DOI:
10.1039/D2QM01035F
(Research Article)
Mater. Chem. Front., 2023,
7, 160-167
Isolation strategy for a novel luminescent Eu3+-pyridine-2,6-dicarboxylic acid complex with high compatibility and stability for light-conversion agricultural films†
Received
10th October 2022
, Accepted 16th November 2022
First published on 16th November 2022
Abstract
Small organic conjugated molecule-based rare earth complexes are a kind of promising light-conversion material used in greenhouse films to improve the crop yield. Unfortunately, achieving a high luminescent performance of this kind of light-conversion material with a low migration rate in the film is still a big challenge. It has been predicted that increasing the interaction between the luminescent complex and polymer backbone could reduce the migration rate of the complex. Herein, we bridged two pyridine-2,6-dicarboxylic acid derivatives coordinated to Eu3+ luminescent centers using different lengths of the alkyl chain. Then, two new light-conversion agents, LDPA-4 (four carbon atoms) and LDPA-6 (six carbon atoms), were prepared. Using this strategy, we achieved a 615 nm red-light emission quantum yield (QY) of 13.16% for LDPA-6, which was much higher than that of LDPA-4 (5.28%) and the reference sample LCA-C (10.77%), respectively. In the resulting light-conversion films, rape samples treated with LDPF-6 exhibited the largest growth rate and yield of 2482.8 kg ha−1 during the experimental plot application, which was 1.15 times higher than that of the pure PE one, demonstrating the special ligand effect of the pyridine-2,6-dicarboxylic acid and the importance of the alkyl chain length between the two luminescent centers.
Introduction
The utilization of light-conversion greenhouse films in agricultural activities is a promising way to protect plants and increase crop yield because the harmful ultraviolet and/or useless yellow-green light could be converted to blue (400–480 nm) or red light (600–700 nm), which are two useful band lights for the photosynthesis of plants.1 In such a light-conversion film, the light-conversion agent is the core component. Therefore, great efforts have been devoted to developing high-performance light-conversion agents to meet the request of practical applications over the past few decades.2 In general, light-conversion agents can be classified into three types: organic chromophores,3 inorganic rare earth complexes,4 and organic rare earth complexes.5 Each type of agent has its own character. For instance, organic chromophores possess the characteristic of a broad range of excitation and emission spectra, a variable molecular structure, and high brightness while requiring elaborate synthetic procedures,6,7 while inorganic rare earth complexes have sharp emission, and large Stokes shift properties but a weak absorption coefficient.8 By comparison, organic rare earth complexes are considered attractive luminescent materials, especially organic chromophore-coordinated lanthanide complexes, which offer more advantages over the other two because of their long lifetimes (in the ms range), high brightness, good light color uniformity, and good photothermal stability.5 Specifically, luminescent lanthanide complexes are interesting compounds that are composed of a lanthanide cation coordinated with an organic chromophore ligand. In this coordinating system, the organic chromophore ligands act as photosensitizers to harvest light and transfer the T1 state energy to the 4f–4f transition bands of the lanthanide ion(III).9–11 For a certain lanthanide complex, the excitation wavelength and emission intensity could be precisely adjusted by rational design of the chromophore ligand.12,13 In the family of lanthanide complexes, Eu3+-β-diketonate complexes, have attracted considerable attention owing to their ultraviolet (UV) light absorption, red emission property, and chemical stability.14–17 In other words, Eu3+-β-diketonate complexes and their derivatives are good candidates for light-conversion agents. Indeed, many highly efficient Eu3+-based complexes have been prepared and used as light-conversion agents.18–21 Even so, the potential light-conversion ability of Eu3+-based complexes is not fully developed. There are still some challenges for Eu3+-based complexes on the road to greenhouse-film practical applications, such as moderate fluorescence quantum efficiency, low lifetime, low photostability, and poor compatibility.
After decades of research, the fluorescence quantum efficiency and lifetime of Eu3+-based complexes have been well investigated by the rational design of their ligand types, stereostructure, and coordinating numbers, with numerous excellent works in the literature.10,22–24 Nevertheless, scant attention has been paid to the photothermal stability and compatibility of Eu3+-based complexes in greenhouse films.25 As is well-known, the poor compatibility of Eu3+-based complexes in polymers leads to poor dispersibility. Moreover, the uniformity of Eu3+-based complexes affects the mechanical properties and transparency of the polymer.26 To solve this problem, the rational modification of the periphery of Eu3+-based complexes is necessary. Significantly, the isolation group strategy has been successfully used to minimize the strong intermolecular electrostatic dipole–dipole interactions among chromophores in the field of nonlinear optical materials.27–29 In this strategy, bulky groups are introduced to the skeleton of the chromophore, or two chromophores are connected by a flexible alkyl chain to increase their steric hindrance. The research results so far have indicated that the orientation of incorporated chromophores can be well aligned with a minimum molecular migration during the device fabrication. Moreover, the alkyl chain in the guest molecule improves its compatibility in the host matrix. Inspired by this, we assume that this effective strategy could be used to prepare novel Eu3+-based complex light-conversion agents and reduce their migration rate.
Herein, two new ligands, BDPA-4 and BDPA-6, were synthesized by linking two pyridine-2,6-dicarboxylic acid derivatives with different lengths of alkyl chains. By using the newly prepared ligands, together with the ligands pyridine-2,6 dicarboxylic acid (PA) and thenoyltrifluoroacetone (TTA), two new Eu3+-based light-conversion agents, LDPA-4 and LDPA-6, were prepared. The literature-reported agent LCA-C was also synthesized for comparison.16 The molecular structures of the compounds are reported in Scheme 1. The photophysical properties of LCA-C, LDPA-4, and LDPA-6 were studied and it was found that all of them exhibited the highest intensity emission peak at 615 nm. The largest quantum yield (QY) was obtained on LDPA-6 (13.16%), followed by LCA-C (10.77%), and then LDPA-4 (5.28%). In the resulting films, the agents LDPA-4 and LDPA-6 with alkyl chains had reduced mobility in the polymer matrix when compared with the LCA-C one, allowing them to stably exist in the film for a long time even under the polar solvent environment. Combining the high red-light emission efficiency and high stability of LDPA-6 in the film, the LDPF-6-film-treated rapes achieved an enhanced growth rate and yield when compared with the pure PE film. In this study, the introduction of an alkyl chain in the light-conversion agents not only improved their compatibility but also had a significant effect on their quantum efficiency in the film. This work provides a new avenue to modify luminescent complexes to improve their stability and light-conversion efficiency. In the future, more attractive light-conversion agents could be developed based on this strategy.
