DOI:
10.1039/D2QI02762C
(Research Article)
Inorg. Chem. Front., 2023,
10, 1818-1825
Electrochemical conversion of CO2 into HCOO− in a synergistic manner by a nanocomposite of Zn2SnO4/ZnO†
Received
30th December 2022
, Accepted 9th February 2023
First published on 15th February 2023
Abstract
Exploring environmentally friendly nanomaterials as alternatives to toxic metals for electrocatalytic CO2 reduction into formate is very important. Designing a nanocomposite catalyst with different phase components is an underlying strategy to achieve prominent catalysts for electrochemical CO2 reduction owing to the potential capacity to regulate electronic structure by interfacial interactions. Herein, we report a novel nanocomposite with components of cublic-like Zn2SnO4 and nanosheet-structured ZnO (Zn2SnO4/ZnO) as an efficient electrocatalyst for the CO2 reduction reaction (CO2RR). This strategy can reduce the use of toxic metals and the cost of the catalyst. The composite exhibited a maximum faradaic efficiency (FE) of 98% for the C1 product, and even approached 90% for formate production at −1.0 V versus the reversible hydrogen electrode, along with excellent durability. In constrast, the maximum FEHCOOH of a single ZnO component or Zn2SnO4 component were both <50% over the potential window. Coupling of X-ray photoelectron/adsorption spectroscopy and electrochemical measurements revealed interfacial charge transfer and oxygen vacancies to result in an optimized electronic structure of Zn2SnO4/ZnO. This was favorable for an HCOO* intermediate-involved reaction pathway, together with an enlarged electochemcial active area, which boosted the selectivity and activity of formate synergistically.
Introduction
The rapid rising of the carbon dioxide level in the Earth's atmosphere has caused serious climate change and subsequent chain reactions.1–5 Methods usually employed for CO2 conversion include thermal catalysis, photocatalysis, and electrocatalysis. Among these methods, electrocatalytic CO2 reduction to valuable fuels and chemicals powered by renewable energy resources can alleviate environmental issues effectively, but also realize the recycling of energy, thereby attracting great interest.6,7 The products of an electrochemical CO2 reduction reaction (CO2RR) are diverse: CO, HCOOH, CH4, C2H5OH, C2H4, and C2H6.8,9 Among them, HCOOH is considered to be a crucial raw material for basic organic chemicals that is commonly applied in food additives, printing, and dyeing.10 Formate is a potential choice for storing and delivering liquid hydrogen. Formate can release H2 if needed, and can be used as a chemical fuel for direct formate fuel cells. Besides, as a liquid product, fomate can be stored and transported readily, and saves the cost of liquefaction processes compared with gas products. Thus, formate presents the highest net value and is economically competitive if evaluated from a technoeconomic aspect.11 However, CO2 electroreduction to formate is hindered due to the high chemical inertness of CO2 (bond energy = 806 kJ mol−1), so a high overpotential is needed for activation.12 In addition, CO2RR involves competition from multiple reaction paths and is usually accompanied by other competitive side reactions, especially the hydrogen-evolution reaction (HER). Even though considerable effort has been devoted to promoting formate production, many problems are encountered, such as a low conversion rate and poor selectivity. Therefore, developing high-performance electrocatalysts to facilitate the CO2-to-formate conversion is highly desired, but challenging.
Some metals and their oxides electrodes, such as Pb,13 Bi,14 Sn,15 and In,16 possess relatively high selectivity towards CO2 reduction to formate. However, the expense of Pd, as well as the toxicity and environmental unfriendliness of In and Pd, makes them unsuitable for large-scale application. Hence, catalysts composed of elements characterized by high abundance and nontoxicity have garnered more attention. Recently, zinc-based catalysts have aroused wide interest due to the low cost, nontoxicity, and capacity for electrocatalytic CO2 reduction.17–19 For example, a ZnO sheet array supported on Zn foil showed a high faradaic efficiency (FE) of 85% for CO2RR to CO at −2.0 V (vs. Ag/AgCl).20 Moreover, Ce0.016Zn0.984O exhibited a maximum FECO of 88% at −1.0 V vs. the reversible hydrogen electrode (RHE) and excellent stability for continuous testing.21 Hence, Zn-based catalysts tend to electrocatalyze the reduction of CO2 to CO. Can the selectivity of ZnO be changed from CO to formate by dual engineering of electronic structure and morphology? In general, the precise manipulation of a component, morphology and size is an effective method to regulate the energy efficiency and selectivity for CO2RR by the “electronic effect” and “geometric effect”.22
Creation of heterostructures with different components affords a feasible approach for engineering the CO2RR performance by “tuning” the electronic structure.12,23 Due to a strong interaction at the interface, CuO/In2O3 composites enable remarkable electroreduction activity of CO2 to CO with a FECO of 93.0% at −0.7 vs. RHE.24 Guan and co-workers25 reported that SnO2/ZnO composites exhibited enhanced performance of CO2-to-HCOO− conversion with a maximum FEHCOO- of 76.70% at −1.16 V vs. RHE. Interfacial charge transport is enhanced in Zn2SnO4/SnO2 heterostructures, which greatly reduces the kinetic barriers of CO2RR and results in excellent selectivity for HCOOH.12 In addition, nanostructuring of composite catalysts would create preferential faceting, vacancy defects and more unsaturated coordination sites, thereby tuning their electronic structure as well as the binding energy of key intermediates.26
Herein, we developed an effective strategy for promoting CO2 electroreduction to formate by constructing a nanocomposite catalyst with Zn2SnO4 and ZnO phase components. With low Sn content of 6.65 wt%, the resultant Zn2SnO4/ZnO composite catalyst with oxygen vacancies exhibited greatly enhanced activity and selectivity for electrochemical CO2 reduction to formate, with a maximum FEHCOOH of 87% at −1.0 V vs. RHE and a larger partial current density of formate (46 mA cm−2), which was markedly superior to those of a single ZnO component or Zn2SnO4 component. Based on experimental analyses, the improved catalytic performance could be ascribed to three aspects. The first was a cubic-like structured Zn2SnO4 and nanosheet-structured ZnO that enabled intimate contact with each other, providing abundant ZnO-Zn2SnO4 heterostructure interfaces, promoting charge redistribution and altering the electronic structure. Second, the nanostructured hybrids were beneficial for mass transport and enlarged the contact between reaction intermediates and the Zn2SnO4/ZnO catalyst. Third, the optimized electronic properties were in favour of a HCOO* intermediate-involved reaction pathway (Fig. S1†), and thereby enhanced the selectivity of formate. This work highlights the design of composite electrocatalysts to render excellent catalytic performance by intimate interface interaction.
