DOI:
10.1039/D2QI02599J
(Research Article)
Inorg. Chem. Front., 2023,
10, 2088-2099
Ionic Fe(III)-porphyrin frameworks for the one-pot synthesis of cyclic carbonates from olefins and CO2†
Received
5th December 2022
, Accepted 23rd February 2023
First published on 27th February 2023
Abstract
In this study, the rational construction of FeIII-centered porphyrin-based bifunctional ionic porous organic polymers (Fe-IPOP1/2) for a one-step, halogen-free, cascade transformation of olefins and CO2 to cyclic carbonates as compared to the conventional two-step process involving epoxides is presented. The ionic polymers, Fe-IPOP1/2 showed selective and recyclable uptake of CO2 with an interaction energy of 32.2/39.6 kJ mol−1 signifying the stronger interaction of carbon dioxide with the frameworks. Both the polymers were found to be thermally stable up to 300 °C and exhibited promising catalytic performance in the one-step, halogen-free synthesis of cyclic carbonates under eco-friendly, cocatalyst/solvent-free, atmospheric pressure conditions. The excellent catalytic activity of Fe-IPOP1/2 for a one-pot synthesis of cyclic carbonates has been ascribed to the presence of highly exposed oxophilic FeIII sites and nucleophilic Br− anions in the polymers. Notably, this one-pot synthesis strategy was extended for the transformation of various substituted olefins to their respective carbonates in good yield and selectivity. Further, Fe-IPOP1 showed good reusability with retention of catalytic activity for multiple cycles of usage.
Introduction
The major contributor to global climate destabilization is anthropogenic and naturally emitted carbon dioxide (CO2), whose concentration is increasing day by day exceeding 400 ppm, currently.1 This significant rise in CO2 concentration in the atmosphere has been attributed to greater consumption of fossil fuels leading to major environmental issues like unpredictable weather patterns, global warming, ocean acidification, and so on.2–4 To overcome this, carbon capture,5 storage, and utilization have been adopted globally to contain the increasing CO2 levels in the atmosphere.6–14 Given the plentiful amount of CO2 in the atmosphere, its use as a non-toxic, abundant, and cheap C1-feedstock for value-added chemical and fuel production has become a major topic of interest for many researchers worldwide.15–21 In this direction, numerous strategies have been used for the utilization of CO2 to obtain value-added chemicals.22–29 Among them, production of cyclic carbonates by the functionalization of epoxides using CO2 has gained special interest due to its absolute atom economy.30–35 Notably, cyclic carbonates are of potential utility as precursors of polymeric materials, raw materials of pharmaceuticals and cosmetics, electrolytes in batteries, and so on.36–39 Generally, cyclic carbonates have been synthesised by coupling CO2 with epoxides.40–43 However, a one-pot reaction utilizing readily available olefins and CO2 is an attractive eco-friendly strategy for the green synthesis of cyclic carbonates as compared to the conventional two-step process via the use of epoxides. Here, the catalyst employed needs to be highly reactive to catalyze the one-pot synthesis through the in situ formation of epoxides. For efficient, one-step transformation of olefins to cyclic carbonates, the catalyst should have a Lewis acidic, oxophilic metal ion such as Mn, Ti, Fe, Mo, etc. for the in situ formation of epoxides, which is favored due to the participation of the metal–oxo intermediate.44–47 In this direction, Fe-based catalysts have attracted significant interest due to their low cost, high abundance, and non-toxicity along with facile formation of FeIV
O active species during the reaction. More importantly, most of the catalysts reported for the synthesis of cyclic carbonates require an additional nucleophilic cocatalyst (halide ions) for facilitating ring-opening of the epoxide step. However, green chemistry practices demand that the synthesis be carried out under environment-friendly and halide-free mild conditions. In this regard, herein we report the strategic construction of bifunctional ionic porous organic polymers, Fe-IPOP1/2, by a polymerization reaction of FeIII–TPyP (TpyP = 5,10,15,20-tetrakis(4-pyridyl)-porphyrin) with 1,4-bis(bromomethyl)benzene/4,4′-bis(bromomethyl)biphenyl, respectively, under an inert atmosphere. Indeed, Fe-IPOP1/2 showed halogen-free CO2 fixation activity with olefins under environment-friendly mild conditions. Furthermore, the role of Lewis acidic/oxophilic FeIII sites was studied by preparing an analogous ionic polymer (Zn-IPOP1) using a Zn–TPyP metalloligand. Interestingly, Zn-IPOP1 did not show catalytic activity for the one-step synthesis of cyclic carbonates highlighting the need for oxophilic FeIII sites for the one-step cyclic carboxylation of olefins. Moreover, the role of nucleophilic Br− ions in halide-free cyclic carbonates synthesis was studied by preparing an analogous non-ionic polymer (Fe-POP1). Overall, this is a rare demonstration of the application of an ionic porphyrin-based polymer for the halogen-free utilization of CO2 in a one-pot reaction of CO2 with olefins.
Results and discussion
Synthesis and characterization
The 5,10,15,20-tetrakis(4′-pyridyl)porphyrin (TPyP) and metallated M-TPyP (M = ZnII, FeIII) ligand were prepared by following a reported procedure with a slight modification (see the ESI, Scheme S1†)48 and characterized by 1H NMR, UV-Vis, and FTIR analysis (Fig. S1–S4†). The metallated porphyrin-based ionic polymers (Zn/Fe-IPOP1) were synthesized by the polymerization reaction of Zn/Fe-TPyP with 1,4-bis(bromomethyl)benzene. However, Fe-IPOP2 was synthesized by reaction of Fe-TPyP with the 4,4′-bis(bromomethyl)biphenyl linker under an inert atmosphere as shown in Scheme 1. Furthermore, to test the role of nucleophilic Br− ions on the halogen-free preparation of cyclic carbonates, an analogous non-ionic polymer (Fe-POP) was synthesized (please refer to the ESI†).49
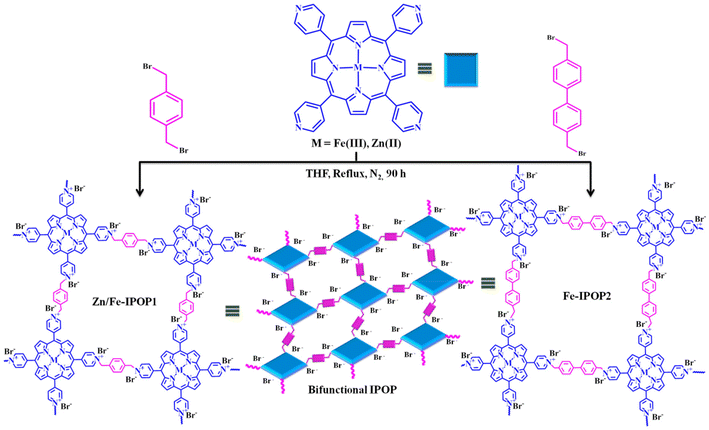 |
| Scheme 1 Synthesis scheme for Zn/Fe-IPOP1 and Fe-IPOP2. | |
The incorporation of a ZnII/FeIII ion into the porphyrin ring was confirmed by the complete absence of pyrrolic N–H peaks (δ = −2.83 ppm) of the porphyrin ring in the 1H NMR spectrum (Fig. S2†). In addition, FTIR spectra of the M–TPyP complex showed the absence of N–H stretching frequencies at 3306 cm−1 supporting the incorporation of metal ions (Fig. S3†). The UV-Vis spectra of the TPyP ligand showed an intense Soret band at 418 nm [a1u → eg] with four Q bands [a2u → eg (forbidden)] at 515, 550, 592, and 648 nm (Fig. S4†). The metallation of the TPyP ligand led to a redshift in the Soret band to 428 nm and also the number of Q bands was reduced to two (560 and 600 nm) due to the symmetry change of the molecule to D4h further supporting the metallation of the porphyrin ring (Fig. S4†).