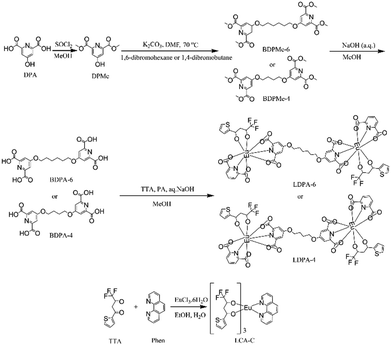 |
| Scheme 1 Synthetic route of the agents LCF-C, LDPA-4, and LDPA-6, respectively. | |
Experimental section
Regents and equipment
All chemicals were commercially available unless otherwise mentioned. Europium chloride hexahydrate (EuCl3·6H2O, 99.99%), thionyl chloride (SOCl2), chelidamic acid monohydrate (DPA), potassium carbonate (K2CO3), sodium hydroxide (NaOH), 1,4-dibromobutane, 1,6-dibromohexane, pyridine-2,6 dicarboxylic acid (PA), Hydrochloric acid (HCl), N,N-dimethylformamide (DMF), methanol (MeOH), ethanol (EtOH), dichloromethane (DCM), 2-thenoyltrifluoroacetone (TTA), and 1,10-phenanthroline (Phen) were purchased from Shanghai Macklin Biochemical Co., Ltd. LDPE (Low-density polyethylene) 100AC was purchased from Beijing Yanshan Petrochemical Co., Ltd. LLDPE (Linear low-density polyethylene) 9047 and LDPE 2420D were purchased from Daqing Petrochemical Co., Ltd. Anti-aging and anti-dripping (AA) was purchased from Shandong Baiyun Plastic Co., Ltd. Vertical mixer WSQB-200 was purchased from Zhejiang Hainai Machinery Technology Co., Ltd. The double screw extruder LTE26-44 and pelletizer 1604-120/VS were purchased from Us Lab Tech. The multifunctional plastic extrusion unit 120 was purchased from Laiwu Xinfu Plastic Machine Co., Ltd.
Synthesis of Eu(TTA)3Phen (LCA-C)
Eu(TTA)3Phen (denoted as LCA-C) was synthesized by the conventional precipitation method. Typically, to a solution of Phen·H2O (14.0 g, 71 mmol, 1.0 equiv.) and TTA (47.4 g, 213 mmol, 3.0 equiv.) in 80 mL ethanol was added a 70 °C solution of EuCl3·6H2O (26.0 g, 71 mmol, 1.0 equiv.) in 60 mL deionized water. Then the mixed solution was stirred at 80 °C until no more precipitate was formed. The obtained precipitate was collected by filtering and then washed with deionized water and anhydrous ethanol several times. Then the filtrate was dried at 60 °C for 12 h in a vacuum oven. Finally, 60 g of LCA-C was obtained as a light-yellow solid.
Synthesis of dimethyl 4-hydroxypyridine-2,6-dicarboxylate (DPMe)
To a 1 L two-neck round-bottom flask was added (52.8 g, 262.0 mmol, 1 equiv.) of chelidamic acid monohydrate and MeOH (500 mL); to the above suspension was slowly added SOCl2 (95.0 mL, 1.30 mol, 5 equiv.) using a syringe at 0 °C. Then, the reaction mixture was stirred at room temperature for 24 h, followed by refluxing for another 2 h. The solvent was removed under reduced pressure at room temperature, and the residue was neutralized with cold 1 M K2CO3 solution. The precipitate was filtered by vacuum filtration and washed with 50% MeOH water solution several times. The brown filtrate was dried under reduced pressure to give a brown solid (55.4 g, 92%). 1H NMR (400 MHz, (CD3)2SO): δ 7.49 (s, 2H), 3.85 (s, 6H), (Fig. S1 of the ESI†). 13C NMR (100 MHz, (CD3)2SO): δ 165.8, 149.3, 116.8, 52.9 (Fig. S2 of the ESI†). HRMS: calcd for [C9H9NO5]+ 212.0261; found [M + K]+ 250.0110 (Fig. S3 of the ESI†).