Results and discussion
A facile one-step hydrothermal method was applied to prepare the Zn2SnO4/ZnO composite catalyst. As displayed by the X-ray diffraction (XRD) pattern in Fig. 1a, all the peaks closely matched the main characteristic peaks of hexagonal-phase ZnO with a P63mc(186) space group (JCPDS number: 36-1451)27 and cubic-phase Zn2SnO4 (JCPDS number: 24-1470).28 Compared with pristine ZnO, the diffraction peaks of ZnO in Zn2SnO4/ZnO composite showed a slight shift towards a high diffraction angle (Fig. S2†), thereby demonstrating the strong interaction between ZnO and Zn2SnO4. This interaction may result in compressive strain in a composite catalyst and be favourable for reducing the energy barrier of HCOOH production, especially the potential-limiting step.29 The scanning electron microscope (SEM) image of the Zn2SnO4/ZnO catalyst is shown in Fig. 1b. It demonstrates the coexistence of a nanosheet and nano-cube in Zn2SnO4/ZnO, which was further confirmed by the transmission electron microscopy (TEM) image in Fig. 1c. As evidenced by a high-angle annular dark field (HAADF)-STEM image and corresponding elemental mappings in Fig. 1f–i, the component in Zn2SnO4/ZnO with nanosheet morphology was ZnO, whereas Zn2SnO4 was cubic. The thickness of the ZnO nanosheet was ∼20 nm, and resulted in more exposed active sites and increased the carrier density of the catalyst.30 Furthermore, the ZnO in Zn2SnO4/ZnO composite had a rough surface with many pore structures, which greatly improved the mass transfer rate of the catalyst (Fig. S3†). Fig. 1d shows the high-resolution TEM (HRTEM) image of the ZnO constituent. The clear lattice fringes with interlayer spacings of 0.16 nm and 0.26 nm were assigned to the (100) and (002) planes of ZnO, respectively. The inset of Fig. 1d shows the corresponding FFT image, which distinctly shows a single-crystal structure with excellent crystallinity. The HRTEM image and corresponding FFT pattern of the Zn2SnO4 constituent are displayed in Fig. 1e. The clear lattice spacing of 0.26 nm was assigned to the (311) planes of Zn2SnO4 with a Fd
m space group. Inductively coupled plasma-optical emission spectrometry (ICP-OES) revealed a relatively low Sn content of ∼6.65 wt% in the composite. Evidently, the ZnSnO4/ZnO composite had been synthesized successfully. To show the great advantage of forming a composite for electrochemical CO2 reduction, pristine ZnO and Zn2SnO4 were also prepared by a one-step hydrothermal method. The XRD patterns in Fig. S4 and 5† demonstrate the phases of the prepared reference materials to be hexagonal-phase ZnO and cubic-phase Zn2SnO4. Fig. S6† shows the SEM image of synthesized ZnO. Accordingly, the ZnO catalyst exhibited a nanosheet morphology with a similar thickness to that in the Zn2SnO4/ZnO composite, which increased the number of exposed active sites for CO2RR.
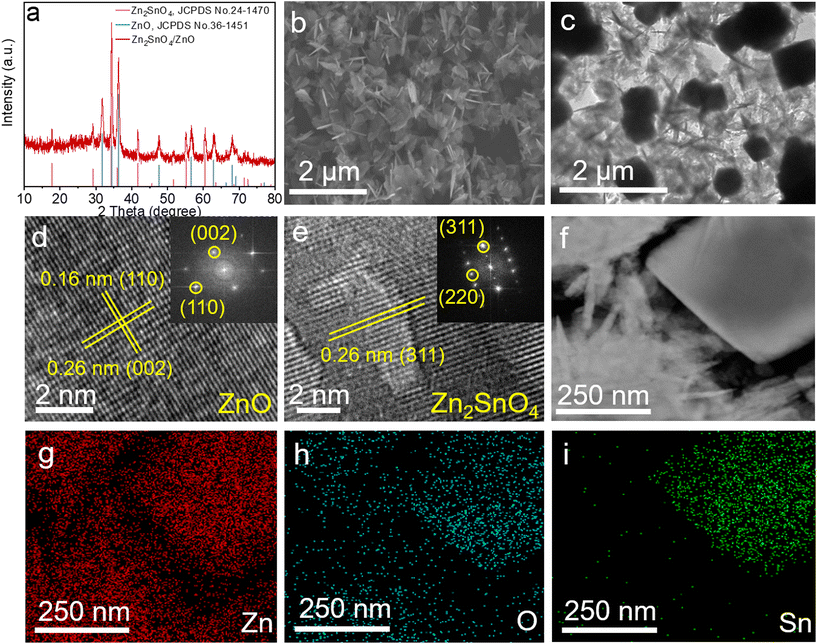 |
| Fig. 1 (a) XRD pattern of Zn2SnO4/ZnO. (b) SEM image of Zn2SnO4/ZnO. (c) TEM image of Zn2SnO4/ZnO. (d) HRTEM image and corresponding FFT pattern of the ZnO constituent. (e) HRTEM image and corresponding FFT pattern of the Zn2SnO4 constituent. (f) STEM of Zn2SnO4/ZnO. (g–i) EDS mapping of Zn, O and Sn. | |
To gain deep insight into the coordination environment and electronic state of the Zn2SnO4/ZnO catalyst, X-ray photoelectron spectroscopy (XPS), X-ray absorption near-edge structure spectroscopy (XANES), and extended X-ray absorption fine structure (EXAFS) spectroscopy were carried out. The XPS survey spectrum in Fig. 2a shows the constituent elements of Zn, Sn, and O in Zn2SnO4/ZnO, which was in agreement with the elemental mapping results stated above. The Zn 2p spectrum of ZnO exhibited two symmetrical peaks of Zn 2p3/2 and Zn 2p1/2 at 1020.6 and 1043.8 eV, respectively (Fig. 2b).31 The two peaks corresponding to Zn 2p3/2 and 2p1/2 of Zn2SnO4 were