The PXRD plots of Zn/Fe-IPOP1 and Fe-IPOP2 showed a broad peak in the 2θ region of 20–27° due to π–π stacking of porphyrin rings (Fig. S5†). New stretching frequencies at 1160 and 1629 cm−1 appeared in the FT-IR spectra, which were assigned to alkyl C–N and the pyridine iminium ion (–C
N+–), respectively, indicating the formation of an ionic polymer (Fig. S6 and S7†).48,50 Furthermore, an additional peak due to the aliphatic C–H stretching frequency at 2978 cm−1 supporting the presence of methylene groups in the polymer was observed (Fig. S6 and S7†).51 The formation of Zn-IPOP1 was also supported by solid-state 13C cross-polarized magic angle spinning (CP-MAS) NMR spectra (Fig. 1a). The methylene carbon (13CH2) linked to the quaternary pyridyl ammonium showed resonance at a chemical shift (δ) of 63 ppm (13Ca) supporting the formation of the ionic polymer. The porphyrin ring carbon linked to the pyridyl ring (13Cg) showed resonance peaks at δ 119 ppm while the pyridyl ring carbon (13Cb) connected to the porphyrin ring was observed at 152 ppm.52 The pyrrole ring carbons (13Cf and 13Cd) appear at δ 131 and 145 ppm, respectively. The carbons (13Ch and 13Cc) of the pyridyl ring appeared at 129 and 148 ppm, respectively.49 The phenyl ring carbon (13Ce) showed a resonance peak at 132 ppm.49
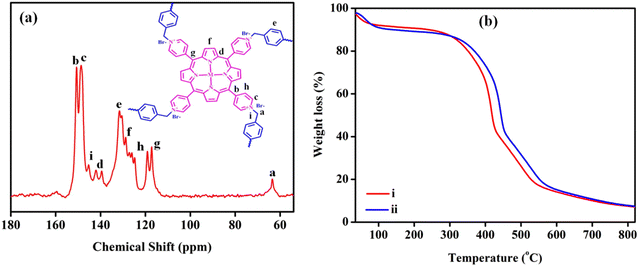 |
| Fig. 1 (a) 13C CP-MAS NMR spectra, and (b) TGA plot for Fe-IPOP1 (i) and Fe-IPOP2 (ii). | |
The thermal stability of the polymers was examined by thermogravimetric analysis (TGA). Fe-IPOP1/2 showed a weight loss of ∼11% in the temperature range between RT and 90 °C corresponding to the loss of adsorbed solvent (acetone) molecules (Fig. 1b). Both Fe-IPOPs exhibited thermal stability up to 300 °C, similar to that of Zn-IPOP1.49 Furthermore, SEM analysis showed spherical morphology for Fe-IPOP1/2 (Fig. S8†). The energy dispersive spectroscopy (EDS) analysis of Fe-IPOP1 revealed the presence of constituent elements in the polymer (Fig. S9†).
X-ray photoelectron spectroscopy (XPS) analysis of Fe-IPOP1/2 was performed to confirm the presence of their constituent elements. The survey spectra of Fe-IPOP1 confirmed the presence of elemental Fe, Cl, Br, C, and N (Fig. S10†). The Fe-spectra show peaks with binding energy (BE) values of 710.7 and 724.7 eV assigned to 2p3/2 and 2p1/2, respectively (Fig. 2a). Interestingly, the observed BE for FeIII matches well with the reported value for the FeIII-centered porphyrin ring, affirming the incorporation of FeIII in the porphyrin core.53,54 Furthermore, Cl spectra show BE peaks at 197.8 and 199.3 eV assigned to 2p3/2 and 2p1/2, which supports the coordination of the –Cl ion to the FeIII center (Fig. 2b).53 Furthermore, the N spectra showed two BE peaks at 398.3 and 400.6 eV due to pyrrolic nitrogen (NP1s) of the porphyrin ring and a quaternary nitrogen (NQ1s), respectively, which confirms the formation of the ionic polymer (Fig. 2c). The Br spectra show the appearance of two BE peaks at 68.2 and 69.1 eV assigned to 5d5/2 and 5d3/2, respectively (Fig. 2d). Similarly, XPS analysis of Fe-IPOP2 confirmed the presence of its constituent elements (Fig. S11†). The Fe spectra show BE peaks at 711.1 and 724.8 eV assigned to 2p3/2 and 2p1/2, respectively (Fig. S11†). However, the Cl spectra depict peaks at 197.9 and 199.5 eV, due to 2p3/2 and 2p1/2, respectively, and the N and Br spectra (Fig. S11†) are found to be similar to those of Fe-IPOP1 affirming the isostructural nature of Fe-IPOP1/2.
