DOI:
10.1039/D2PY00833E
(Paper)
Polym. Chem., 2023,
14, 62-70
Colorless polyimides derived from norbornyl bis-benzocyclobutene-containing diamines†
Received
27th June 2022
, Accepted 7th November 2022
First published on 19th November 2022
Abstract
Two diamines containing norbornyl bis-benzocyclobutene (N2BC) units (CANAL-2 and CANAL-4) were synthesized by catalytic norbornene–arene annulation reactions between bromoanilines and norbornadiene, and the regioisomers of CANAL-4 were separated using column chromatography. Two sets of colorless polyimides (CPIs) were then prepared via a traditional one-step method using CANAL diamines and commercial dianhydrides as the monomers. The N2BC-containing CPIs displayed exceptionally high glass transition temperatures (Tg) of 418–480 °C, good optical properties (61–84% transmittance at 400 nm), and modest coefficients of thermal expansion (CTE) as low as 23.9 ppm K−1. For the given dianhydrides, the CPIs from CANAL-4 demonstrated similar lower interchain distances, lower Tg, lower CTE, and better mechanical properties compared to the CANAL-2-derived ones. These differences can be explained by the reduced rotational barrier but more pronounced inter- and intra-molecular interactions originating from their less ortho-methyl substituents. The CPIs from anti-CANAL-4 exhibited more compact chain packing and thus better dimensional stability as compared to those from syn-CANAL-4. For certain diamines, the CPIs from norbornane-2-spiro-α-cyclopentanone-α′-spiro-2′′-norbornane-5,5′′,6,6′′-tetracarboxylic dianhydride (CpODA) showed the best integrated properties due to the rigid and contorted CpODA residues, and additional dipole–dipole interactions of the ketone moieties. These CPIs show great promise as candidate materials for optical and optoelectronic applications because of their excellent combination of light coloration, and balanced thermal and mechanical properties.
Introduction
Colorless polyimides (CPI) have been attracting considerable attention from both academia and industry due to their many distinctive advantages, such as superior heat resistance, excellent optical transparency, low density, inherent flexibility, and ease of processing.1,2 Consequently, CPIs have found numerous applications in the field of flexible displays, transparent flexible printing circuits, organic photovoltaics, and other optical and optoelectronic devices, replacing inorganic glass and conventional yellowish polyimides.3–6 The low optical transmittance and deep color of traditional polyimides are attributable to their conjugated aromatic backbones, and formation of charge transfer complexes (CTCs). Thus, the optical properties of polyimides could be improved through either breaking electronic conjugation or suppressing CTC formation. Effective methodologies for structural manipulation include the adoption of fluorinated or alicyclic monomers. However, the improvement in optical properties is usually accompanied by the loss in thermal stability and mechanical robustness. It remains a challenge to prepare CPIs with high glass transition temperatures (Tg) (>400 °C) and excellent optical properties.1,2,7–10
The introduction of alicyclic moieties into polyimide backbones can disrupt chain conjugation, increase interchain distances, and weaken or even erase CTC formation due to their non-coplanar architectures, as well as reduced electron-withdrawing or electron-donating capability. Thus, non-aromatic dianhydrides and diamines, including cyclobutane-1,2,3,4-tetracarboxylic dianhydride (CBDA), cyclohexanetetracarboxylic dianhydride (HPMDA), and dicyclohexyl-tetracarboxylic dianhydride (HBPDA), have been intensively exploited for CPI synthesis.7,10–22 Polyimides derived from CBDA exhibit high Tg, low CTE, and favourable optical transmittance due to their excellent structural rigidity and linearity. However, their solubility in organic solvents is limited, and they also display low film toughness, with