Synthesis of BDPMe-4 and BDPMe-6
To a 500 mL two-neck round-bottom flask was added a mixture of dimethyl 4-hydroxypyridine-2,6-dicarboxylate (20 g, 94.8 mmol, 2.0 equiv.), 1,4-dibromobutane (10.2 g, 47.4 mmol, 1.0 equiv.), K2CO3 (19.6 g, 142.2 mmol, 3.0 equiv.), and DMF (200 mL). Then, the reaction mixture was reacted at 80 °C under a N2 atmosphere for 12 h. After that, the reaction mixture was poured into 500 mL water and a white precipitate was formed. The resulting white precipitate was collected by filtration and washed with water three times. Then, the precipitate was redissolved in 250 mL CH2Cl2 and washed with a 150 mL aqueous NaOH solution (5 wt%) twice. The organic phase was concentrated by rotary evaporation and dried under vacuum to give BDPMe-4 as a brown solid in an 80% yield. 1H NMR (400 MHz, CDCl3): δ 7.81 (s, 4H), 4.23 (t, J = 8.0 Hz, 4H), 4.01 (s, 12H), 2.08–2.06 (m, 4H) (Fig. S4 of the ESI†). 13C NMR (100 MHz, CDCl3): δ 166.9, 165.2, 149.9, 114.5, 68.3, 53.3, 25.4 (Fig. S5 of the ESI†). HRMS [M + Na]+ calcd for C26H33N2O10 + 499.1224; found: 499.1322 (Fig. S6 of the ESI†); Replacing 1,4-dibromobutane with 1,6-dibromobutane, BDPMe-6 was synthesized in a similar way as for BDPMe-4 and obtained as a white solid in an 80% yield. 1H NMR (400 MHz, CDCl3): δ 7.80 (s, 4H), 4.15 (t, J = 8.0 Hz, 4H), 4.00 (s, 12H), 1.92–1.86 (m, 4H), 1.59–1.55 (m, 4H) (Fig. S7 of the ESI†). 13C NMR (100 MHz, CDCl3): δ 167.0, 165.2, 149.8, 114.5, 68.8, 53.3, 28.7, 25.6 (Fig. S8 of the ESI†). HRMS [M + Na]+ calcd for C26H33N2O10+ 504.1097; found: 527.1637 (Fig. S9 of the ESI†).
Synthesis of BDPA-4 and BDPA-6
To a 250 mL round-bottom flask was added a mixture of BDPMe-4 (5 g, 10.5 mmol, 1.0 equiv.), NaOH (2.1 g, 52.5 mmol, 5.0 equiv.), methanol (50 mL), and water (50 mL). The reaction mixture was stirred at 60 °C for 12 h and then acidified with 3 M HCl to pH 4. Then, the obtained precipitate was collected by centrifugation, washed with H2O, and dried under vacuum to give BDPA-4 as a brown solid in a 60% yield. 1H NMR (400 MHz, D2O): δ 7.50 (s, 4H), 4.25 (s, 4H), 2.00 (s, 4H) (Fig. S10 of the ESI†). 13C NMR (100 Hz, D2O): δ 173.2, 165.4, 155.0, 111.9, 68.9, 25.4 (Fig. S11 of the ESI†). Anal. Cald. for C18H16N2O10: C, 51.44; H, 3.84; N, 6.66; Found: C, 51.26; H, 3.83; N, 6.55. BDPA-6 was prepared in a similar way as BDPA-4 and obtained as a white solid in a 60% yield. 1H NMR (400 MHz, D2O): δ 7.45 (s, 4H), 4.13 (t, J = 8.0 Hz, 4H), 1.77 (t, J = 8.0 Hz, 4H), 1.48–1.44 (m, 4H) (Fig. S12 of the ESI†). 13C NMR (100 Hz, D2O): δ 172.7, 166.9, 154.6, 111.4, 68.7, 28.0, 24.7 (Fig. S13 of the ESI†). Anal. Calcd. for C20H20N2O10: C, 53.57; H, 4.50; N, 6.25; Found: C, 53.19; H, 4.72; N, 6.54.
Synthesis of LDPA-4 and LDPA-6
To a solution of BDPA-4 (35.0 g, 83.3 mmol, 1.0 equiv.), pyridine-2,6-dicarboxylic acid (27.8 g, 166.7 mmol, 2.0 equiv.), TTA (37.0 g, 166.7 mmol, 2.0 equiv.), and NaOH (26.7 g, 666.7 mmol, 8.0 equiv.) in 250 mL water was added a solution of europium chloride hexahydrate (61.0 g, 166.7 mmol, 2.0 equiv.) in 100 mL water, and then the mixture was stirred at room temperature for 2 h. The obtained precipitate was collected by filtering and washed with water several times, and dried under vacuum to give BDPA-4 as a brown solid. By using BDPA-6, LDPA-6 was prepared in a similar way and obtained as a white solid.
Characterization
Liquid-state 1H NMR and 13C NMR spectra were collected using an Advance Bruker III 400 M (400 MHz) instrument with tetramethylsilane (TMS) as an internal standard. Elemental analysis was carried out on an Elementar Vario El cube analyzer. Fourier transform infrared (FT-IR) spectroscopy was performed on a Nicolet IS10 FT-IR spectrometer in the 400–4000 cm−1 range using the KBr disk method. UV-Vis absorption experiments were performed on a UV-2550 UV-vis spectrometer in the 200–800 nm range and at a resolution of 1 nm. Fluorescence spectra were recorded using an RF-5301 fluorescence spectrometer, equipped with a Xe flash lamp as the excitation source and a slit width of ∼5 nm. The absolute QY was verified using an FLS1000 fluorescence spectrometer with an excitation wavelength (λ) of 348 nm and an emission range of 330–750 nm. X-Ray diffraction (XRD) patterns were recorded on an Enraf Nonius CAD4 instrument with a monochromator Cu Kα source operated at 40 kV and 30 mA for single-crystal analysis.