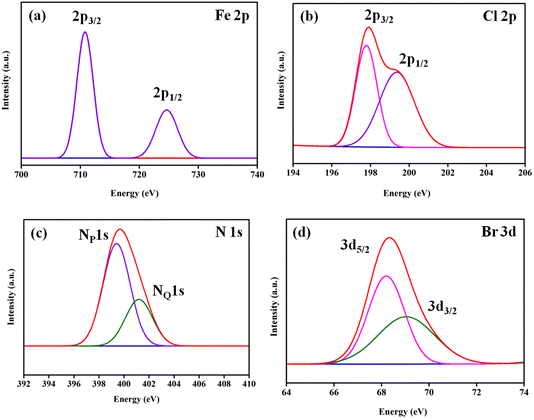 |
| Fig. 2 XPS of Fe-IPOP2: (a) Fe 2p, (b) Cl 2p, (c) N 1s, and (d) Br 3d spectra. | |
Gas adsorption studies
N2 adsorption of Fe-IPOP1/2 was measured to determine the porosity of the ionic polymers. The estimated BET surface area of Fe-IPOP1 (26.5 m2 g−1) was found to be in close agreement with the value reported for isostructural Zn-IPOP1 (16 m2 g−1) (Fig. S12†), whereas Fe-IPOP2 showed a relatively higher BET surface area of 77.5 m2 g−1 (Fig. S13†). Furthermore, pore size distribution analysis of Fe-IPOP1/2 revealed pore sizes of 6.1/8.3 Å, respectively. The relatively larger pore size in Fe-IPOP2 has been ascribed to the presence of the longer linker of 4,4′-bis(bromomethyl)biphenyl (7.2 Å) rather than 1,4-bis(bromomethyl)benzene (5.3 Å) used in Fe-IPOP1 (Fig. S14†). Furthermore, to test the CO2-philicity of Fe-IPOP1/2, gas sorption studies were carried out. As can be seen from Fig. 3, both Fe-IPOP1/2 showed type-I isotherms with uptake of 40.6/28.6 and 64.4/43.9 cc g−1 at 273 and 298 K, respectively (Fig. 3a and b). The relatively higher CO2 uptake of Fe-IPOP2 as compared to that of Fe-IPOP1 can be correlated to its higher surface area/porosity. Furthermore, the accurate prediction of CO2 uptake was carried out by adopting the Freundlich–Langmuir equation55 (Fig. S15 and S18†) and the adsorption energy (Qst) was determined from the Clausius–Clayperon equation.56 Interestingly, the Qst for CO2 with Fe-IPOP1/2 was calculated and found to be 32.2/39.6 kJ mol−1 signifying the stronger interaction of carbon dioxide with the frameworks (Fig. S19 and S20†). Additionally, selective gas sorption measurements (Fig. 3c) revealed negligible uptake of N2, and CH4 with relatively high Henry gas selectivity constants of 71 and 69 for KCO2/N2 and KCO2/CH4, respectively (Fig. S21†). Notably, Fe-IPOP1 showed recyclable CO2 adsorption properties (Fig. 3d). Furthermore, the basic and acidic strengths of Fe-IPOP1 quantified by CO2 and NH3 TPD (temperature-programmed desorption) analysis were found to be 0.46 and 0.53 mmol g−1, respectively (Fig. S22†). Thus, the TPD analysis revealed the bifunctional nature of Fe-IPOP1 having moderate basic and acidic sites, which is an essential requirement for effective transformation of CO2 under mild conditions.
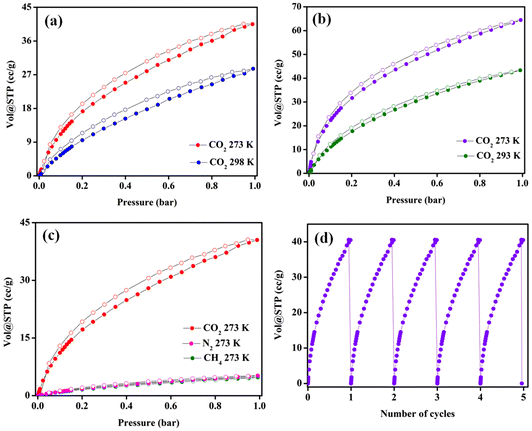 |
| Fig. 3 CO2 adsorption isotherms for (a) Fe-IPOP1 and (b) Fe-IPOP2. (c) Selective CO2 adsorption isotherm, and (d) recyclable CO2 adsorption for Fe-IPOP1. | |
Catalytic epoxidation reaction of olefins to epoxides
The presence of oxophilic/Lewis acidic FeIII and nucleophilic (Br−) sites in Fe-IPOP1/2 motivated us to test their catalytic performance for one-pot, halogen-free fixation of readily available olefins with CO2 to produce valuable feedstocks, that is cyclic carbonates. It is worth noting that the majority of reports on cyclic carbonates describe utilizing epoxides, which are prepared by the epoxidation of olefins catalyzed by Lewis acidic metal ions.57–61 Hence, to establish the formation of an epoxide as an intermediate en route to the formation of cyclic carbonates, the catalytic activity of Fe-IPOP1/2 was tested for the generation of epoxides from olefins using PhIO as an oxidizing agent at RT. The catalytic conditions were optimized using styrene as a model substrate by varying the reaction parameters (Table S1†). Notably, both Fe-IPOP1/2 catalyzed the epoxidation of styrene to yield styrene oxide (SO) with more than 99% yield within 18 h at RT with 100% selectivity (Table 1 and Fig. S23, S24†). Furthermore, to check the role of FeIII ions in catalyzing the epoxidation reaction, a control experiment was carried out using analogous Zn-centered porphyrin POP (Zn-IPOP1) as a catalyst. To our delight, negligible conversion (<5%) of styrene to SO was observed under the optimized conditions (Fig. S25†). This study highlights the importance of oxophilic FeIII ions for the oxidization of olefins to epoxides. Furthermore, the catalytic study was extended to the oxidation of various olefins including both substituted styrenes and linear alkenes (Table 1). Interestingly, most of the olefins were found to undergo conversion to their respective oxides with high yield and selectivity (Table 1).