located at 1022.8 eV and 1045.9 eV. The two relative peaks in Zn2SnO4/ZnO were positioned at 1021.19 and 1044.28 eV, respectively. These results demonstrated that the valence of Zn in the Zn2SnO4/ZnO composite had been optimized, and lay between that of pristine Zn2SnO4 and ZnO, and perhaps contributed to the enhanced activity and selectivity towards CO2 electroreduction to formate. Fig. 2c shows the Sn 3d XPS spectra of Zn2SnO4/ZnO and Zn2SnO4. In the case of Zn2SnO4, the two main peaks with binding energies of 494.2 eV and 485.6 eV belonged to the Sn 3d3/2 and Sn 3d5/2 of Sn4+.32 Two smaller peaks at 492.5 eV and 484.0 eV were ascribed to the small amount of metallic Sn.33 When forming a composite with ZnO, both of these two peaks of Sn 3d3/2 and Sn 3d5/2 of Sn4+ showed a slight shift towards high binding energies and were located at 494.7 and 486.1 eV, respectively, thereby indicating electron depletion around Sn atoms.32 In the high-resolution O 1s spectrum of Zn2SnO4, two peaks centered at 529.3 eV and 530.5 eV were attributed to the coordination of O in Sn–O–Sn and Sn–O–Zn, respectively (Fig. 2d).12 For ZnO, two peaks with binding energies of 529.9 and 531.3 eV could be observed in the O 1s spectrum, and were attributed to lattice O and oxygen vacancies (Ov).34 When forming Zn2SnO4/ZnO composite materials, the peaks related to Ov and lattice O shifted to high binding energies, which demonstrated the charge redistribution in a composite system. These Ov defects play an important part in increasing the selectivity of formate and the active sites of CO2RR.35,36 Furthermore, the H* adsorption free energy could be weakened by Ov and, thus, inhibit the HER side reaction.37–39
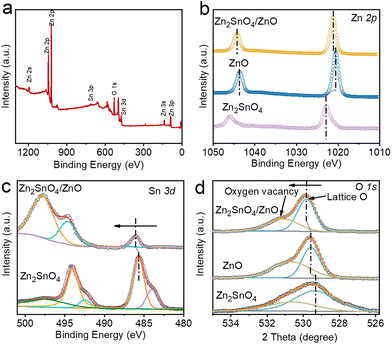 |
| Fig. 2 Characterizations of electronic structure. (a) XPS survey spectrum of Zn2SnO4/ZnO. (b) High-resolution Zn 2p XPS spectra of Zn2SnO4/ZnO, ZnO and Zn2SnO4. (c) High-resolution Sn 3d XPS spectra of Zn2SnO4/ZnO and Zn2SnO4. (d) High-resolution O 1s XPS spectra of Zn2SnO4/ZnO, ZnO and Zn2SnO4. | |
Fig. 3a displays the Zn K-edge XANES spectrum of Zn2SnO4/ZnO compared with prepared ZnO, commercial ZnO (Comm. ZnO), and commercial Zn (Comm. Zn). In the case of prepared ZnO, the main absorption peak at 9668 eV and anterior resonance peak of 9683 eV are typical features of hexagonal-phase ZnO.35 However, its pre-edge adsorption exhibited an obvious negative shift in comparison with that of Comm. ZnO, thereby demonstrating the lower oxidation state of Zn in prepared ZnO.36 Meanwhile, the pre-edge adsorption of Zn2SnO4/ZnO had a slightly positive shift compared with that of prepared ZnO, but lower than that of Comm. ZnO, implying that the valence state of Zn in formed Zn2SnO4/ZnO was lower than +2. The Fourier transform (FT) spectra of Zn2SnO4/ZnO and reference samples are shown in Fig. 3b. The first coordination shell in prepared ZnO had Zn–O coordination with a scattering peak at 1.58 Å, which was right-shifted by 0.02 Å compared with that in Comm. ZnO, and demonstrated an elongated Zn–O bond in prepared ZnO compared with that in Comm. ZnO. However, the peak associated with Zn–O coordination was located at 1.57 Å for Zn2SnO4/ZnO. This value was larger than that of Comm. ZnO but smaller than that of prepared ZnO, which indicated the charge redistribution of the Zn2SnO4/ZnO composite. To further study the changes in valence state and electronic structure, R and k space-involved wavelet transform (WT) analyses were undertaken. The WT EXAFS contour plots of Comm. ZnO, Comm. Zn, prepared ZnO and Zn2SnO4/ZnO are shown in Fig. 3c–f. The WT plots of Zn2SnO4/ZnO showed two maxima at 6.27 Å−1 and 7.38 Å−1, which belonged to Zn–O and Zn–Zn co-ordinations, respectively. The intensity and k-value of the two backscattering paths were similar to those of Comm. ZnO and prepared ZnO, implying the same structural order.