Results and discussion
FT-IR analysis of LCA-C, LDPA-4, and LDPA-6
To further confirm the chemical structures of the prepared ligands and light-converting agents, FT-IR spectroscopy was employed. As shown in Fig. S14 (ESI†), the agent LCA-C exhibited peaks at 1626 and 1571 cm−1 belonging to the stretching vibration peak of the C
O group of the TTA ligand, while peaks at 1602 and 1538 cm−1 were also observed and originated from the stretching vibration peaks of C
C bond and C
N bond of the Phen ligand, indicating that the Phen and TTA ligands were successful coordinated with the Eu3+.16 In the newly prepared ligands BDPA-4 and BDPA-6, the wide peak band at 3200–2500 cm−1 and peak at 1720 cm−1 were attributed to the scattered O–H stretching vibration and the C
O stretching vibration, respectively. Compared with BDPA-4 and BDPA-6, the scattered O–H stretching vibration peak was found to disappear and the C
O stretching vibration peak was blue-shifted to 1615 cm−1 in the spectra of LDPA-4 and LDPA-6, confirming the formation of Eu–O bond, and therein the successful synthesis of LDPA-4 and LDPA-6.30
Photophysical properties of LCA-C, LDPA-4, and LDPA-6
To investigate the photophysical properties of the obtained three light-conversion agents, ethanol solutions of LCA-C (1 × 10−5 mol L−1), LDPA-4 (0.5 × 10−5 mol L−1), and LDPA-6 (0.5 × 10−5 mol L−1) were prepared and analyzed by UV-vis spectroscopy. As shown in Fig. 1a, compounds LCA-C, LDPA-4, and LDPA-6 exhibited two identical UV absorption bands at 250–290 nm and 310–370 nm, respectively, which were attributed to π–π* transitions of the aromatic groups in the ligand.31,32 These results indicated that the three light-conversion agents could effectively absorb multi-band UV lights. Thereafter, the excitation spectra and fluorescence emission spectra of LCA-C, LDPA-4, and LDPA-6 were investigated. As shown in Fig. 1b (left side), the maximum excitation wavelength of LCA-C was determined to be 381 nm. For LDPA-4 and LDPA-6, both had two similar maximum excitation wavelengths, which were observed at 282 nm and 364 nm, respectively. In these three complexes, their excitation spectra of the ligand overlapped well with the UV-light-absorption spectra, indicating that these light-conversion agents are good candidates for converting UV light to long wavelength light.32 As expected, the agents LCA-C, LDPA-4, and LDPA-6 exhibited efficient characteristic Eu3+ emission peaks at 580, 593, 615, 653, and 700 nm at 370 nm excitation, corresponding to the 4f transition of Eu3+ (5d0 → 7fi, i = 0–4).32–34 The highest intensity emission peak was observed at 615 nm, which was attributed to the 5D0 → 7f2 electric-dipole (ED) transition.32
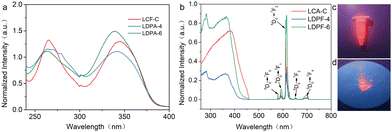 |
| Fig. 1 (a) Normalized UV-vis absorption spectra of LCA-C, LDPA-4, and LDPA-6, respectively. (b) Normalized excitation (λem = 615 nm) and emission (λex = 370 nm) spectra of LCA-C, LDPA-4 and LDPA-6, respectively. Digital photos of LDPA-6 (c) in an aqueous solution and (d) in the solid state at the excitation of 375 nm. | |
Based on the emission spectra, the absolute fluorescence QYs of LCA-C, LDPA-4, and LDPA-6 were determined to be 10.77%, 5.28%, and 13.16%, respectively. As is known, a high QY of a luminescent molecule would originate from the strong interaction between the rare earth ion and ligands and a high ratio of radiative/nonradiative transition.34 Here, LDPA-6 showed higher QYs than LCA-C, which could be attributed to the following: (1) a more rigid molecular structure was formed when using pyridine-2,6-dicarboxylic acid derivative as the ligand, which reduces the nonradiative transition; (2) the pyridine-2,6-dicarboxylic acid derivative acted as an antenna, which increased the energy transfer between the ligands; (3) there was a more effective electronic interaction between Eu3+ and the pyridine-2,6-dicarboxylic acid derivative ligand. Fig. 1c and d are the digital photos of LDPA-6 in an aqueous solution and in the solid state at the excitation of 375 nm, respectively, indicating its good red-light emission properties. It was noteworthy that even though LDPA-4 and LDPA-6 had the same luminescent centers, LDPA-4 exhibited a much smaller QY than LDPA-6. Considering their molecular structure, the smaller QY of LDPA-4 could be attributed to aggregation-caused quenching because of the shorter alkyl chain between the two emission centers. This indicates that the length of the alkyl chain was critical for the QY of our two luminescent center systems.
Photophysical properties of the light-conversion films LCF-C, LDPF-4, and LDPF-6
With these three light-conversion agents in hand, three different light-conversion films were prepared (The details are provided in the ESI†), which were denoted as LCF-C, LDPF-4, and LDPF-6, respectively. Their UV-vis absorption and transmission spectra were collected and compared with the reference pure PE. As shown in Fig. 2a, the reference pure PE only exhibited a weak absorption peak at around 275 nm and almost no absorption in the range of 300–400 nm, while the three light-conversion films all exhibited broad absorption in the ultraviolet range of 250–400 nm. Among the three films, the LDPF-6 film had the highest absorption intensity compared to the other two, indicating that LDPF-6 could absorb most of the UV light. To verify this, the transmittance property of LCF-C, LDPF-4, and LDPF-6 films was also studied. As shown in Fig. 2b, pure PE showed the highest transmittance of 65% in the range of 250–425 nm. In comparison, the UV transmittance of the LCF-C, LDPF-4, and LDPF-6 films dropped to 51.1%, 55.5%, and 38.2%, respectively. Obviously, the LDPF-6 film had the highest UV blocking ability, coincident with its UV absorption results. In addition, there was no obvious difference in transmittance in the visible-light region between the three light-conversion films and pure PE film, indicating that the visible light would not be affected by introducing a suitable amount of light-conversion agent.