Table 1 The catalytic activity of Fe-IPOP1/2 for epoxidation of olefinsa
One-step cyclic carbonate synthesis from olefins and CO2
The high catalytic activity of Fe-IPOP1/2 towards epoxidation of both aromatic and aliphatic olefins motivated us to test their catalytic performance for one-pot cyclic carbonate synthesis using readily accessible olefins and CO2 (Fig. 4a). To start with, the catalysis was performed using styrene as a model substrate and the reaction conditions were optimized by varying the temperature and time of reaction (Fig. 4 and Table S2†). Under the optimized reaction conditions, Fe-IPOP1 catalyzed the one-step oxidative carboxylation of styrene to styrene carbonate (SC) with >99% conversion within 24 h under halogen-free mild conditions of 1 atm of CO2 (Fig. 4 and S26, S27, Table S2†). Furthermore, controlled experiments performed using polymer precursors i.e. Fe-TPyP, 1,4-bis(bromomethyl)benzene, 4,4′-bis(bromomethyl)biphenyl and FeCl3 did not show styrene carbonate formation (Table S2†), highlighting the essential requirement of Fe-IPOP1 for generating cyclic carbonates from olefins and CO2. Thus, the control experiments revealed that for one-pot, halogen-free transformation of CO2 to cyclic carbonates both oxophilic metal and nucleophilic halogen sites are essential. In the case of Fe-TPyP and FeCl3 catalyzed reactions, although the presence of an oxophilic metal site is fulfilled, the nucleophilic Br− sites are absent, which are necessary for promoting ring-opening of the epoxides and therefore results in no formation of cyclic carbonates. Furthermore, the catalytic activity carried out with Fe-IPOP2 as the catalyst showed almost similar results to that of Fe-IPOP1 with >99% conversion of styrene to SC (Fig. S28†). The efficient halogen-free catalytic activity of Fe-IPOP1 was further extended for one-step cyclic carboxylation of various olefins including substituted styrenes and linear alkenes to their respective cyclic carbonates. Interestingly, Fe-IPOP1 catalyzed the transformation of a series of olefins to cyclic carbonates under halogen-free optimized conditions and the catalytic conversions are summarized in Table 2 (Fig. S29–S32†). The relatively lower conversion of long-chain linear alkenes, say 1-hexene (73%), 1-octene (69%), and 1-decene (64%), has been ascribed to their bulkiness and lower π-electron density.62 Notably, a comparison of the catalytic activity of Fe-IPOP1 with reported systems revealed its superior performance for one-step cyclic carbonate synthesis from readily accessible olefin and CO2 (Table 3).
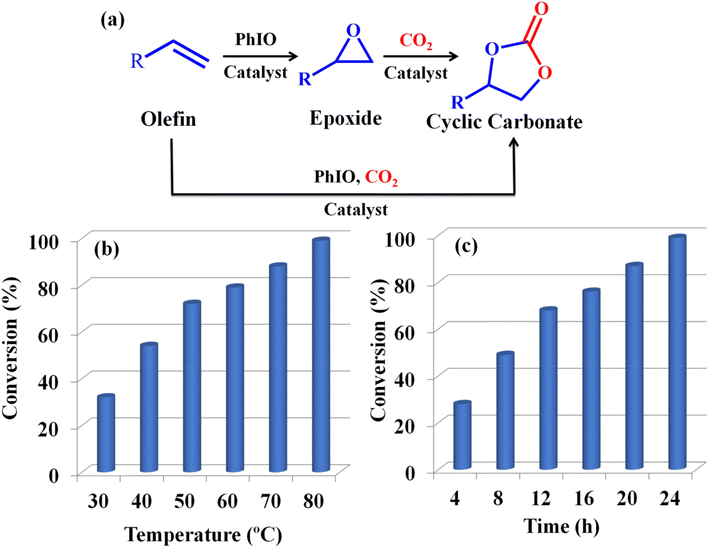 |
| Fig. 4 (a) Catalytic one-pot synthesis of cyclic carbonates. Optimization of the catalytic reaction by varying the temperature (b) and time (c). | |
Table 2 Catalytic one-step synthesis of cyclic carbonates from olefin and CO2a
Table 3 Comparison of catalytic activity of Fe-IPOP1 for one-step synthesis of styrene carbonates with that of literature-reported catalysts
S. no. |
Catalyst |
Co-catalyst |
Pressure |
Temperature (°C) |
Conversion (%) |
Ref. |
1 |
ZnW PYIs14 |
TBABr |
05 |
50 |
92 |
63
|
2 |
MOF-590 |
TBABr |
01 |
80 |
93 |
64
|
3 |
MNP@SiO2–8Mn |
PPNCl |
10 |
80 |
99 |
65
|
4 |
Ti-MMM-E |
TBABr |
08 |
70 |
92 |
66
|
5 |
FeIII@MOF1 |
TBABr |
08 |
80 |
98.6 |
67
|
6 |
MOF-892 |
TBABr |
01 |
80 |
51 |
68
|
7 |
ImBr-MOF-545(Mn) |
— |
05 |
70 |
99 |
59
|
8
|
Fe-IPOP1
|
Halogen free
|
01
|
80
|
99
|
This work
|
Mechanistic investigation for one-step cyclic carboxylation of olefins
As discussed in the aforementioned section, the one-step cyclic carbonate synthesis from olefins and CO2 proceeds through the formation of an epoxide intermediate. To establish the in situ formation of epoxide, the progress of the catalysis was monitored by a time-dependent 1H NMR study. As shown in Fig. 5, 1H NMR spectra of the aliquot taken at 6 h showed peaks corresponding to styrene oxide along with the product, styrene carbonate, and reactant, styrene (Fig. 5). Furthermore, as the reaction time increased to 18 h, the intensity of the peaks due to styrene oxide decreases and that of styrene carbonate increases, and at 24 h only peaks due to the SC are observed. This study unambiguously confirms the in situ generation of styrene oxide during the one-step cyclic carboxylation of olefins. Once the styrene oxide (epoxide) is formed, its coordination to a Lewis acidic (FeIII) site leads to the polarization of the epoxide into which insertion of a CO2 molecule takes place. Furthermore, to confirm the interaction of epoxide with the FeIII site, a control experiment was carried out in which Fe-POP1 catalyst was treated with epoxide (styrene oxide) for 2 h and then the catalyst was recovered and washed thoroughly with methanol followed by drying at 80 °C under vacuum. The FT-IR spectra of the recovered sample showed peaks due to styrene oxide, supporting the polarization of styrene oxide at FeIII sites (Fig. S33†). This step is followed by ring-opening of SO by nucleophilic Br− ions of the polymer. To support this step, an analogous polymer, Fe-POP, which lacks free Br− ions, was synthesized (Scheme S2†) and its catalytic performance tested in the one-step cyclic carbonate synthesis under identical conditions.48 To our delight, only styrene oxide (>99) formation was observed as opposed to styrene carbonate formation as in the case of Fe-POP1 (Table 2). However, the addition of a cocatalyst (2.5 mol% TBABr) to the reaction led to the formation of styrene carbonate. These studies brought out the essential requirement of a bifunctional catalyst composed of acidic and nucleophilic sites to achieve a one-step synthesis of cyclic carbonates under halogen-free conditions.