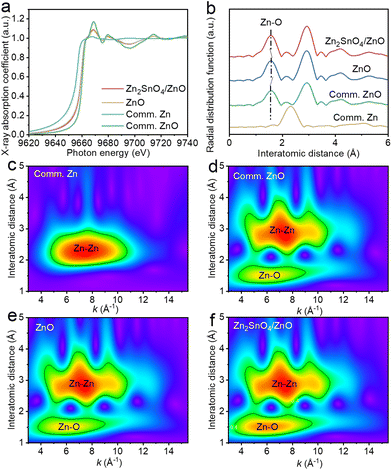 |
| Fig. 3 (a) Zn K-edge XANES and (b) k3-weighted FT-EXAFS spectra of ZnO, Zn2SnO4/ZnO and reference materials. WT analysis of (c) Comm. Zn, (d) Comm. ZnO, (e) ZnO and (f) Zn2SnO4/ZnO. | |
The Sn K-edge XANES spectra of Zn2SnO4/ZnO and commercial references are displayed in Fig. 4a. The higher maximum adsorption of Zn2SnO4/ZnO than that of commercial SnO2 (Comm. SnO2) demonstrated the higher degree of structural distortion.40 Moreover, the slightly right-shifted white-line peak of Zn2SnO4/ZnO compared with Comm. SnO2 demonstrated the lower electron density of Sn in the composite. Fig. 4b illustrates the FT-EXAFS spectra of Zn2SnO4/ZnO, Comm. SnO2, Sn and SnO. For Comm. SnO2, two scattering peaks related to Sn–O and Sn–Sn were detected. The spectrum of Zn2SnO4/ZnO displayed a dominant peak at 1.59 Å (attributed to a Sn–O interaction) and a lower-intensity peak at 3.43 Å (corresponding to a Sn–Sn interaction). Both peaks were negative-shifted relative to those of Comm. SnO2. Besides, a new peak appeared at 2.69 Å for Zn2SnO4/ZnO, which could be assigned to a Sn–Zn interaction.41 A maximum at 6.6 Å−1 was observed in the WT plots of Zn2SnO4/ZnO, which corresponded to the Sn–O interaction compared with the references Comm. SnO2, SnO and Sn foil (Fig. 4c–f). The k-value of the Sn–O path in Zn2SnO4/ZnO was similar to that of Comm. SnO2, but the intensity was greater.
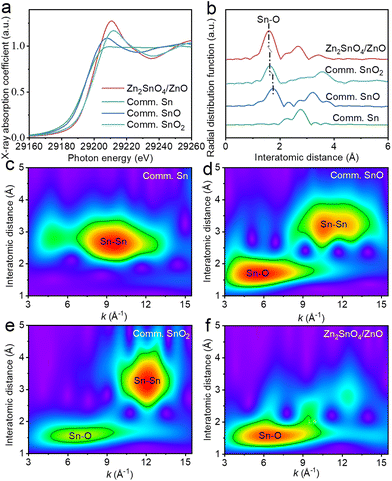 |
| Fig. 4 (a) Sn K-edge XANES and (b) k3-weighted FT-EXAFS spectra of Zn2SnO4/ZnO and reference materials. WT analysis of (c) Comm. Sn, (d) Comm. SnO, (e) Comm. SnO2 and (f) Zn2SnO4/ZnO. | |
The CO2RR performance of Zn2SnO4/ZnO composite was investigated in a flow cell using a three electrode system (Fig. S7†). The performance of pristine Zn2SnO4 and ZnO was also evaluated as references. The linear sweep voltammetry (LSV) curves of Zn2SnO4/ZnO, ZnO and Zn2SnO4 in CO2- saturated and Ar-saturated 0.5 M KHCO3 electrolyte are displayed in Fig. S8.† All three catalysts exhibited enhanced current density in the CO2-saturated electrolyte than in the Ar-saturated electrolyte. More importantly, the current density of Zn2SnO4/ZnO in CO2-saturated solution was greater than that of pristine ZnO and Zn2SnO4 (Fig. 5a), which demonstrated the enhanced activity of a composite catalyst towards CO2 reduction. The products of CO2RR on Zn2SnO4/ZnO and reference catalysts were evaluated after continuous electrochemical measurement for 1 h under different potentials (Fig. 5b). Gas products were quantified via on-line gas chromatography (Fig. S9†). Liquid products were measured by 1H nuclear magnetic resonance (NMR) spectroscopy (Fig. S10†). Fig. 5c shows that synthetic ZnO exhibited selectivity for formate production with a maximum faradaic efficiency (FE) of 38% at an applied potential of −0.9 V vs. RHE, which is high selectivity for a ZnO-based catalyst for HCOOH production compared with that reported previously. This result could be attributed to oxygen vacancies enhancing CO2 adsorption and two-dimensional nanosheet morphology, which leads to special crystal orientation. Even so, CO was the main product of CO2RR on a ZnO catalyst with FE >60% at all applied potentials. In the case of Zn2SnO4, the main product of CO2RR was HCOOH, but FEHCOOH was <50% over all applied potentials (Fig. 5d). The FECO and FEH2 for Zn2SnO4 are displayed in Fig. S11.† Upon formation of a composite, Zn2SnO4/ZnO exhibited greatly enhanced selectivity for formate with a maximum FEHCOOH of 87% at −1.0 V vs. RHE. Moreover, the FEHCOOH of Zn2SnO4/ZnO was maintained at >80% at a wide potential window (−0.8 V to −1.1 V vs. RHE) (Fig. 5e and f). The total C1 products, including CO and formate, was >97% for Zn2SnO4/ZnO over a potential window of −0.8 V to −1.1 V vs. RHE (Fig. S11†), thereby demonstrating a strong capacity for suppressing the HER.