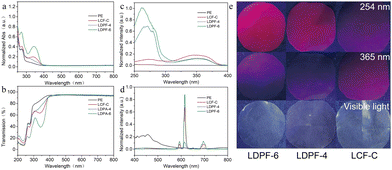 |
| Fig. 2 (a) Absorption and (b) transmittance spectra of the PE, LCF-C, LDPF-4, and LDPF-6 films, respectively. (c) Excitation absorption spectra of the PE, LCF-C, LDPF-4, and LDPF-6 films, respectively, λem = 615 nm. (d) Emission spectra of the PE, LCF-C, LDPF-4, and LDPF-6 films, respectively, λex = 370 nm. (e) Top row: digital images of LCF-C, LDPF-4, and LDPF-6 at 254 nm light excitation; middle row: digital images of LCF-C, LDPF-4, and LDPF-6 at 365 nm light excitation; bottom row: digital images of LCF-C, LDPF-4, and LDPF-6 under visible light. | |
As mentioned above, all three light-conversion films could effectively absorb UV light. Considering the intrinsic properties of the light-conversion agents, the absorbed UV light has a high probability of being converted into red light. To study the light-conversion ability of the three light-conversion films, their fluorescence excitation spectra and fluorescence emission spectra were measured. As displayed in Fig. 2c, all three films displayed a similar broad excitation band in the range of 300–400 nm. The difference was that one more strong excitation band in the range of 250–300 nm was observed on the LDPF-4 and LDPF-6 films, resulting in a stronger shielding ability than LCF-C against the adverse effects of high-energy, low-band UV light on crops. Fig. 2d presents the emission spectra of the three light-conversion films, which displayed similar emission behaviors as the corresponding light-conversion agents. Consequently, the absolute fluorescence QYs of LCF-C, LDPF-4, and LDPF-6 were determined to be 2.71%, 1.42%, and 3.41%, respectively. Moreover, the LCF-C, LDPF-4, and LDPF-6 films were irradiated with 254 or 365 nm monochromatic UV light and compared with the visible-light one. As shown in Fig. 2e, under the irradiation of visible light, there was no obvious difference between these films. Once the films were irradiated with UV light, all the films emitted bright red light, indicating their good UV-to-red light-conversion efficiency. In comparison, the LDPF-6 film exhibited the deepest red color, followed by LDAP-4 and then LCF-C when irradiated with 254 nm UV light. Switching the UV light wavelength to 365 nm, the color depth decreased as follows: LCF-C > LDPF-6 > LDPF-4. These observed phenomena were consistent with their excitation spectra analysis.
Stability properties of the light-conversion agents in the light-conversion films
In the natural environment, doped light-conversion agents will migrate from the inner layer to the surface of the film gradually and will ultimately lose their light-conversion function. From the view of practical applications, the low migration rate of light-conversion agents in a film is highly desired. To investigate the stability of the light-conversion agents in film, the LCF-C, LDPF-4, and LDPF-6 films were soaked in polar ethanol (95%) for 12, 18, 24, 48, and 72 h, respectively, and the soaked solutions were measured by UV-vis spectrometry. As shown in Fig. 3a–c, the UV absorption intensities of the soaked solutions of the three films increased with time, which indicated that all the films exhibited a degree of light-conversion-agent migration in polar solution. To achieve a more direct comparison, the time-dependent UV-vis absorption intensities of the light-conversion-film-soaked solutions were plotted. As displayed in Fig. 3d, all three films showed a fast migration of the light-conversion agents in 24 h. After that, the absorption intensity of the soaked solutions increased a little with the prolonging of the time. In comparison, the LDPF-6 film with the longer alkyl-chain-linked light-conversion agents exhibited the lowest migration rate in ethanol solution over the whole test time, followed by LDPF-4 and then LCF-C. The smallest molecular weight and lack of hindered alkyl chain in the LCA-C molecule enabled LCA-C to migrate more easily in the PE film. These results demonstrate that using an alkyl chain to connect two luminescent centers is a promising strategy to increase the interaction between the light-conversion agent and the polymer backbone, resulting in high stability of the light-conversion agent in the film. LDPA-6 is a good light-conversion agent that is suitable for long-term use in light-conversion films and can effectively improve the service life of light-conversion films.
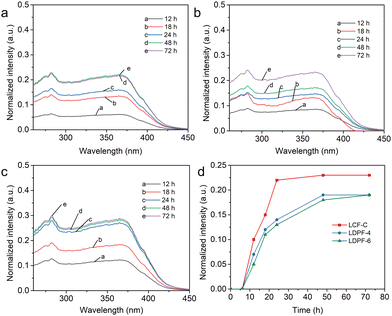 |
| Fig. 3 UV-Vis spectra of the light-conversion-agent film-soaked ethanol solution at different times: (a) LDPF-6, (b) LDPF-4, and (c) LCF-C, respectively. (d) Time-dependent UV absorption intensity of LCF-C-, LDPF-4-, and LDPF-6-soaked ethanol solution, respectively. | |
Mechanical properties of the light-conversion films
Predictably, the mechanical properties of the PE film will be affected after the introduction of a light-conversion agent. In view of this, the tensile strength and tear strength of the three light-conversion films were tested, and pure PE was taken as the reference sample, and the test results are listed in Table 1. As revealed, the introduction of a light-conversion agent brought about a distinct change in the mechanical properties of LCF-C, LDPF-4, and LDPF-6 when compared with pure PE. For instance, the longitudinal tensile strength and longitudinal elongation at break of LCF-C and LDPF-4 decreased to some extent, while their tear strength (both lateral and longitudinal) was observed to improve. In the case of LDPF-6, relative to pure PE, its mechanical properties in all aspects were enhanced, indicating the high compatibility of LDPA-6 in the matrix. The increase in the mechanical properties of LDPF-6 could be attributed to the long alky chain plasticization effect in LDPA-6. While the decrease in mechanical properties of LDPF-4 and LCF-F originated from the formation of defects in the film, which were caused by the aggregation of LDPA-4 and LCA-C, respectively. In comparison, the shorter distance between the two connected luminescent centers in LDPA-4 caused an easier aggregation. Hence, the decline in the mechanical properties of the LDPF-4 film was even more pronounced than that of LCF-C. These results indicate that a light-conversion agent with a special molecular structure in the film can not only convert UV to red light for crop growth but also act as a kind of plasticizer, and thereby, through a rational design, the mechanical properties of a film can be improved.