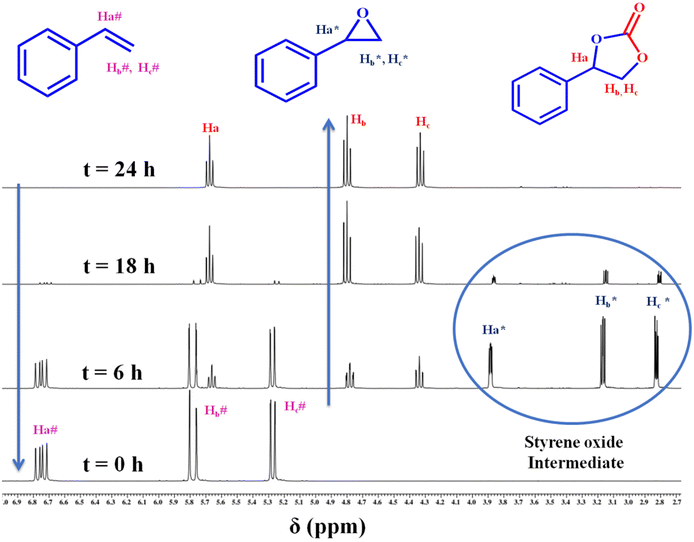 |
| Fig. 5 Time-dependent 1H NMR (400 MHz, CDCl3, 20 °C) spectra for one-pot styrene carbonate synthesis. | |
Based on the above discussion, the mechanism for the one-step cascade reaction of CO2 with styrene catalyzed by a bifunctional Fe-POP1 catalyst is shown in Scheme 2. The direct carboxylation of styrene proceeds with the in situ generation of styrene oxide by the oxidation of styrene. Then polarization of styrene oxide at the FeIII sites, followed by its ring-opening upon nucleophilic attack of a Br− anion, results in a bromo-alkoxide intermediate. Then the subsequent addition of CO2 produces metal carbonate species and its ring-closure reaction leads to styrene carbonate and its elimination regenerates the catalyst.
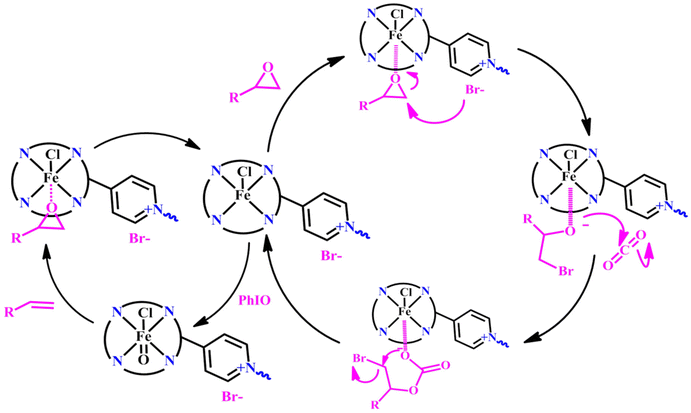 |
| Scheme 2 Plausible mechanism for catalytic one-pot reaction of CO2 with an olefin to generate cyclic carbonates. | |
Recyclability and catalyst leaching test
Chemical stability and reusability are essential requirements for a heterogeneous catalyst. The reusability of Fe-IPOP1 was investigated by isolating it from the reaction mixture followed by activation at 100 °C for 12 h. It is important to note that Fe-IPOP1 was reusable for eight cycles with no significant loss of activity (Fig. 6a and S34†). Additionally, PXRD, FTIR, and XPS analyses of the regenerated polymer confirm its structural stability (Fig. S5, S35 and S36†). Furthermore, the BET surface area (22.3 m2 g−1) of the recycled sample was found to be almost close to that of the as-synthesized sample (26.5 m2 g−1), supporting retention of the original network structure even after eight catalytic cycles. To exclude any leaching of active metal (FeIII) into the homogeneous system, the reaction was halted after 8 h and the catalyst was separated by filtration, and the reaction was continued for some more time. Surprisingly, no discernible rise in conversion was detected, indicating the absence of leaching of active species into solution (Fig. 6b). Furthermore, MP-AES analysis of the reaction filtrate showed no detectable FeIII ions in the solution, which rule out any leaching during catalysis (Fig. S37†).
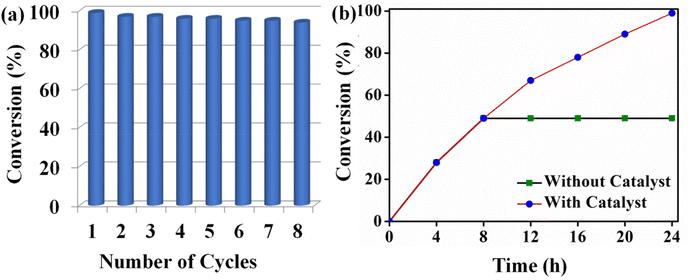 |
| Fig. 6 (a) Catalyst reusability and (b) leaching test. | |
Conclusion
In summary, a strategic design of bifunctional Fe-porphyrin-based ionic POP by integrating oxophilic Fe sites and nucleophilic Br− anions essential for the one-pot synthesis of cyclic carbonates under eco-friendly and halogen-free conditions is presented. The role of oxophilic and nucleophilic sites in catalytic activity was established. Importantly, Fe-IPOP1 showed excellent reusability with retention of catalytic performance and structural integrity. This work constitutes the first demonstration of an ionic Fe-porphyrin polymer-catalyzed one-pot synthesis of cyclic carbonates from readily accessible olefins and carbon dioxide.
Conflicts of interest
The authors declare no conflict of interest.
Acknowledgements
CMN thanks the Science & Engineering Research Board (SERB), Department of Science and Technology, Govt. of India, for financial support (CRG/2018/001176). R. D. and S. K. thank IIT Ropar for their fellowships.
References
- F. Joos, G.-K. Plattner, T. F. Stocker, O. Marchal and A. Schmittner, Global Warming and Marine Carbon Cycle Feedbacks on Future Atmospheric CO2, Science, 1999, 284, 464–467 CrossRef CAS PubMed.
- M. Z. Jacobson, Review of solutions to global warming, air pollution, and energy security, Energy Environ. Sci., 2009, 2, 148–173 RSC.
- H. D. Matthews, T. L. Graham, S. Keverian, C. Lamontagne, D. Seto and T. J. Smith, National contributions to observed global warming, Environ. Res. Lett., 2014, 9, 014010 CrossRef CAS.
- W. D. Jones, Carbon Capture and Conversion, J. Am. Chem. Soc., 2020, 142, 4955–4957 CrossRef CAS PubMed.
- M. Wilson, S. N. Barrientos-Palomo, P. C. Stevens, N. L. Mitchell, G. Oswald, C. M. Nagaraja and J. P. S. Badyal, Substrate-Independent Epitaxial Growth of the Metal-Organic Framework MOF-508a, ACS Appl. Mater. Interfaces, 2018, 10, 4057–4065 CrossRef CAS PubMed.