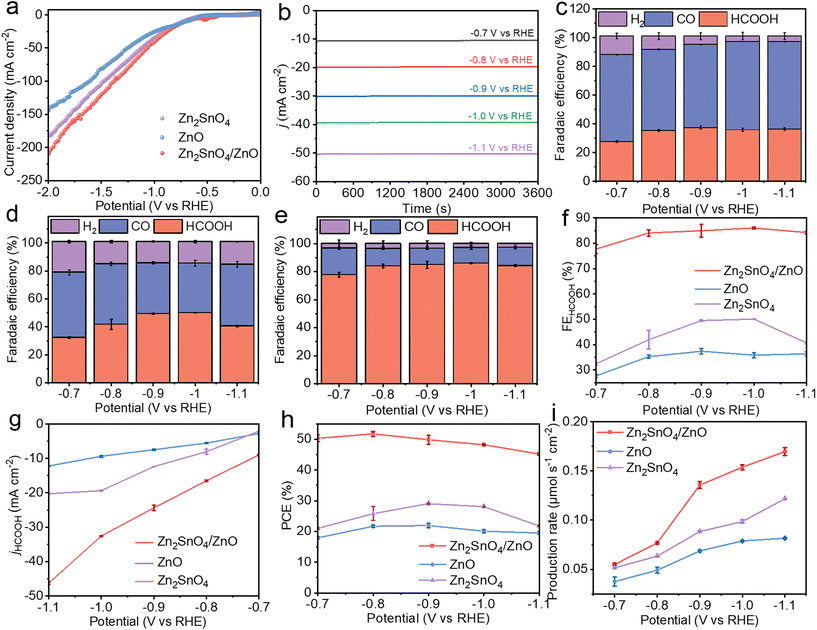 |
| Fig. 5 (a) LSV curves of Zn2SnO4/ZnO, Zn2SnO4 and ZnO in 0.5 M KHCO3 saturated with CO2. (b) I–t curves of Zn2SnO4/ZnO at various potentials. FE towards H2, CO and HCOOH of (c) prepared ZnO, (d) Zn2SnO4 and (e) Zn2SnO4/ZnO. (f) FEHCOOH of prepared ZnO, Zn2SnO4 and Zn2SnO4/ZnO. (g) HCOOH partial current density of prepared ZnO, Zn2SnO4 and Zn2SnO4/ZnO. (h) Half-cell PCE for CO2-to-formate conversion for Zn2SnO4/ZnO, prepared ZnO and Zn2SnO4, respectively. (i) Formate production rate at different voltages for Zn2SnO4/ZnO, prepared ZnO and Zn2SnO4. | |
Zn2SnO4/ZnO exhibited excellent performance towards HCOOH production in comparison with single ZnO and Zn2SnO4, which corroborated the advantages of a synergistic effect in Zn2SnO4/ZnO. The partial current density of HCOOH was 46 mA cm−2 at −1.1 V vs. RHE, which was 2.3-fold and 3.8-fold larger than that of Zn2SnO4 and ZnO, respectively (Fig. 5g). The partial current density of H2 and CO on three typical electrocatalysts is shown in Fig. S12.† Accordingly, the half-cell PCE37 of CO2-to-formate on the Zn2SnO4/ZnO composite was ∼45% over the potential window, which was much higher than that of Zn2SnO4 and ZnO (Fig. 5h). As illustrated by Fig. 5i, a high formate production rate36 of 0.17 μmol s−1 cm−1 was delivered by Zn2SnO4/ZnO at −1.1 V vs. RHE, which was much higher than that of ZnO (0.08 μmol s−1 cm−1) and Zn2SnO4 (0.12 μmol s−1 cm−1). These data demonstrated the enhanced activity and selectivity of the Zn2SnO4/ZnO composite towards CO2-to-formate conversion.
Inspired by the superior catalytic performance of the composite electrode, the electrochemical active surface area (ECSA) was measured by cyclic voltammetry under various potentials to assess the number of active sites (Fig. S13†). As reported,40,42,43 ECSA is directly proportional to double-layer capacitance (Cdl). As confirmed by Fig. 6a, Zn2SnO4/ZnO exhibited a higher Cdl (0.246 mF cm−2) than that of Zn2SnO4 (0.162 mF cm−2) or ZnO (0.196 mF cm−2), which indicated more exposed active sites of the composite catalyst. The ECSA of Zn2SnO4/ZnO, ZnO and Zn2SnO4 was calculated to be 0.6 cm2, 0.47 cm2, and 0.39 cm2, respectively. However, the ESCA-normalized partial current density of formate for Zn2SnO4/ZnO was 77 mA cm−2 at −1.1 V vs. RHE, which was 1.5-times and 1.8-times higher than that of Zn2SnO4 and ZnO (Fig. 6b), which demonstrated the enhanced intrinsic catalytic activity of active sites in the Zn2SnO4/ZnO composite. The enhanced ECSA-normalized total current density also confirmed the improvement of catalytic activity of Zn2SnO4/ZnO (Fig. S14†). Electrochemical impedance spectroscopy of three typical catalysts were also measured for the study of charge-transfer kinetics (Fig. 6c). Zn2SnO4/ZnO possessed the fastest electrode kinetics, as evidenced by the smallest charge transfer resistance (Rct) derived from Nyquist plots.44–46 A chronoamperometric (i–t) test at −1.0 V (vs. RHE) was conducted to investigate the electrochemical durability of Zn2SnO4/ZnO. As evidenced by Fig. 6d, Zn2SnO4/ZnO could exhibit an increase in total current density during the first hour of testing, which was attributed to activation of the catalyst. Afterwards, a stable total current density of −125 mA cm−2 was maintained over 11 h. The FEHCOOH of Zn2SnO4/ZnO was >80% over the whole period of stability testing, thereby indicating the excellent electrochemical stability of Zn2SnO4/ZnO for CO2-to-formate conversion. Accordingly, the greater number of exposed active sites, excellent ability for electron transfer and optimized surface electronic structure contributed synergistically to the enhanced activity and selectivity of the Zn2SnO4/ZnO composite for electroreduction of CO2 to HCOO−.