Table 1 Mechanical properties of the light-conversion films
Item |
LDPF-6 |
LDPF-4 |
LCF-C |
PE |
Standard |
Tensile strength (Mpa) |
Longitudinal |
28.36 |
20.03 |
22.66 |
24.63 |
≥16 |
Lateral |
26.64 |
21.47 |
21.21 |
21.21 |
Elongation at break (%) |
Longitudinal |
840.01 |
483.28 |
653.28 |
704.68 |
≥320 |
Lateral |
843.25 |
448.50 |
768.87 |
691.88 |
Tear strength (kN m−1) |
Longitudinal |
130.41 |
120.30 |
120.28 |
105.34 |
≥60 |
Lateral |
132.64 |
117.55 |
109.52 |
100.02 |
Thickness (mm) |
0.09 |
0.10 |
0.10 |
0.105 |
0.100 |
Biomass analysis of the light-conversion-film-treated rape samples
Typically, under a changing environment, plants can redistribute the biomass along the different parts of the plant to maintain their maximum growth rates.35 To study the effect of the light-conversion agents, the PE, LCF-C, LDPF-4, and LDPF-6 films were used to treat rape samples independently (The details are provided in the ESI†). The average biomass contents of the light-conversion-film-treated rapes are listed in Table 2. As revealed, the aboveground biomass contents of the LCF-C and LDPF-4 films-treated rape samples were 3.74 and 5.37 g, respectively, which were both less than that of the PE-treated rape samples of 5.543 g, which could be explained by the decreased visible-light transmittance of the LCF-C and LDPF-4 films in the range of 400–425 nm. In contrast, the aboveground biomass content of the LDPF-6 film-treated rape samples was measured to be 6.223 g, which was 1.12 times as much as that of the PE-treated ones. As for the belowground biomass, the PE, LCF-C, LDPF-4, and LDPF-6 films-treated rape samples achieved amounts of 0.131, 0.127, 0.152, and 0.176 g, respectively. Compared with the pure PE, both the LDPF-4 and LDPF-6 films-treated rape samples exhibited an increase in belowground biomass by 16.0% and 34.4%, respectively, whereas the LCF-C film still had less effect on the rape growth. Thus, a light-conversion film with a high UV-to-red light-conversion efficiency could play a critical role in increasing the biomass of the rape.
Table 2 Biomass amount of rape after treatment with the light-conversion films PE, LCF-C, LDPF-4, and LDPF-6, respectively
Films |
Aboveground biomass (g) |
Belowground biomass (g) |
PE |
5.543 |
0.131 |
LCF-C |
3.740 |
0.127 |
LDPF-4 |
5.370 |
0.152 |
LDPF-6 |
6.223 |
0.176 |
Yield analysis of the light-conversion-films-treated rape samples
Measurement of the rape samples yield is a direct way to verify the effect of the light-conversion film on rape growth. Therefore, we took photos of the rapes at the seedling stage (Fig. 4a–d) and mature stage (Fig. 4e–h). In the seedling stage, the LDPF-6 film had a distinct influence on the rape growth rate, whereby the treated rape had a high density of rape leaves, indicating the presence of the LDPF-6 film with a high red-light-emission efficiency accelerated the growth of the rape. While the other three film-treated rape samples showed a lower growth rate. In the mature stage, all four treated rape samples grew well, reflecting their good growing environment. To reveal the yield difference, the weight of the four matured rape samples was calculated.
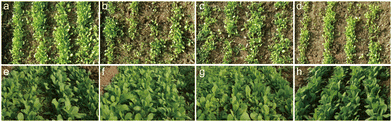 |
| Fig. 4 Digital photos of rapes in the early growth period by using the light-converting films: (a) LDPF-6, (b) LDPF-4, (c) LCF-C, and (d) PE, respectively. Digital photos of rapes in the ripening stage by using the light-converting films: (e) LDPF-6, (f) LDPF-4, (g) LCF-C, and (h) PE, respectively. | |
As shown in Fig. 5, the yield decreased as follows: LDPF-6 (2482.8 kg ha−1) > LCF-C (2281.9 kg ha−1) > LDPF-4 (2194.9 kg ha−1) > PE (2155.9 kg ha−1). Compared with PE, LCF-C, and LDPF-4, the yield of LDPF-6 was the highest, by 15.2%, 8.8%, and 13.1%, respectively. The highest rape yield achieved on the LDPF-6 could be attributed to the high light-conversion efficiency and high stability of the incorporated LDPA-6. For the LDPF-4 and PE film-treated samples, the rape yield was almost the same, and their small difference could be explained by the low UV to red-light conversion efficiency and large migration rate of LDPA-4. Based on the above discussions, the application of the newly prepared light-conversion film on rape could effectively improve its nutrition accumulation during the growth and development stages.
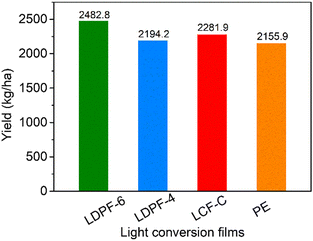 |
| Fig. 5 Yield of rapes after being treated with the light-conversion films: PE, LCF-C, LDPF-4, and LDPF-6, respectively. | |
Conclusions
In conclusion, two new light-conversion agents, LDPA-4 and LDPA-6, were successfully synthesized by linking two pyridine-2,6-dicarboxylic acid derivatives coordinated to Eu3+ luminescent centers with different lengths of the alkyl chain. The length of the alkyl chain had a big impact on their QY. As a result, LDPA-6 with an alkyl chain of six carbon atoms had the largest QY of 13.16% when compared with LDPA-4 (four carbon atoms) of 5.28% and the reference LCA-C of 10.77%. Moreover, the presence of a longer alkyl chain in LDPA-6 endowed it with the highest stability and compatibility in the PE film. By using LDPA-6-doped shed film, the growth rate and yield of rape were largely improved relative to the pure PE film. The treated rapes achieved the largest rape yield of 2482.8 kg ha−1, which was 1.15 times larger than that of pure film PE-treated ones. Characterizations and experimental plot results proved that the long-term stability and high intrinsic light-converting efficiency of LDPA-6 in the film ensured the rape could accept much more red light during the whole growth period. This work provides a new strategy to design high-performance light-converting films and demonstrate its successful practical applications.