- R. Das and C. M. Nagaraja, Noble metal-free Cu(I)-anchored NHC-based MOF for highly recyclable fixation of CO2 under RT and atmospheric pressure conditions, Green Chem., 2021, 23, 5195–5204 RSC.
- A. Katelhon, R. Meys, S. Deutz, S. Suh and A. Bardow, Climate change mitigation potential of carbon capture and utilization in the chemical industry, Proc. Natl. Acad. Sci. U. S. A., 2019, 116, 11187–11194 CrossRef CAS PubMed.
- C. M. Nagaraja, R. Haldar, T. K. Maji and C. N. R. Rao, Chiral Porous Metal-Organic Frameworks of Co(II) & Ni(II): Synthesis, Structure, Magnetic Properties and CO2 Uptake, Cryst. Growth Des., 2012, 12, 975 CrossRef CAS.
- Q. Liu, L. Wu, R. Jackstell and M. Beller, Using carbon dioxide as a building block in organic synthesis, Nat. Commun., 2015, 6, 5933 CrossRef PubMed.
- R. Das, T. Ezhil, A. S. Palakkal, D. Muthukumar, R. S. Pillai and C. M. Nagaraja, Efficient chemical fixation of CO2 from direct air under environment-friendly co-catalyst and solvent-free ambient conditions, J. Mater. Chem. A, 2021, 9, 23127–23139 RSC.
- T. Sakakura, J. C. Choi and H. Yasuda, Transformation of Carbon Dioxide, Chem. Rev., 2007, 107, 2365–2387 CrossRef CAS PubMed.
- S. C. Peter, Reduction of CO2 to Chemicals and Fuels: A Solution to Global Warming and Energy Crisis, ACS Energy Lett., 2018, 3, 1557–1561 CrossRef CAS.
- R. Das, S. S. Manna, B. Pathak and C. M. Nagaraja, Strategic Design of Mg-Centered Porphyrin Metal-Organic Framework for Efficient Visible Light-Promoted Fixation of CO2 under Ambient Conditions: Combined Experimental and Theoretical Investigation, ACS Appl. Mater. Interfaces, 2022, 14, 33285–33296 CrossRef CAS PubMed.
- T. K. Pal, D. De and P. K. Bharadwaj, Metal-organic frameworks as heterogeneous catalysts for the chemical conversion of carbon dioxide, Fuel, 2022, 320, 123904 CrossRef CAS.
- W. D. Jones, Carbon Capture and Conversion, J. Am. Chem. Soc., 2020, 142, 4955–4957 CrossRef CAS PubMed.
- A. Katelhon, R. Meys, S. Deutz, S. Suh and A. Bardow, Climate change mitigation potential of carbon capture and utilization in the chemical industry, Proc. Natl. Acad. Sci. U. S. A., 2019, 116, 11187–11194 CrossRef CAS PubMed.
- B. Ugale, S. Kumar, T. J. D. Kumar and C. M. Nagaraja, Environmentally Friendly, Co-catalyst-Free Chemical Fixation of CO2 at Mild Conditions Using Dual-Walled Nitrogen-Rich Three Dimensional Porous Metal–Organic Frameworks, Inorg. Chem., 2019, 58, 3925–3936 CrossRef CAS PubMed.
- Q. Liu, L. Wu, R. Jackstell and M. Beller, Using carbon dioxide as a building block in organic synthesis, Nat. Commun., 2015, 6, 5933 CrossRef PubMed.
- T. Sakakura, J. C. Choi and H. Yasuda, Transformation of Carbon Dioxide, Chem. Rev., 2007, 107, 2365–2387 CrossRef CAS PubMed.
- R. Das, S. S. Dhankhar and C. M. Nagaraja, Construction of a bifunctional Zn(II)-organic framework containing basic amine functionality for selective capture and room temperature fixation of CO2, Inorg. Chem. Front., 2020, 7, 72–81 RSC.
- Y.-H. Zou, Y.-B. Huang, D.-H. Si, Q. Yin, Q.-J. Wu, Z. Weng and R. Cao, Porous Metal-Organic Framework Liquids for Enhanced CO2 Adsorption and Catalytic Conversion, Angew. Chem., Int. Ed., 2021, 60, 20915 CrossRef CAS PubMed.
- X. L. Meng, Y. Nie, J. Sun, W. G. Cheng, J. Q. Wang, H. Y. He and S. J. Zhang, Superbase/cellulose: an environmentally benign catalyst for chemical fixation of carbon dioxide into cyclic carbonates, Green Chem., 2014, 16, 2771–2778 RSC.
- R. Das, V. Parihar and C. M. Nagaraja, Strategic design of a bifunctional Ag(I)-grafted NHC-MOF for efficient chemical fixation of CO2 from a dilute gas under ambient conditions, Inorg. Chem. Front., 2022, 9, 2583–2593 RSC.
- Y. Mitsuka, N. Ogiwara, M. Mukoyoshi, H. Kitagawa, T. Yamamoto, T. Toriyama, S. Matsumura, M. Haneda, S. Kawaguchi, Y. Kubota and H. Kobayashi, Fabrication of Integrated Copper-Based Nanoparticles/Amorphous Metal–Organic Framework by a Facile Spray-Drying Method: Highly Enhanced CO2 Hydrogenation Activity for Methanol Synthesis, Angew. Chem., Int. Ed., 2021, 60, 22283–22288 CrossRef CAS PubMed.
- M. SK, S. Barman, S. Paul, R. De, S. S. Sreejith, H. Reinsch, M. Grzywa, N. Stock, D. Volkmer, S. Biswas and S. Roy, An Anthracene-Based Metal-Organic Framework for Selective Photo-Reduction of Carbon Dioxide to Formic Acid Coupled with Water Oxidation, Chem. – Eur. J., 2021, 27, 4098–4107 CrossRef CAS PubMed.
- Y. Gu, M. Tamura, Y. Nakagawa, K. Nakao, K. Suzukic and K. Tomishige, Direct synthesis of polycarbonate diols from atmospheric flow CO2 and diols without using dehydrating agents, Green Chem., 2021, 23, 5786–5796 RSC.
- Q.-J. Wu, J. Liang, Y.-B. Huang and R. Cao, Thermo-, Electro-, and Photocatalytic CO2 Conversion to Value Added Products over Porous Metal/Covalent Organic Frameworks, Acc. Chem. Res., 2022, 55, 2978–2997 CrossRef CAS PubMed.