elongation-at-break values <10%.13–16 In contrast, the HPMDA- and HBPDA-derived CPIs exhibit good solubility and high elongation-at-break. Nonetheless, their Tg and dimensional stability are generally insufficient for practical applications due to the lack of structural rigidity.17–20 Thus, polycyclic dianhydrides and diamines have been developed to increase the chain rigidity of CPIs.23–30 For example, Matsumoto reported a series of alicyclic dianhydrides with norbornanedicarboxylic anhydride (NCA) segments, and the resulting CPIs displayed extremely high Tg and excellent optical properties.23–27 In particular, the combination of CpODA with aromatic diamines can generate CPIs with Tg values >400 °C and CTE as low as 13 ppm K−1.23 Furthermore, our group reported CPIs made from 2R,5R,7S,10S-naphthanetetracarboxylic dianhydride, which displayed high Tg (312–418 °C) and favorable mechanical properties.28 Polycyclic diamines were also exploited for CPI synthesis.29,30 Our group developed CPIs made from adamantane-containing diamines and alicyclic dianhydrides. These CPIs displayed Tg values of 285–440 °C, optical transmittance values >80% at 400 nm, and favorable thermal stability.29 Ando et al. prepared CPIs through the Tröger's base (TB) formation reaction of imide-containing diamines derived from HPMDA, CBDA, and bicyclo[2.2.2]oct-7-ene-2,3,5,6-tetracarboxylic dianhydride (BTA). The resulting CPIs possessed a CTE of 39–47 ppm K−1, cut-off wavelength (λcut–off) of around 300 nm, and initial modulus of 0.8–1.2 GPa.30
The N2BC moiety has a unique W-shaped architecture with two benzocyclobutene rings fused with one norbornyl ring.31–36 Xia et al. developed an efficient approach to synthesizing N2BC-containing polymers (also known as CANAL polymers) through catalytic norbornene–arene annulation (CANAL) reactions between aryl dibromides and 2,5-norbornadiene (NBD), using palladium acetate (Pd(OAc)2)/triphenylphosphine (Ph3P) as the catalyst and caesium carbonate (Cs2CO3) as the base. The side reactions were suppressed by blocking other reaction sites in aryl dibromides, and stereo-selective exo-isomers were formed in high yield (>90%) at a low catalyst loading of 1 mol%.31 CANAL reactions are compatible with various functional groups, including amines, chlorides, ethers, and esters. Thus, a large variety of CANAL polymers have been prepared, and they showed high surface areas, good solubility in organic solvents, and excellent gas separation performance due to their rigid and contorted architectures.32–36 N2BC-containing diamines can also be synthesized from bromo-anilines and NBD. The TB polymerization of N2BC-containing diamines produced ladder-type CANAL-TB polymers, which exhibited extremely high surface areas (up to 987 m2 g−1), and consequently an O2 permeability of 200–500 barrer and modest O2/N2 selectivity of 4.6–5.2, exceeding the 2008 Robeson's upper bound.37,38 N2BC-containing diamines were also combined with 4,4′-(hexafluoroisopropylidene)diphthalic anhydride (6FDA) to produce microporous polyimides. The resulting polymers displayed surface areas of 200–500 m2 g−1, H2 permeability of up to 1100 barrer, and reasonable H2/CH4 and H2/N2 selectivity, approaching the corresponding 2008 Robeson's upper bounds.39
So far, the research regarding N2BC-containing polymers is concentrated on the syntheses of polymers with diverse architectures, and the assessment of their gas separation performance. Herein, a series of N2BC-containing CPIs with exceptionally high Tg and outstanding optical properties are reported. Two N2BC-containing diamines (CANAL-2 and CANAL-4) were prepared through the CANAL reactions of NBD and 3-bromo-2-methylaniline or 3-bromo-2,6-dimethylaniline, respectively, and CPIs were then prepared using these diamines and 6FDA or alicyclic dianhydrides. The thermal, mechanical, and optical properties of the resultant CPIs were systematically investigated, and their structure–property relationship was discussed.
