. Palmstrom, X. Wang, J. Hao, S. P. Dunfield, A. E. Louks, S. P. Harvey and C. Li, Carrier Control in Sn–Pb Perovskites via 2D Cation Engineering for All-Perovskite Tandem Solar Cells with Improved Efficiency and Stability, Nat. Energy, 2022, 7, 642–651 CrossRef CAS
.
- W. Zhang, X. Wu, Q. Cheng, B. Wang, S. U. Zafar, B. Han, J. Zhang, H. Zhang, X. Liu and Y. Zhang, Inhibiting the Growth of 1D Intermediates in Quasi-2D Ruddlesden–Popper Perovskites, Adv. Funct. Mater., 2022, 2206594 CrossRef CAS
.
- J. Yang, S. C. Cho, S. Lee, J. W. Yoon, W. H. Jeong, H. Song, J. T. Oh, S. G. Lim, S. Y. Bae, B. R. Lee, M. Ahmadi, E. H. Sargent, W. Yi, S. U. Lee and H. Choi, Guanidinium-Pseudohalide Perovskite Interfaces Enable Surface Reconstruction of Colloidal Quantum Dots for Efficient and Stable Photovoltaics, ACS Nano, 2022, 16, 1649–1660 CrossRef CAS PubMed
.
- A. T. Fafarman, W.-k Koh, B. T. Diroll, D. K. Kim, D.-K. Ko, S. J. Oh, X. Ye, V. Doan-Nguyen, M. R. Crump and D. C. Reifsnyder, Thiocyanate-Capped Nanocrystal Colloids: Vibrational Reporter of Surface Chemistry and Solution-based Route to Enhanced Coupling in Nanocrystal Solids, J. Am. Chem. Soc., 2011, 133, 15753–15761 CrossRef CAS PubMed
.
- B. Sun, O. Voznyy, H. Tan, P. Stadler, M. Liu, G. Walters, A. H. Proppe, M. Liu, J. Fan and T. Zhuang, Pseudohalide-Exchanged Quantum Dot Solids Achieve Record Auantum Efficiency in Infrared Photovoltaics, Adv. Mater., 2017, 29, 1700749 CrossRef PubMed
.
- H. Dong, Z. Wu, J. Xi, X. Xu, L. Zuo, T. Lei, X. Zhao, L. Zhang, X. Hou and A. K. Y. Jen, Pseudohalide-Induced Recrystallization Engineering For CH3NH3PbI3 Film And Its Application In Highly Efficient Inverted Planar Heterojunction Perovskite Solar Cells, Adv. Funct. Mater., 2018, 28, 1704836 CrossRef
.
- B. Yu, J. Shi, S. Tan, Y. Cui, W. Zhao, H. Wu, Y. Luo, D. Li and Q. Meng, Efficient (> 20%) and Stable All-Inorganic Cesium Lead Triiodide Solar Cell Enabled by Thiocyanate Molten Salts, Angew. Chem., Int. Ed., 2021, 60, 13436–13443 CrossRef CAS PubMed
.
- B. A. Koscher, J. K. Swabeck, N. D. Bronstein and A. P. Alivisatos, Essentially Trap-Free CsPbBr3 Colloidal Nanocrystals By Postsynthetic Thiocyanate Surface Treatment, J. Am. Chem. Soc., 2017, 139, 6566–6569 CrossRef CAS PubMed
.
- J. T. Lin, Y. K. Hu, C. H. Hou, C. C. Liao, W. T. Chuang, C. W. Chiu, M. K. Tsai, J. J. Shyue and P. T. Chou, Superior Stability and Emission Quantum Yield (23% ± 3%) of Single-Layer 2D Tin Perovskite TEA2SnI4 via Thiocyanate Passivation, Small, 2020, 16, 2000903 CrossRef CAS PubMed
.
- X. Zhang, G. Wu, W. Fu, M. Qin, W. Yang, J. Yan, Z. Zhang, X. Lu and H. Chen, Orientation Regulation of Phenylethylammonium Cation based 2D Perovskite Solar Cell with Efficiency Higher than 11%, Adv. Energy Mater., 2018, 8, 1702498 CrossRef
.
- W. Li, M. U. Rothmann, Y. Zhu, W. Chen, C. Yang, Y. Yuan, Y. Y. Choo, X. Wen, Y.-B. Cheng and U. Bach, The Critical Role of Composition-Dependent Intragrain Planar Defects in The Performance of MA1–xFAxPbI3 Perovskite Solar Cells, Nat. Energy, 2021, 6, 624–632 CrossRef CAS
.