- J. Liang, R.-P. Chen, X.-Y. Wang, T.-T. Liu, X.-S. Wang, Y.-B. Huang and R. Cao, Postsynthetic ionization of an imidazolecontaining metal-organic framework for the cycloaddition of carbon dioxide and epoxides, Chem. Sci., 2017, 8, 1570–1575 RSC.
- C. He, J. Liang, Y.-H. Zou, J.-D. Yi, Y.-B. Huang and R. Cao, Metal-organic frameworks bonded with metal N-heterocyclic carbenes for efficient catalysis, Natl. Sci. Rev., 2022, 9, 157 CrossRef PubMed.
- J. Liang, Y.-B. Huang and R. Cao, Metal–organic frameworks and porous organic polymers for sustainable fixation of carbon dioxide into cyclic carbonates, Coord. Chem. Rev., 2019, 378, 32–65 CrossRef CAS.
- R. Das, D. Muthukumar, R. S. Pillai and C. M. Nagara, Rational Design of a ZnII MOF with Multiple Functional Sites for Highly Efficient Fixation of CO2 under Mild Conditions: Combined Experimental and Theoretical Investigation, Chem. – Eur. J., 2020, 26, 17445–17454 CrossRef CAS PubMed.
- M. Ding, R. W. Flaig, H.-L. Jiang and O. M. Yaghi, Carbon capture and conversion using metal–organic frameworks and MOF-based materials, Chem. Soc. Rev., 2019, 48, 2783–2828 RSC.
- S. S. Dhankhar, B. Ugale and C. M. Nagaraja, Co-Catalyst-Free Chemical Fixation of CO2 into Cyclic Carbonates by using Metal-Organic Frameworks as Efficient Heterogeneous Catalysts, Chem. – Asian J., 2020, 15, 2403–2427 CrossRef PubMed.
- T. K. Pal, D. De and P. K. Bhardwaj, Metal–organic frameworks for the chemical fixation of CO2 into cyclic carbonates, Coord. Chem. Rev., 2020, 408, 213173 CrossRef CAS.
- R. Das and C. M. Nagaraja, Highly Efficient Fixation of Carbon
Dioxide at RT and Atmospheric Pressure Conditions: Influence of Polar Functionality on Selective Capture and Conversion of CO2, Inorg. Chem., 2020, 59, 9765–9773 CrossRef CAS PubMed.
- B. Scrosati, J. Hassounab and Y.-K. Sun, Lithium-ion batteries. A look into the future, Energy Environ. Sci., 2011, 4, 3287–3295 RSC.
- B. Grignard, S. Gennen, C. Jérôme, A. W. Kleij and C. Detrembleur, Advances in the use of CO2 as a renewable feedstock for the synthesis of polymers, Chem. Soc. Rev., 2019, 48, 4466–4514 RSC.
- H. Liu, S. Lin, Y. Feng and P. Theato, CO2-Responsive polymer materials, Polym. Chem., 2017, 8, 12–23 RSC.
- S. Fukuoka, M. Kawamura, K. Komiya, M. Tojo, H. Hachiya, K. Hasegawa, M. Aminaka, H. Okamoto, I. Fukawad and S. Konno, A novel non-phosgene polycarbonate production process using by-product CO2 as starting material, Green Chem., 2003, 5, 497–507 RSC.
- S. Liu, H. Chen and X. Zhang, Bifunctional {Pb10K2}–Organic Framework for High Catalytic Activity in Cycloaddition of CO2 with Epoxides and Knoevenagel Condensation, ACS Catal., 2022, 12, 10373–10383 CrossRef CAS.
- Y. B. N. Tran, P. T. K. Nguyen, Q. T. Luong and K. D. Nguyen, Series of M-MOF-184 (M = Mg, Co, Ni, Zn, Cu, Fe) Metal-Organic Frameworks for Catalysis Cycloaddition of CO2, Inorg. Chem., 2020, 59, 16747–16759 CrossRef CAS PubMed.
- G. Zhai, Y. Liu, L. Lei, J. Wang, Z. Wang, Z. Zheng, P. Wang, H. Cheng, Y. Dai and B. Huang, Light-Promoted CO2 Conversion from Epoxides to Cyclic Carbonates at Ambient Conditions over a Bi-Based Metal-Organic Framework, ACS Catal., 2021, 11, 1988–1994 CrossRef CAS.
- R. Das, T. Ezhil and C. M. Nagaraja, Design of Bifunctional Zinc(II)–Organic Framework for Efficient Coupling of CO2 with Terminal/Internal Epoxides under Mild Conditions, Cryst. Growth Des., 2022, 22, 598–607 CrossRef CAS.
- G. De Faveri, G. Ilyashenko and M. Watkinson, Recent advances in catalytic asymmetric epoxidation using the environmentally benign oxidant hydrogen peroxide and its derivatives, Chem. Soc. Rev., 2011, 40, 1722–1760 RSC.
- N. Anbazhagan, G. Imran, A. Qurashi, A. Pandurangan and S. Manimaran, Confinement of Mn3+ redox sites in Mn-KIT-6 and its catalytic activity for styrene epoxidation, Microporous Mesoporous Mater., 2017, 247, 190–197 CrossRef CAS.
- S. Rayati, P. Nafarieh and M. M. Amini, The synthesis, characterization and catalytic application of manganese porphyrins bonded to novel modified SBA-15, New J. Chem., 2018, 42, 6464–6471 RSC.
- K. Zhang, O. K. Farha, J. T. Hupp and S. T. Nguyen, Complete double epoxidation of divinylbenzene using Mn(porphyrin)-based porous organic polymers, ACS Catal., 2015, 5, 4859–4866 CrossRef CAS.
- N. Sharma, S. S. Dhankhar and C. M. Nagaraja, Environment-friendly, co-catalyst and solvent-free fixation of CO2 using an ionic Zinc(II)-porphyrin complex immobilized in porous metal-organic framework, Sustainable Energy Fuels, 2019, 3, 2977–2982 RSC.
- X. Yao, K. Chen, L. Qiu, Z. Yang and L. He, Ferric Porphyrin-Based Porous Organic Polymers for CO2 Photocatalytic Reduction to Syngas with Selectivity Control, Chem. Mater., 2021, 33, 8863–8872 CrossRef CAS.
- B. Ugale, S. S. Dhankhar and C. M. Nagaraja, Exceptionally stable and 20-connected lanthanide metal-organic frameworks (MOFs) for selective CO2 capture and conversion to cyclic carbonates at atmospheric pressure, Cryst. Growth Des., 2018, 18, 2432–2440 CrossRef CAS.