Conflicts of interest
There are no conflicts to declare.
Acknowledgements
This work was supported by Science and Technology Project of Inner Mongolia Autonomous Region (2021GG0063), Central Public-interest Scientific Institution Basal Research Fund (No. BSRF202105), Key scientific and technological projects of Xinjiang production and Construction Corps (No. 2021AB007), and National Key Research and Development Program of China (No. 2021YFD1700700).
References
- D. Verdaguer, M. A. K. Jansen, L. Llorens, L. O. Morales and S. Neugart, UV-A radiation effects on higher plants: Exploring the known unknown, Plant Sci., 2017, 255, 72–81 CrossRef CAS PubMed.
- Y. Liu, Z. Gui and J. Liu, Research Progress of Light Wavelength Conversion Materials and Their Applications in Functional Agricultural Films, Polymers, 2022, 14, 851 CrossRef CAS PubMed.
- K.-W. Kim, G.-H. Kim, S.-H. Kwon, H.-I. Yoon, J.-E. Son and J.-H. Choi, Synthesis and photophysical properties of blue-emitting fluorescence dyes derived from naphthalimide derivatives containing a diacetylene linkage group, Dyes Pigm., 2018, 158, 353–361 CrossRef CAS.
- J. Zhang, X. Zhang, J. Zhang, W. Ma, X. Ji, S. Liao, Z. Qiu, W. Zhou, L. Yu and S. Lian, Near-UV-to-red light conversion through energy transfer in Ca2Sr(PO4)2:Ce3+,Mn2+ for plant growth, J. Mater. Chem. C, 2017, 5, 12069–12076 RSC.
- Y. Hasegawa, Y. Kitagawa and T. Nakanishi, Effective photosensitized, electrosensitized, and mechanosensitized luminescence of lanthanide complexes, NPG Asia Mater., 2018, 10, 52–70 CrossRef CAS.
- Y. Yu, Y. Wang, W. Liu, X. Jia, L. Ma, L. Ren, M. Xue and X. Liu, Exploration of highly efficient light conversion agents for agricultural film based on the bay-substituted perylene diimides derivatives, Dyes Pigm., 2018, 159, 483–490 CrossRef CAS.
- D.-H. Kim, Y. Chang, S. Park, M. G. Jeong, Y. Kwon, K. Zhou, J. Noh, Y.-K. Choi, T. M. Hong, Y.-T. Chang and S. H. Ryu, Blue-conversion of organic dyes produces artifacts in multicolor fluorescence imaging, Chem. Sci., 2021, 12, 8660–8667 RSC.
- W. Wu, Z. Zhang, R. Dong, G. Xie, J. Zhou, K. Wu, H. Zhang, Q. Cai and B. Lei, Characterization and properties of a Sr2Si5N8:Eu2 + -based light-conversion agricultural film, J. Rare Earths, 2020, 38, 539–545 CrossRef CAS.
- N. B. D. Lima, S. M. C. Gonçalves, S. A. Júnior and A. M. Simas, A Comprehensive Strategy to Boost the Quantum Yield of Luminescence of Europium Complexes, Sci. Rep., 2013, 3, 2395 CrossRef PubMed.
- Y. Hasegawa, Y. Wada and S. Yanagida, Strategies for the design of luminescent lanthanide(III) complexes and their photonic applications, J. Photochem. Photobiol., C, 2004, 5, 183–202 CrossRef CAS.
- G. F. de Sá, O. L. Malta, C. de Mello Donegá, A. M. Simas, R. L. Longo, P. A. Santa-Cruz and E. F. da Silva, Spectroscopic properties and design of highly luminescent lanthanide coordination complexes, Coord. Chem. Rev., 2000, 196, 165–195 CrossRef.
- V. Tsaryuk, V. Zolin, J. Legendziewicz, R. Szostak and J. Sokolnicki, Effect of ligand radicals on vibrational IR, Raman and vibronic spectra of europium β-diketonates, Spectrochim. Acta, Part A, 2005, 61, 185–191 CrossRef CAS PubMed.
- S. Biju, M. L. P. Reddy, A. H. Cowley and K. V. Vasudevan, Molecular Ladders of Lanthanide-3-phenyl-4-benzoyl-5-isoxazolonate and Bis(2-(diphenylphosphino)phenyl) Ether Oxide Complexes: The Role of the Ancillary Ligand in the Sensitization of Eu3+ and Tb3+ Luminescence, Cryst. Growth Des., 2009, 9, 3562–3569 CrossRef CAS.
- S. Zhao, L. Zhang, W. Li and L. Li, Preparation and Fluorescent Property of Eu(TTA)3Phen Incorporated in Polycarbonate Resin, Polym. J., 2006, 38, 523–526 CrossRef CAS.
- H. Xiao, M. Chen, C. Mei, H. Yin, X. Zhang and X. Cao, Eu(III), Tb(III) complexes with novel ligands containing pyridine-2,6-dicarboxylic acid unit: Synthesis, characterization, fluorescence properties and application in biological imaging, Spectrochim. Acta, Part A, 2011, 84, 238–242 CrossRef CAS PubMed.
- P. K. Shahi, A. K. Singh, S. K. Singh, S. B. Rai and B. Ullrich, Revelation of the Technological Versatility of the Eu(TTA)3Phen Complex by Demonstrating Energy Harvesting, Ultraviolet Light Detection, Temperature Sensing, and Laser Applications, ACS Appl. Mater. Interfaces, 2015, 7, 18231–18239 CrossRef CAS PubMed.