- X. Lian, J. Chen, Y. Zhang, M. Qin, J. Li, S. Tian, W. Yang, X. Lu, G. Wu and H. Chen, Highly Efficient Sn/Pb Binary Perovskite Solar Cell via Precursor Engineering: A Two-Step Fabrication Process, Adv. Funct. Mater., 2019, 29, 1807024 CrossRef
.
- W. Zhang, X. Wu, J. Zhou, B. Han, X. Liu, Y. Zhang and H. Zhou, Pseudohalide-Assisted Growth of Oriented Large Grains for High-Performance and Stable 2D Perovskite Solar Cells, ACS Energy Lett., 2022, 7, 1842–1849 CrossRef CAS
.
- H. Lu, Y. Liu, P. Ahlawat, A. Mishra, W. R. Tress, F. T. Eickemeyer, Y. Yang, F. Fu, Z. Wang and C. E. Avalos, Vapor-Assisted Deposition of Highly Efficient, Stable Black-Phase FAPbI3 Perovskite Solar Cells, Science, 2020, 370, eabb8985 CrossRef CAS PubMed
.
- D. Kim, H. J. Jung, I. J. Park, B. W. Larson, S. P. Dunfield, C. Xiao, J. Kim, J. Tong, P. Boonmongkolras and S. G. Ji, Efficient, Stable Silicon Tandem Cells Enabled by Anion-Engineered Wide-Bandgap Perovskites, Science, 2020, 368, 155–160 CrossRef CAS PubMed
.
- D. B. Khadka, Y. Shirai, M. Yanagida and K. Miyano, Pseudohalide Functional Additives in Tin Halide Perovskite for Efficient and Stable Pb-Free Perovskite Solar Cells, ACS Appl. Energy Mater., 2021, 4, 12819–12826 CrossRef CAS
.
- D. Yu, Q. Wei, H. Li, J. Xie, X. Jiang, T. Pan, H. Wang, M. Pan, W. Zhou, W. Liu, P. C. Y. Chow and Z. Ning, Quasi-2D Bilayer Surface Passivation for High Efficiency Narrow Bandgap Perovskite Solar Cells, Angew. Chem., Int. Ed., 2022, 61, e202202346 CAS
.
- N. Yarita, H. Tahara, M. Saruyama, T. Kawawaki, R. Sato, T. Teranishi and Y. Kanemitsu, Impact of Postsynthetic Surface Modification on Photoluminescence Intermittency in Formamidinium Lead Bromide Perovskite Nanocrystals, J. Phys. Chem. Lett., 2017, 8, 6041–6047 CrossRef CAS PubMed
.
- F. Xu, J. Liu, A. S. Subbiah, W. Liu, J. Kang, G. T. Harrison, X. Yang, F. H. Isikgor, E. Aydin, M. De Bastiani and S. De Wolf, Potassium Thiocyanate-Assisted Enhancement of Slot-Die-Coated Perovskite Films for High-Performance Solar Cells, Small Sci., 2021, 1, 2000044 CrossRef CAS
.
- C. Zheng, C. Bi, F. Huang, D. Binks and J. Tian, Stable and Strong Emission CsPbBr3 Quantum Dots by Surface Engineering for High-Performance Optoelectronic Films, ACS Appl. Mater. Interfaces, 2019, 11, 25410–25416 CrossRef CAS PubMed
.
- J.-J. Cao, Y.-H. Lou, W.-F. Yang, K.-L. Wang, Z.-H. Su, J. Chen, C.-H. Chen, C. Dong, X.-Y. Gao and Z.-K. Wang, Multifunctional Potassium Thiocyanate Interlayer for Eco-Friendly Tin Perovskite Indoor and Outdoor Photovoltaics, Chem. Eng. J., 2022, 433, 133832 CrossRef CAS
.
- Q. Chen, J. Wu, J. T. Matondo, L. Bai, D. M. Maurice and M. Guli, Optimization of Bulk Defects in Sn/Pb Mixed Perovskite Solar Cells Through Synergistic Effect of Potassium Thiocyanate, Sol. RRL, 2020, 4, 2000584 CrossRef CAS
.