Results and discussion
Monomer syntheses
CANAL-2 and CANAL-4 were prepared according to a literature method developed by Xia et al. (Scheme 1).37–39 Methyl substituents ortho to the bromides are essential to achieve these diamines with high yields since they can facilitate the formation of a palladacycle and block other possible reaction sites.31,37–39 Two regioisomers (syn and anti) existed for each diamine due to different spatial arrangements of methyl and amino groups.37,38 This observation was confirmed by their NMR spectra and melting points (Fig. 1 and S1, S2†). The melting points of these diamines were within a range of 10 °C, indicative of the existence of regioisomers. In the 1H NMR spectrum of CANAL-4 (Fig. 1A), the signals at 6.52 and 6.42 ppm (peaks 1 and 2) represented the aromatic protons, whereas the signals at 3.03, 2.16, 1.88, and 0.62 (peaks 4, 5, 6, 7, and 8) were assigned to the aliphatic protons in the norbornyl and benzylmethyl segments. Three peaks were detected for the bridgehead protons (peaks 6 and 6′) due to their different chemical environments, where the peaks at 2.28 and 2.07 ppm were assigned to the syn isomer and the peak at 2.18 ppm corresponded to the anti-isomer. The anti-to-syn ratio was approximately 1
:
1 according to the peak integrals. Three peaks (peaks 9 and 9′) were also observed for the bridgehead carbons in the 13C NMR spectrum of CANAL-4, further confirming the existence of regioisomers (Fig. S1†). Similar results were observed in the NMR spectra of CANAL-2 (Fig. 1B and S2†). The signals in the 1H and 13C NMR spectra of CANAL-2 and CANAL-4 were fully assigned (Fig. 1 and S1, S2†), and the ratios of integrals of all the peaks in 1H spectra generally agreed with the calculated values. CANAL-2 was used for CPI synthesis without separation of the regioisomers. The pure regioisomers of CANAL-4, i.e. anti-CANAL-4 and syn-CANAL-4, were isolated according to a literature method.38 The singlet at 2.19 ppm in the 1H NMR spectrum of anti-CANAL-4 was indicative of the same chemical environment of the bridgehead protons (peak 6 in Fig. S3†). In contrast, two singlets were observed at 2.34 and 2.26 ppm in the 1H NMR spectrum of syn-CANAL-4 (peaks 6 and 6′ in Fig. S4†), reflecting the distinctive chemical environments of the bridgehead protons. The aforementioned results were in good accordance with the 1H NMR patterns in our previous report.38
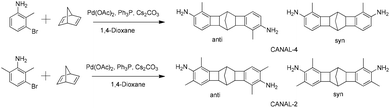 |
| Scheme 1 Synthetic routes to CANAL diamines. | |
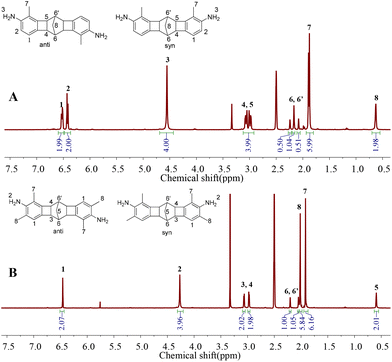 |
| Fig. 1
1H NMR spectra of CANAL-4 (A) and CANAL-2 (B). | |
Polymer syntheses
As depicted in Scheme 2, the high temperature solution polycondensation of the regioisomer mixture of N2BC-containing diamines with typical dianhydrides, i.e., 6FDA, HPMDA, HBPDA, BTA, and CpODA, produced a series of CPIs. For comparative purposes, CPIs were also prepared from pure regioisomers of CANAL-4 and representative dianhydrides (6FDA and CpODA). The number-average molecular weights (Mn) and polydispersity (PDI) of the CPIs from the regioisomer mixture were 22–202 kg mol−1 and 1.48–2.91, respectively (Table S1†). The molecular weights of the CPIs from pure regioisomers and 6FDA were markedly higher than that of 6FDA-CANAL-4, possibly due to the improvement in monomer purity during the isolation. Robust and transparent films were readily cast from m-cresol or NMP solutions, further confirming their adequate molecular weights. The successful synthesis of CPIs with designated structures was verified by their FT-IR and 1H NMR spectra. In the 1H NMR spectra of 6FDA-derived CPIs, the signals at around 7.9–8.1 and 6.9–7.1 ppm were assignable to the aromatic protons. The signals at 3.3–3.4, 2.4–2.6, 2.0–2.1, and 1.0 ppm represented the cyclobutene methine protons, bridgehead methine protons, benzylmethyl protons, and methylene protons, respectively (Fig. S5 and S6†). No signals were detected in the downfield region (>10 ppm), indicating the completion of imidization reactions. In the FTIR spectra of the CPIs (Fig. S7†), the characteristic absorption bands at around 1780, 1720 and 1370 cm−1 were ascribed to the asymmetric and symmetric C
O stretching, and C–N stretching in imide groups, respectively. Moreover, the peaks at 2850 and 2920 cm−1 corresponded to the secondary and tertiary C–H stretching. No absorptions were detected at around 3080 (N–H stretching) and 1650 cm−1 (C
O stretching in amide groups), further confirming complete ring closure in these CPIs.