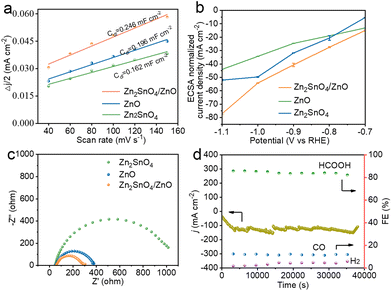 |
| Fig. 6 (a) Cdl of Zn2SnO4/ZnO, Zn2SnO4 and ZnO. (b) ECSA-normalized formate partial current density of Zn2SnO4/ZnO, Zn2SnO4 and ZnO. (c) Nyquist plots of Zn2SnO4/ZnO, Zn2SnO4 and ZnO. (d) Stability test on the Zn2SnO4/ZnO electrode at −1.0 V (vs. RHE). | |
Conclusions
A novel Zn2SnO4/ZnO heterostructure nanocomposite catalyst was synthesized via a simple one-step hydrothermal method and applied for CO2 electroreduction in 0.5 M KHCO3 solution. We report, for the first time, that this Zn2SnO4/ZnO hybrid material was employed for electrochemical CO2-to-formate conversion. Advanced characterization involving XPS and X-ray adsorption spectroscopy demonstrated the charge redistribution of Zn2SnO4/ZnO driven by intimate interfacial interaction. The resultant Zn2SnO4/ZnO composite catalyst exhibited significantly enhanced selectivity and activity for CO2RR to formate compared with that of constituent ZnO and Zn2SnO4. A remarkable FEHCOOH of up to 87% at −1.0 V vs. RHE was achieved, surpassing the maximum FEHCOOH of ZnO (38%) and Zn2SnO4 (50%) significantly. The greatly enhanced performance for electrochemical CO2 reduction to formate was due to the optimized electronic structure, higher electrochemically active surface area and the presence of oxygen vacancies on the surface. Our study may provide new insights for the design of electrocatalysts with high selectivity and activity for CO2RR by a synergistic effect of the constructed nanocomposite.
Conflicts of interest
There are no conflicts to declare.
Acknowledgements
This work was supported by the Taishan Scholar Program of Shandong Province, China (ts201712045), National Natural Science Foundation of China (22102079) and Natural Science Foundation of Shandong Province of China (ZR2021YQ10).
References
- M. Yamamoto-Kawai, F. A. McLaughlin, E. C. Carmack, S. Nishino and K. J. S. Shimada, Aragonite Undersaturation in the Arctic Ocean: Effects of Ocean Acidification and Sea Ice Melt, Science, 2009, 326, 1098–1100 CrossRef CAS PubMed
.
- X. Duan, J. Xu, Z. Wei, J. Ma, S. Guo, S. Wang, H. Liu and S. Dou, Metal-Free Carbon Materials for CO2 Electrochemical Reduction, Adv. Mater., 2017, 29, 1701784 CrossRef PubMed
.
- M. Zhong, K. Tran, Y. Min, C. Wang, Z. Wang, C.-T. Dinh, P. De Luna, Z. Yu, A. S. Rasouli and P. J. N. Brodersen, Accelerated discovery of CO2 electrocatalysts using active machine learning, Nature, 2020, 581, 178–183 CrossRef CAS PubMed
.
- S. C. Peter, Reduction of CO2 to Chemicals and Fuels: A Solution to Global Warming and Energy Crisis, ACS Energy Lett., 2018, 3, 1557–1561 CrossRef CAS
.
-
C.-T. A. Lee, H. Jiang, R. Dasgupta and M. Torres, A Framework for Understanding Whole-Earth Carbon Cycling, Deep Carbon: Past to Present, Cambridge University Press, 2019, 313–357 Search PubMed
.
- S. Zhu, E. P. Delmo, T. Li, X. Qin, J. Tian, L. Zhang and M. Shao, Recent Advances in Catalyst Structure and Composition Engineering Strategies for Regulating CO2 Electrochemical Reduction, Adv. Mater., 2021, 33, 2005484 CrossRef CAS PubMed
.
- J. Feng, S. Zeng, J. Feng, H. Dong and X. J. Zhang, CO2 Electroreduction in Ionic Liquids: A Review, Chin. J. Chem., 2018, 36, 961–970 CrossRef CAS
.
- Y. Wang, Z. Chen, P. Han, Y. Du, Z. Gu, X. Xu and G. Zheng, Single-Atomic Cu with Multiple Oxygen Vacancies on Ceria for Electrocatalytic CO2 Reduction to CH4, ACS Catal., 2018, 8, 7113–7119 CrossRef CAS
.
- Y. Guo, X. He, Y. Su, Y. Dai, M. Xie, S. Yang, J. Chen, K. Wang, D. Zhou and C. Wang, Machine-Learning-Guided Discovery and Optimization of Additives in Preparing Cu Catalysts for CO2 Reduction, J. Am. Chem. Soc., 2021, 143, 5755–5762 CrossRef CAS PubMed
.
- H. Zeidan and M. E. Marti, Separation of Formic Acid from Aqueous Solutions onto Anion Exchange Resins: Equilibrium, Kinetic, and Thermodynamic Data, J. Chem. Eng., 2019, 64, 2718–2727 CAS
.
- I. Dutta, S. Chatterjee, H. Cheng, R. K. Parsapur, Z. Liu, Z. Li, E. Ye, H. Kawanami, J. S. C. Low, Z. Lai, X. J. Loh and K. W. Huang, Formic Acid to Power towards Low-Carbon Economy, Adv. Energy Mater., 2022, 12, 2103799 CrossRef CAS
.
- K. Wang, D. Liu, P. Deng, L. Liu, S. Lu, Z. Sun, Y. Ma, Y. Wang, M. Li, B. Y. Xia, C. Xiao and S. Ding, Band alignment in Zn2SnO4/SnO2 heterostructure enabling efficient CO2 electrochemical reduction, Nano Energy, 2019, 64, 103954 CrossRef CAS
.