- Y. Su, J. Yang, G. Liu, W. Sheng, J. Zhang, Y. Zhong, L. Tan and Y. Chen, Acetic Acid-Assisted Synergistic Modulation of Crystallization Kinetics and Inhibition of Sn2+ Oxidation in Tin-Based Perovskite Solar Cells, Adv. Funct. Mater., 2022, 32, 2109631 CrossRef CAS
.
- N. K. Noel, M. Congiu, A. J. Ramadan, S. Fearn, D. P. McMeekin, J. B. Patel, M. B. Johnston, B. Wenger and H. J. Snaith, Unveiling The Influence of pH on The Crystallization of Hybrid Perovskites, Delivering Low Voltage Loss Photovoltaics, Joule, 2017, 1, 328–343 CrossRef CAS
.
- P. K. Nayak, D. T. Moore, B. Wenger, S. Nayak, A. A. Haghighirad, A. Fineberg, N. K. Noel, O. G. Reid, G. Rumbles and P. Kukura, Mechanism For Rapid Growth of Organic–Inorganic Halide Perovskite Crystals, Nat. Commun., 2016, 7, 1–8 Search PubMed
.
- L. Meng, Q. Wei, Z. Yang, D. Yang, J. Feng, X. Ren, Y. Liu and S. Liu, Improved Perovskite Solar Cell Efficiency by Tuning The Colloidal Size and Free Ion Concentration in Precursor Solution Using Formic Acid Additive, J. Energy Chem., 2020, 41, 43–51 CrossRef
.
- Y. Liu, Y. Zhang, X. Zhu, Z. Yang, W. Ke, J. Feng, X. Ren, K. Zhao, M. Liu and M. G. Kanatzidis, Inch-Sized High-Quality Perovskite Single Crystals by Suppressing Phase Segregation for Light-Powered Integrated Circuits, Sci. Adv., 2021, 7, eabc8844 CrossRef CAS PubMed
.
- X. Meng, T. Wu, X. Liu, X. He, T. Noda, Y. Wang, H. Segawa and L. Han, Highly Reproducible and Efficient FASnI3 Perovskite Solar Cells Fabricated with Volatilizable Reducing Solvent, J. Phys. Chem. Lett., 2020, 11, 2965–2971 CrossRef CAS PubMed
.
- A. Wang, X. Deng, J. Wang, S. Wang, X. Niu, F. Hao and L. Ding, Ionic Liquid Reducing Energy Loss and Stabilizing CsPbI2Br Solar Cells, Nano Energy, 2021, 81, 105631 CrossRef CAS
.
- Y. Chen, N. Li, L. Wang, L. Li, Z. Xu, H. Jiao, P. Liu, C. Zhu, H. Zai and M. Sun, Impacts of Alkaline on The Defects Property and Crystallization Kinetics in Perovskite Solar Cells, Nat. Commun., 2019, 10, 1–10 CrossRef PubMed
.
- S. Lv, W. Gao, G. Xing, L. Chao, L. Song, M. Li, L. Fu, Y. Chen and C. Ran, Improving the Air Resistance of the Precursor Solution for Ambient-Air Coating of an Sn–Pb Perovskite Film with Superior Photovoltaic Performance, ACS Appl. Mater. Interfaces, 2022, 14, 43362–43371 CrossRef CAS PubMed
.
- Y. Cui, J. Shi, F. Meng, B. Yu, S. Tan, S. He, C. Tan, Y. Li, H. Wu, Y. Luo, D. Li and Q. Meng, A Versatile Molten-Salt Induction Strategy to Achieve Efficient CsPbI3 Perovskite Solar Cells with a High Open-Circuit Voltage >1.2 V, Adv. Mater., 2022, 2205028 CrossRef CAS PubMed
.
- C. Liang, H. Gu, Y. Xia, Z. Wang, X. Liu, J. Xia, S. Zuo, Y. Hu, X. Gao and W. Hui, Two-dimensional Ruddlesden–Popper layered Perovskite Solar Cells Based on Phase-Pure Thin Films, Nat. Energy, 2021, 6, 38–45 CrossRef CAS
.
- S. Nagane, U. Bansode, O. Game, S. Chhatre and S. Ogale, CH3NH3PbI(3−x)(BF4)x: Molecular Ion Substituted Hybrid Perovskite, Chem. Commun., 2014, 50, 9741–9744 RSC
.