- D. Chakraborty, S. Nandi, M. A. Sinnwell, J. Liu, R. Kushwaha, P. K. Thallapally and R. Vaidhyanathan, Hyper-Cross-linked Porous Organic Frameworks with Ultramicropores for Selective Xenon Capture, ACS Appl. Mater. Interfaces, 2019, 11, 13279–13284 CrossRef CAS PubMed.
- S. Jayakumar, H. Li, J. Chen and Q. Yang, Cationic Zn-Porphyrin Polymer Coated onto CNTs as a Cooperative Catalyst for the Synthesis of Cyclic Carbonates, ACS Appl. Mater. Interfaces, 2018, 10, 2546–2555 CrossRef CAS PubMed.
- B.-X. Dong, S.-L. Qian, F.-Y. Bu, Y.-C. Wu, L.-G. Feng, Y.-L. Teng, W.-L. Liu and Z.-W. Li, Electrochemical Reduction of CO2 to CO by a Heterogeneous Catalyst of Fe-Porphyrin-Based Metal–Organic Framework, ACS Appl. Energy Mater., 2018, 1, 4662–4669 CrossRef CAS.
- I. Liberman, R. Shimoni, R. Ifraemov, I. Rozenberg, C. Singh and I. Hod, Active-Site Modulation in an Fe-Porphyrin-Based Metal–Organic Framework through Ligand Axial Coordination: Accelerating Electrocatalysis and Charge-Transport Kinetics, J. Am. Chem. Soc., 2020, 142, 1933–1940 CrossRef CAS PubMed.
-
R. T. Yang, Gas Separation by Adsorption Processes, Butterworth, Boston, 1997 Search PubMed.
- H. Pan, J. A. Ritter and P. B. Balbuena, Examination of the Approximations Used in Determining the Isosteric Heat of Adsorption from the Clausius-Clapeyron Equation, Langmuir, 1998, 14, 6323–6327 CrossRef CAS.
- B. Ugale, S. S. Dhankhar and C. M. Nagaraja, Construction of 3-Fold-Interpenetrated Three-Dimensional Metal–Organic Frameworks of Nickel(II) for Highly Efficient Capture and Conversion of Carbon Dioxide, Inorg. Chem., 2016, 55, 9757–9766 CrossRef CAS PubMed.
- Y. Gu, B. A. Anjali, S. Yoon, Y. Choe, Y. G. Chung and D.-W. Park, Defect-engineered MOF-801 for cycloaddition of CO2 with epoxides, J. Mater. Chem. A, 2022, 10, 10051–10061 RSC.
- G. Singh and C. M. Nagaraja, Highly efficient metal/solvent-free chemical fixation of CO2 at atmospheric pressure conditions using functionalized porous covalent organic frameworks, J. CO2 Util., 2021, 53, 101716 CrossRef CAS.
- J. F. Kurisingal, Y. Rachuri, A. S. Palakkal, R. S. Pillai, Y. Gu, Y. Choe and D.-W. Park, Water-Tolerant DUT-Series Metal-Organic Frameworks: A Theoretical-Experimental Study for the Chemical Fixation of CO2 and Catalytic Transfer Hydrogenation of Ethyl Levulinate to γ-Valerolactone, ACS Appl. Mater. Interfaces, 2019, 11, 41458–41471 CrossRef CAS PubMed.
- S. S. Dhankhar, R. Das, B. Ugale, R. S. Pillai and C. M. Nagaraja, Chemical Fixation of CO2 Under Solvent and Co-Catalyst-free Conditions Using a Highly Porous Two-fold Interpenetrated Cu(II)-Metal-Organic Framework, Cryst. Growth Des., 2021, 21, 1233–1241 CrossRef CAS.
- K. Yu, P. Puthiaraj and W.-S. Ahn, One-pot catalytic transformation of olefins into cyclic carbonates over an imidazolium bromide-functionalized Mn(III)-porphyrin metal–organic framework, Appl. Catal., B, 2020, 273, 119059 CrossRef CAS.
- Q. Han, B. Qi, W. Ren, C. He, J. Niu and C. Duan, Polyoxometalate-based homochiral metal-organic frameworks for tandem asymmetric transformation of cyclic carbonates from olefins, Nat. Commun., 2015, 6, 10007 CrossRef PubMed.
- H. T. D. Nguyen, Y. B. N. Tran, H. N. Nguyen, T. C. Nguyen, F. Gandara and P. T. K. Nguyen, A Series of Metal–Organic Frameworks for Selective CO2 Capture and Catalytic Oxidative Carboxylation of Olefins, Inorg. Chem., 2018, 57, 13772–13782 CrossRef CAS PubMed.
- L. D. Dias, R. M. B. Carrilho, C. A. Henriques, M. J. F. Calvete, A. M. Masdeu-Bultj, C. Claver, L. M. Rossi and M. M. Pereira, Hybrid Metalloporphyrin Magnetic Nanoparticles as Catalysts for Sequential Transformation of Alkenes and CO2 into Cyclic Carbonates, ChemCatChem, 2018, 10, 2792–2803 CrossRef CAS.
- N. V. Maksimchuka, I. D. Ivanchikovaa, A. B. Ayupova and O. A. Kholdeeva, One-step solvent-free synthesis of cyclic carbonates by oxidative carboxylation of styrenes over a recyclable Ti-containing catalyst, Appl. Catal., B, 2016, 181, 363–370 CrossRef.
- N. Sharma, S. S. Dhankhar, S. Kumar, T. J. D. Kumar and C. M. Nagaraja, Rational Design of a 3D MnII-Metal-Organic Framework Based on a Nonmetallated Porphyrin Linker for Selective
Capture of CO2 and One-Pot Synthesis of Styrene Carbonates, Chem. – Eur. J., 2018, 24, 16662–16669 CrossRef CAS PubMed.
- P. T. K. Nguyen, H. T. D. Nguyen, H. N. Nguyen, C. A. Trickett, Q. T. Ton, E. Gutierrez-Puebla, M. A. Monge, K. E. Cordova and F. Gandara, New Metal-Organic Frameworks for Chemical Fixation of CO2, ACS Appl. Mater. Interfaces, 2018, 10, 733–744 CrossRef CAS PubMed.
Footnote |
† Electronic supplementary information (ESI) available: Synthesis procedures, FTIR plots, UV-Vis spectra, and 1H NMR spectra of the catalytic reactions. See DOI: https://doi.org/10.1039/d2qi02599j |
|
This journal is © the Partner Organisations 2023 |