- W. Zhang, Y. Hu, L. Ma, G. Zhu, Y. Wang, X. Xue, R. Chen, S. Yang and Z. Jin, Progress and Perspective of Electrocatalytic CO2 Reduction for Renewable Carbonaceous Fuels and Chemicals, Adv. Sci., 2018, 5, 1700275 CrossRef PubMed
.
- X. Feng, H. Zou, R. Zheng, W. Wei, R. Wang, W. Zou, G. Lim, J. Hong, L. Duan and H. Chen, Bi2O3/BiO2 Nanoheterojunction for Highly Efficient Electrocatalytic
CO2 Reduction to Formate, Nano Lett., 2022, 22, 1656–1664 CrossRef CAS PubMed
.
- F. Cheng, X. Zhang, K. Mu, X. Ma, M. Jiao, Z. Wang, P. Limpachanangkul, B. Chalermsinnsuwan, Y. Gao, Y. Li, Z. Chen and L. Liu, Recent Progress of Sn-Based Derivative Catalysts for Electrochemical Reduction of CO2, Energy Technol., 2020, 9, 2000799 CrossRef
.
- Y. Huang, X. Mao, G. Yuan, D. Zhang, B. Pan, J. Deng, Y. Shi, N. Han, C. Li, L. Zhang, L. Wang, L. He, Y. Li and Y. Li, Size-Dependent Selectivity of Electrochemical CO2 Reduction on Converted In2O3 Nanocrystals, Angew. Chem., Int. Ed., 2021, 60, 15844–15848 CrossRef CAS PubMed
.
- B. Ren, Z. Zhang, G. Wen, X. Zhang, M. Xu, Y. Weng, Y. Nie, H. Dou, Y. Jiang, Y. P. Deng, G. Sun, D. Luo, L. Shui, X. Wang, M. Feng, A. Yu and Z. Chen, Dual-Scale Integration Design of Sn–ZnO Catalyst toward Efficient and Stable CO2 Electroreduction, Adv. Mater., 2022, 34, 2204637 CrossRef CAS PubMed
.
- Z. Zhang, G. Wen, D. Luo, B. Ren, Y. Zhu, R. Gao, H. Dou, G. Sun, M. Feng, Z. Bai, A. Yu and Z. Chen, “Two Ships in a Bottle” Design for Zn–Ag–O Catalyst Enabling Selective and Long-Lasting CO2 Electroreduction, J. Am. Chem. Soc., 2021, 143, 6855–6864 CrossRef CAS PubMed
.
- X. Jiang, F. Cai, D. Gao, J. Dong, S. Miao, G. Wang and X. Bao, Electrocatalytic reduction of carbon dioxide over reduced nanoporous zinc oxide, Electrochem. Commun., 2016, 68, 67–70 CrossRef CAS
.
- H.-i. Nam, K. R. Park, Y.-W. Choi, H.-j. Sim, K. Y. Sohn and D.-H. Lim, Electrocatalytic CO2 reduction using self-supported zinc sulfide arrays for selective CO production, Appl. Surf. Sci., 2023, 612, 155646 CrossRef CAS
.
- X. Ren, Y. Gao, L. Zheng, Z. Wang, P. Wang, Z. Zheng, Y. Liu, H. Cheng, Y. Dai and B. Huang, Oxygen vacancy enhancing CO2 electrochemical reduction to CO on Ce-doped ZnO catalysts, Surf. Interfaces, 2021, 23, 100923 CrossRef CAS
.
- D. Yang, B. Ni and X. Wang, Heterogeneous Catalysts with Well-Defined Active Metal Sites toward CO2 Electrocatalytic Reduction, Adv. Energy Mater., 2022, 2001142 Search PubMed
.
- C. Lv, C. Yan, G. Chen, Y. Ding, J. Sun, Y. Zhou and G. Yu, An Amorphous Noble-Metal-Free Electrocatalyst that Enables Nitrogen Fixation under Ambient Conditions, Angew. Chem., 2018, 130, 6181–6184 CrossRef
.
- S. Chu, S. Hong, J. Masa, X. Li and Z. Sun, Synergistic catalysis of CuO/In2O3 composites for highly selective electrochemical CO2 reduction to CO, Chem. Commun., 2019, 55, 12380–12383 RSC
.
- Y. Guan, Y. Liu, J. Yi and J. Zhang, Zeolitic imidazolate framework-derived composites with SnO2 and ZnO phase components for electrocatalytic carbon dioxide reduction, Dalton Trans., 2022, 51, 7274–7283 RSC
.
- L. Wang, W. Chen, D. Zhang, Y. Du, R. Amal, S. Qiao, J. Wu and Z. Yin, Surface strategies for catalytic CO2 reduction: from two-dimensional materials to nanoclusters to single atoms, Chem. Soc. Rev., 2019, 48, 5310–5349 RSC
.
- Z. Zheng, W. Liang, R. Lin, Z. Hu, Y. Wang, H. Lu, W. Zhong, S. Shen and Y. Pan, Facile Synthesis of Zinc Indium Oxide Nanofibers Distributed with Low Content of Silver for Superior Antibacterial Activity, Small Struct., 2023, 2200291 CrossRef
.
- Q. Lu, Z. Wei, C. Li, J. Ma and L. Li, Photocatalytic degradation of methyl orange by noble metal Ag modified semiconductor Zn2SnO4, Mater. Sci. Semicond. Process., 2022, 138, 106290 CrossRef CAS
.
- Y. Xing, X. Kong, X. Guo, Y. Liu, Q. Li, Y. Zhang, Y. Sheng, X. Yang, Z. Geng and J. Zeng, Bi@Sn Core–Shell Structure with Compressive Strain Boosts the Electroreduction of CO2 into Formic Acid, Adv. Sci., 2020, 7, 1902989 CrossRef CAS PubMed
.
- X. Wan, X. Liu, Y. Li, R. Yu, L. Zheng, W. Yan, H. Wang, M. Xu and J. Shui, Fe–N–C electrocatalyst with dense active sites and efficient mass transport for high-performance proton exchange membrane fuel cells, Nat. Catal., 2019, 2, 259–268 CrossRef CAS
.