- G. Maggioni, A. Campagnaro, M. Tonezzer, S. Carturan and A. Quaranta, Deposition and Characterization of Luminescent Eu(tta)3phen-Doped Parylene-Based Thin-Film Materials, Chem. Phys. Chem., 2013, 14, 1853–1863 CrossRef CAS PubMed.
- C. Yang, Z.-F. Sun, L. Liu and L.-Q. Zhang, Preparation and luminescence performance of rare earth agriculture-used light transformation composites, J. Mater. Sci., 2008, 43, 1681–1687 CrossRef CAS.
- D. Wang, Y. Yu, X. Ai, H. Pan, H. Zhang and L. Dong, Polylactide/poly(butylene adipate-co-terephthalate)/rare earth complexes as biodegradable light conversion agricultural films, Polym. Adv. Technol., 2019, 30, 203–211 CrossRef CAS.
- Y. Yu, P. Xu, S. Jia, H. Pan, H. Zhang, D. Wang and L. Dong, Exploring polylactide/poly(butylene adipate-co-terephthalate)/rare earth complexes biodegradable light conversion agricultural films, Int. J. Biol. Macromol., 2019, 127, 210–221 CrossRef CAS PubMed.
- Y. Liu, J. Liu, Q. Liu, W. He and I. V. Kityk, Photophysical Spectral Features of fluorescent complexes on the basis of the novel ligand β-thujaplicin, J. Lumin., 2020, 218, 116852 CrossRef CAS.
- C. Tao, K. Du, Q. Yin, J. Zhu, H. Yan, F. Zhu and L. Zhang, Pyridine-2,6-dicarboxylic acid for the sensitization of europium(iii) luminescence with very long lifetimes, RSC Adv., 2015, 5, 58936–58942 RSC.
- A. Rodríguez-Rodríguez, D. Esteban-Gómez, R. Tripier, G. Tircsó, Z. Garda, I. Tóth, A. de Blas, T. Rodríguez-Blas and C. Platas-Iglesias, Lanthanide(III) Complexes with a Reinforced Cyclam Ligand Show Unprecedented Kinetic Inertness, J. Am. Chem. Soc., 2014, 136, 17954–17957 CrossRef PubMed.
- G. R. Choppin and Z. M. Wang, Correlation between Ligand Coordination Number and the Shift of the 7F0–5D0 Transition Frequency in Europium(III) Complexes, Inorg. Chem., 1997, 36, 249–252 CrossRef CAS.
- W. Li, Z. Yao, R. Yao and S. Liu, Polyethylene Grafted Polyether Pentaerythritol Mono-Maleate to Improve Wettability of Liquid on Polyethylene Films, Polym.-Plast. Technol. Eng., 2013, 52, 603–606 CrossRef CAS.
- Z. Lin, R. Kabe, N. Nishimura, K. Jinnai and C. Adachi, Organic Long-Persistent Luminescence from a Flexible and Transparent Doped Polymer, Adv. Mater., 2018, 30, 1803713 CrossRef PubMed.
- W. Lin, Y. Cui, J. Gao, J. Yu, T. Liang and G. Qian, Six-branched chromophores with isolation groups: synthesis and enhanced optical nonlinearity, J. Mater. Chem., 2012, 22, 9202–9208 RSC.
- H. Xu, F. Liu, S. Chen, W. Shi, Z. Xie, J. Wang, Z. Zhai, J. Liu, H. Li and J. Wang, Design and synthesis of novel H-Shaped chromophore for enhanced nonlinear optical properties, Dyes Pigm., 2019, 165, 144–150 CrossRef CAS.
- Z. a Li, P. Chen, Y. Xie, Z. Li and J. Qin, Ar–ArF Self-Assembly of Star-Shaped Second-Order Nonlinear Optical Chromophores Achieving Large Macroscopic Nonlinearities, Adv. Electron. Mater., 2017, 3, 1700138 CrossRef.
- B. Li, Z.-J. Ding, Z. Li and H. Li, Simultaneous enhancement of mechanical strength and luminescence performance in double-network supramolecular hydrogels, J. Mater. Chem. C, 2018, 6, 6869–6874 RSC.
- R. Boddula and S. Vaidyanathan, White light emissive bipolar ligand and their EuIII complex for white/red light emitting diodes, J. Photochem. Photobiol., A, 2017, 347, 26–40 CrossRef CAS.
- R. Boddula, J. Tagare, K. Singh and S. Vaidyanathan, White light-emissive europium complexes and their versatile applications, Mater. Chem. Front., 2021, 5, 3159–3175 RSC.
- T. Posati, F. Bellezza, A. Cipiciani, F. Costantino, M. Nocchetti, L. Tarpani and L. Latterini, Synthesis and Characterization of Luminescent Nanoclays, Cryst. Growth Des., 2010, 10, 2847–2850 CrossRef CAS.
- T. Zhu, P. Chen, H. Li, W. Sun, T. Gao and P. Yan, Structural effects on the photophysical properties of mono-β-diketonate and bis-β-diketonate EuIII complexes, Phys. Chem. Chem. Phys., 2015, 17, 16136–16144 RSC.
- S. Li, S. Dong, Y. Fu, B. Zhou, S. Liu, H. Shen, Y. Xu, X. Gao, J. Xiao, S. Wu and F. Li, Air or soil temperature matters the responses of alpine plants in biomass accumulation to climate warming, Sci. Total Environ., 2022, 844, 157141 CrossRef CAS PubMed.
Footnotes |
† Electronic supplementary information (ESI) available. See DOI: https://doi.org/10.1039/d2qm01035f |
‡ Maolin Zhang, Chipeng Zhao contributed equally to this work. |
|
This journal is © the Partner Organisations 2023 |
Click here to see how this site uses Cookies. View our privacy policy here.