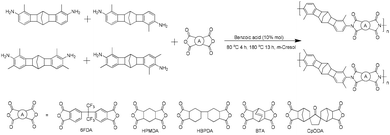 |
| Scheme 2 Synthetic routes to N2BC-containing CPIs. | |
Polymer properties
Microstructures.
The microstructures of the N2BC-containing CPIs were characterized through WAXD measurements. All the CPIs were amorphous as indicated by their broad diffraction features (Fig. 2). The average interchain distances (d-spacing) of the CANAL-4 and CANAL-2-derived CPIs were 5.72–6.03 and 5.93–6.30 Å, respectively (Table 1). For certain dianhydrides, the d-spacing values of the CANAL-2-derived CPIs were markedly higher than those of the CANAL-4-based ones. Chain packing in the CANAL-2-derived CPIs was hindered to a greater extent in comparison with that in the CANAL-4-based ones. This can be rationalized in terms of the fact that the two ortho methyl groups in each imide unit can more efficiently prevent the rotation of the C–N bonds compared to the mono-ortho-methylated imide segments. Furthermore, the CPIs from anti-CANAL-4 showed slightly lower d-spacing values than those from syn-CANAL-4. This phenomenon can be explained by the more uniform distribution of methyl substituents in the anti-CANAL-4-derived polymers (Fig. S8†).38 For the given diamines, the d-spacing values of these CPIs followed the order of HPMDA ≈ BTA > 6FDA ≈ CpODA > HBPDA. The HPBDA-derived CPIs displayed the most densely packed chains due to their most flexible chains. In contrast, the CPIs from HPMDA and BTA exhibited the greatest d-spacing values because of their most rigid backbones. Despite lower chain rigidity caused by the bridging linkage, the segmental motions in 6FDA and CpODA derived CPIs were suppressed by the bulky hexafluoroisopropylidene moieties or spiro structures, resulting in their moderate d-spacing values.23,40
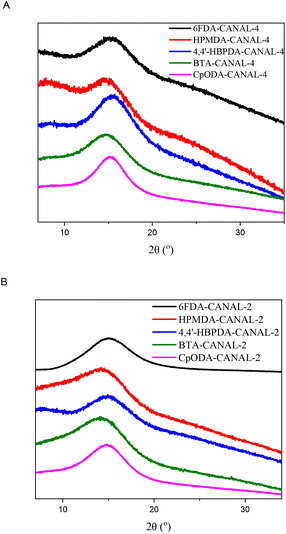 |
| Fig. 2 WAXD patterns of N2BC-containing CPIs (A: CANAL-4-derived CPIs and B: CANAL-2-derived CPIs). | |
Table 1 Microstructural, thermal, and mechanical properties of N2BC-containing CPIs
Polyimide |
d-Spacing (Å) |
T
g a (°C) |
T
5% b (°C) |
Char yieldb (%) |
Tensile strength (MPa) |
Modulus (GPa) |
Elongation at break (%) |
CTEc (ppm K−1) |
Glass transition temperature, determined by DMA at a heating rate of 3 °C min−1 at 1 Hz.
Measured by TGA in nitrogen at a heating rate of 10 °C min−1.
Coefficient of thermal expansion, measured using TMA at a heating rate of 5 K min−1 from 100 to 200 °C.
The structures of the comparative polyimides are depicted in Scheme S1† (yellow polyimides: PMDA-ODA and BPDA-PDA; aromatic CPIs: 6FDA-TFMB, BPDA-TFMB, and 6FDA-6FBAPP; and semi-aromatic CPIs: CBDA-TFMB and H'PMDA-TFMB).