- J. Zhang, S. Shao, D. Zhou, Q. Xu and T. Wang, ZnO nanowire arrays decorated 3D N-doped reduced graphene oxide nanotube framework for enhanced photocatalytic CO2 reduction performance, J. CO2 Util., 2021, 50, 101584 CrossRef CAS
.
- S. Jain, S. Verma, S. P. Singh and S. N. Sharma, An electrochemical biosensor based on novel butylamine capped CZTS nanoparticles immobilized by uricase for uric acid detection, Biosens. Bioelectron., 2019, 1279, 135–141 CrossRef PubMed
.
- Y. Zhang, H. Jang, X. Ge, W. Zhang, Z. Li, L. Hou, L. Zhai, X. Wei, Z. Wang, M. G. Kim, S. Liu, Q. Qin and X. Liu, Single-Atom Sn on Tensile-Strained ZnO Nanosheets for Highly Efficient Conversion of CO2 into Formate, Adv. Energy Mater., 2022, 12, 2202695 CrossRef CAS
.
- Z. Chen, T. Fan, Y.-Q. Zhang, J. Xiao, M. Gao, N. Duan, J. Zhang, J. Li, Q. Liu, X. Yi and J.-L. Luo, Wavy SnO2 catalyzed simultaneous reinforcement of carbon dioxide adsorption and activation towards electrochemical conversion of CO2 to HCOOH, Appl. Catal., B, 2020, 261, 118243 CrossRef CAS
.
- R. Daiyan, E. C. Lovell, B. Huang, M. Zubair, J. Leverett, Q. Zhang, S. Lim, J. Horlyck, J. Tang and X. Lu, Uncovering Atomic-Scale Stability and Reactivity in Engineered Zinc Oxide Electrocatalysts for Controllable Syngas Production, Adv. Energy Mater., 2020, 10, 2001381 CrossRef CAS
.
- Z. Geng, X. Kong, W. Chen, H. Su, Y. Liu, F. Cai, G. Wang and J. Zeng, Oxygen Vacancies in ZnO Nanosheets Enhance CO2 Electrochemical Reduction to CO, Angew. Chem., Int. Ed., 2018, 57, 6054–6059 CrossRef CAS PubMed
.
- B. Ning, M. Liu, Y. Hu, H. Jiang and C. Li, Defect engineered SnO2 nanoparticles enable strong CO2 chemisorption toward efficient electroconversion to formate, Dalton Trans., 2022, 51, 3512–3519 RSC
.
- Y. Ding, L. Zhao, Y. Tong, W. Kong, B. Li, J. Wang, X. Han, W. Xing and J. Xu, Designing High-Performance Se-Decorated Edges of MoSe2 Nanostripes for the Hydrogen Oxidation Reaction: Effect of Transition Metal Doping, J. Phys. Chem. C, 2022, 126, 13617–13628 CrossRef CAS
.
- Z. Li, A. Cao, Q. Zheng, Y. Fu, T. Wang, K. T. Arul, J. L. Chen, B. Yang, N. M. Adli, L. Lei, C. L. Dong, J. Xiao, G. Wu and Y. Hou, Elucidation of the Synergistic Effect of Dopants and Vacancies on Promoted Selectivity for CO2 Electroreduction to Formate, Adv. Mater., 2021, 33, 2005113 CrossRef CAS PubMed
.
- G. Li, H. Jang, S. Liu, Z. Li, M. G. Kim, Q. Qin, X. Liu and J. Cho, The synergistic effect of Hf-O-Ru bonds and oxygen vacancies in Ru/HfO2 for enhanced hydrogen evolution, Nat. Commun., 2022, 13, 1270 CrossRef CAS PubMed
.
- N.-E. Sung, K. H. Chae and I.-J. Lee, Structural Characterization of Zinc-Tin-Oxide Films Deposited on Quartz Substrates by Radio Frequency Magnetron Sputtering, J. Nanosci. Nanotechnol., 2016, 16, 10356–10360 CrossRef CAS
.
- S. Zhou, H. Jang, Q. Qin, L. Hou, M. G. Kim, S. Liu, X. Liu and J. Cho, Boosting Hydrogen Evolution Reaction by Phase Engineering and Phosphorus Doping on Ru/P-TiO2, Angew. Chem., Int. Ed., 2022, 61, e202212196 CAS
.
- X. Jiang, H. Jang, S. Liu, Z. Li, M. G. Kim, C. Li, Q. Qin, X. Liu and J. Cho, The Heterostructure of Ru2P/WO3/NPC Synergistically Promotes H2O Dissociation for Improved Hydrogen Evolution, Angew. Chem., 2021, 133, 4156–4162 CrossRef
.
- S. Ma, J. Deng, Y. Xu, W. Tao, X. Wang, Z. Lin, Q. Zhang, L. Gu and W. Zhong, Pollen-like self-supported FeIr alloy for improved hydrogen evolution reaction in acid electrolyte, J. Energy Chem., 2022, 66, 560–565 CrossRef CAS
.
- S. Shen, Z. Hu, H. Zhang, K. Song, Z. Wang, Z. Lin, Q. Zhang, L. Gu and W. Zhong, Highly Active Si Sites Enabled by Negative Valent Ru for Electrocatalytic Hydrogen Evolution in LaRuSi, Angew. Chem., Int. Ed., 2022, 61, e202206460 CAS
.
- Z. Lin, B. Xiao, M. Huang, L. Yan, Z. Wang, Y. Huang, S. Shen, Q. Zhang, L. Gu and W. Zhong, Realizing Negatively Charged Metal Atoms through Controllable d-Electron Transfer in Ternary Ir1−-xRhxSb Intermetallic Alloy for Hydrogen Evolution Reaction, Adv. Energy Mater., 2022, 12, 2200855 CrossRef CAS
.
|
This journal is © the Partner Organisations 2023 |