- J. Zhang, S. Wu, T. Liu, Z. Zhu and A. K. Y. Jen, Boosting Photovoltaic Performance for Lead Halide Perovskites Solar Cells with BF4− anion Substitutions, Adv. Funct. Mater., 2019, 29, 1808833 CrossRef CAS
.
- H. Zhang, S. Xu, T. Guo, D. Du, Y. Tao, L. Zhang, G. Liu, X. Chen, J. Ye, Z. Guo and H. Zheng, Dual Effect of Superhalogen Ionic Liquids Ensures Efficient Carrier Transport for Highly Efficient and Stable Perovskite Solar Cells, ACS Appl. Mater. Interfaces, 2022, 14, 28826–28833 CrossRef CAS PubMed
.
- S. Nagane, S. Macpherson, M. A. Hope, D. J. Kubicki, W. Li, S. D. Verma, J. Ferrer Orri, Y.-H. Chiang, J. L. MacManus-Driscoll, C. P. Grey and S. D. Stranks, Tetrafluoroborate-Induced Reduction in Defect Density in Hybrid Perovskites through Halide Management, Adv. Mater., 2021, 33, 2102462 CrossRef CAS PubMed
.
- H. Zhang, Z. Ren, K. Liu, M. Qin, Z. Wu, D. Shen, Y. Zhang, H. T. Chandran, J. Hao, C.-S. Lee, X. Lu, Z. Zheng, J. Huang and G. Li, Controllable Heterogenous Seeding-Induced Crystallization for High-Efficiency FAPbI3-Based Perovskite Solar Cells Over 24%, Adv. Mater., 2022, 34, 2204366 CrossRef CAS PubMed
.
- H. Zhang, Z. Ren, K. Liu, M. Qin, Z. Wu, D. Shen, Y. Zhang, H. T. Chandran, J. Hao and C. S. Lee, Controllable Heterogenous Seeding-Induced Crystallization for High-Efficiency FAPbI3-Based Perovskite Solar Cells Over 24%, Adv. Mater., 2022, 34, 2204366 CrossRef CAS PubMed
.
- Y.-H. Lin, N. Sakai, P. Da, J. Wu, H. C. Sansom, A. J. Ramadan, S. Mahesh, J. Liu, R. D. J. Oliver, J. Lim, L. Aspitarte, K. Sharma, P. K. Madhu, A. B. Morales-Vilches, P. K. Nayak, S. Bai, F. Gao, C. R. M. Grovenor, M. B. Johnston, J. G. Labram, J. R. Durrant, J. M. Ball, B. Wenger, B. Stannowski and H. J. Snaith, A Piperidinium Salt Stabilizes Efficient Metal-Halide Perovskite Solar Cells, Science, 2020, 369, 96–102 CrossRef CAS PubMed
.
- G. L. Gutsev and A. I. Boldyrev, DVM-Xα Calculations on The Ionization Potentials of MXk+1− Complex Anions and The Electron Affinities of MXk+1 “superhalogens”, Chem. Phys., 1981, 56, 277–283 CrossRef CAS
.
- H. Fang and P. Jena, Super-Ion Inspired Colorful Hybrid Perovskite Solar Cells, J. Mater. Chem. A, 2016, 4, 4728–4737 RSC
.
- J. Sanchez-Diaz, R. S. Sánchez, S. Masi, M. Kreĉmarová, A. O. Alvarez, E. M. Barea, J. Rodriguez-Romero, V. S. Chirvony, J. F. Sánchez-Royo, J. P. Martinez-Pastor and I. Mora-Seró, Tin Perovskite Solar Cells with >1,300 h of Operational Stability in N2 through A Synergistic Chemical Engineering Approach, Joule, 2022, 6, 861–883 CrossRef CAS PubMed
.
- J. Tao, X. Liu, J. Shen, S. Han, L. Guan, G. Fu, D.-B. Kuang and S. Yang, F-Type Pseudo-Halide Anions for High-Efficiency and Stable Wide-Band-Gap Inverted Perovskite Solar Cells with Fill Factor Exceeding 84%, ACS Nano, 2022, 16, 10798–10810 CrossRef CAS PubMed
.
- T. Bu, J. Li, H. Li, C. Tian, J. Su, G. Tong, L. K. Ono, C. Wang, Z. Lin and N. Chai, Lead Halide–Templated Crystallization of Methylamine-Free Perovskite for Efficient Photovoltaic Modules, Science, 2021, 372, 1327–1332 CrossRef CAS PubMed
.
|
This journal is © the Partner Organisations 2023 |