|
6FDA-CANAL-4 |
5.79 |
448 |
475 |
54.1 |
90 |
1.9 |
7.6 |
53.1 |
6FDA-anti-CANAL-4 |
5.71 |
440 |
489 |
58.6 |
119 |
2.4 |
8.2 |
43.9 |
6FDA-syn-CANAL-4 |
5.86 |
441 |
484 |
59.8 |
101 |
2.1 |
7.3 |
49.0 |
HPMDA-CANAL-4 |
5.97 |
466 |
483 |
41.7 |
90 |
2.1 |
9.7 |
46.9 |
HBPDA-CANAL-4 |
5.72 |
418 |
454 |
20.9 |
100 |
2.3 |
7.6 |
56.5 |
BTA-CANAL-4 |
6.03 |
452 |
451 |
31.9 |
92 |
1.9 |
12.8 |
42.0 |
CpODA-CANAL-4 |
5.83 |
462 |
466 |
30.3 |
87 |
2.1 |
15.3 |
34.3 |
CpODA-anti-CANAL-4 |
5.80 |
459 |
464 |
28.4 |
102 |
2.6 |
7.3 |
23.9 |
CpODA-syn-CANAL-4 |
5.82 |
462 |
462 |
29.0 |
87 |
1.9 |
18.3 |
35.2 |
6FDA-CANAL-2 |
6.01 |
456 |
484 |
55.5 |
70 |
1.6 |
10.5 |
57.4 |
HPMDA-CANAL-2 |
6.30 |
480 |
488 |
43.3 |
78 |
1.6 |
12.1 |
51.2 |
HBPDA-CANAL-2 |
5.93 |
437 |
450 |
20.9 |
76 |
1.5 |
10.0 |
66.2 |
BTA-CANAL-2 |
6.27 |
460 |
455 |
31.4 |
81 |
1.8 |
11.1 |
45.9 |
CpODA-CANAL-2 |
5.98 |
478 |
480 |
29.4 |
78 |
1.8 |
13.5 |
44.8 |
PMDA-ODA46,d |
|
421 |
560 |
|
121 |
1.5 |
52 |
31 |
BPDA-PDA44 |
|
350 |
606 |
|
424 |
5.7 |
52 |
4 |
6FDA-TFMB47 |
|
351 |
542 |
|
99 |
3.1 |
5.8 |
59 |
BPDA-TFMB44 |
|
373 |
580 |
|
286 |
4.1 |
31 |
20 |
6FDA-6FBAPP8 |
|
263 |
|
|
87 |
2.0 |
16 |
51 |
CBDA-TFMB48 |
|
356 |
459 |
|
103 |
3.65 |
5 |
20.7 |
H'PMDA-TFMB48 |
|
357 |
491 |
|
110 |
2.73 |
57 |
46.0 |
Solubility.
The solubility of the N2BC-containing CPIs was measured in various organic solvents (Table S1†). These CPIs displayed better solubility in typical organic solvents compared to conventional aromatic or semi-aromatic CPIs reported in the lieterature.41–43 In particular, some CPIs were even soluble in low boiling solvents including tetrahydrofuran and chloroform. This observation can be ascribed to the synergistic effects of unique W-shaped N2BC moieties and ortho-methyl substituents, which can prevent dense chain packing and suppress the inter-molecular CTC formation. The CTC formation was further weakened due to the poor electron-withdrawing capability of the alicyclic dianhydride residues, leading to the significantly enhanced solubility of the N2BC-containing CPIs. The 6FDA-derived CPIs showed the best solubility in this series, which can be attributed to the further weakened inter- and intra-molecular interactions originating from the lower polarizability of C–F bonds, as well as the bulky hexafluoroisopropylidene moieties.40 In contrast, the CpODA-derived polymers exhibited the poorest solubility in polar solvents due to their highest contents of non-polar alicyclic segments, as well as the additional dipole–dipole interactions caused by the ketone groups.23 For the given dianhydrides, the CPIs derived from CANAL-2, CANAL-4, and pure regioisomers of CANAL-4 exhibited similar solubility in spite of the difference in chain packing, indicating that their solubility behavior was predominantly influenced by the N2BC moieties.
Thermal properties
The thermal decomposition behavior of the N2BC-containing CPIs was evaluated through TGA measurements. One-stage degradation profiles were observed for all the CPIs (Fig. S9 and S10†). The 5% weight loss temperatures (T5%) of the CPIs were 450–489 °C (Table 1), inferior to those of archetypal wholly aromatic polyimides (542–606 °C).8,41–44,46,47 For the given diamines, the CPIs from 6FDA and HPMDA exhibited greater T5% values relative to other CPIs, while those from HBPDA and BTA displayed lower thermal stability. This observation implied that the thermal stability of these CPIs was dictated by their imide and aromatic contents, which tended to be less susceptible to thermal degradation than aliphatic segments. A similar trend was also observed for char yields at 800 °C, which followed the sequence of 6FDA > HPMDA > BTA ≈ CpODA > HBPDA for certain diamines. For certain dianhydrides, the CPIs from CANAL-2, CANAL-4, and pure regioisomers of CANAL-4 showed similar T5% and char yield values because of their structural similarity.
The glass transition profiles of the N2BC-containing CPIs were studied by using DMA (Table 1 and Fig. 3). Several aspects can influence the Tg values of polyimides, including structural rigidity, and inter- and intra-molecular interactions. Bulky segments, such as fused rings and methyl groups adjacent to the imide rings, can restrict intra-segmental rotations and thus significantly enhance the chain rigidity. On the other hand, flexible linkages can improve the inter- and intra-molecular interactions by facilitating dense chain packing, reflected by the interchain distances. In this work, the synergistic effects of bulky, rigid, contorted N2BC moieties and ortho-positioned methyl groups resulted in exceptionally high Tg (418–480 °C) of the resultant CPIs, significantly higher than those of the majority of wholly and semi-aromatic polyimides in the literature.7,8,42–48 Thermal degradation may occur in DMA measurements since the Tg values of some polymers were comparable or even higher than their corresponding T5% values. The Tg values of the CANAL-2-derived CPIs were 8–19 °C higher than those of the CANAL-4-derived polymers for certain dianhydrides, despite their apparently weaker inter- and intra-molecular interactions revealed by their greater d-spacing values. This phenomenon suggested that intra-chain rigidity dominated the glass transition behavior. Specifically, the rotational motion of the C–N bonds in the imide rings of the CANAL-2-derived CPIs was restricted to a greater extent by two ortho-methyl substituents relative to that of the mono-methylated CANAL-4-derived ones. For certain dianhydrides, the CPIs from anti-CANAL-4 exhibited slightly lower Tg values relative to those from syn-CANAL-4 (Table 1 and Fig. 3C). For the given diamines, the Tg of these CPIs followed the order of HPMDA > CpODA > BTA > 6FDA > HBPDA. The CPIs from HBPDA displayed the lowest Tg across this series because of their lowest aromatic contents and most flexible backbones. Despite the presence of bridge linkages, the 6FDA-derived compound showed considerably higher Tg than the HBPDA-based ones due to the restricted chain motion caused by the bulky hexafluoroisopropylidene moieties, as well as their highest aromatic contents. The Tg values of the CPIs from HPMDA, BTA, and CpODA were significantly higher than those of the 6FDA- and HBPDA-based ones, which can be ascribed to the lack of flexible linkages in the dianhydride residues. Despite their highest alicyclic contents, the CpODA-derived CPIs exhibited apparently higher Tg than those from BTA. This can be explained by their stronger inter- and intra-molecular interactions, particularly dipole–dipole interactions of the ketone groups, evidenced by their lower d-spacing values. The highest Tg values of the HPMDA-derived CPIs can be rationalized in terms of their highest imide contents, as well as balanced chain rigidity and interchain distances.
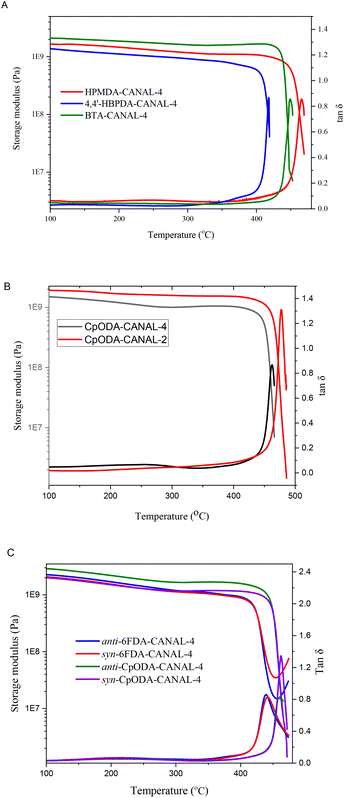 |
| Fig. 3 DMA curves of N2BC-containing CPIs (A: CANAL-4-derived CPIs; B: CANAL-2-derived CPIs; and C: CPIs from pure regioisomers). | |
Dimensional stability is essential for the practical use of CPIs, which can be evaluated according to their in-plane CTE values determined by TMA measurements (Fig. 4). As listed in Table 1, the CTE values of the N2BC-containing CPIs were 23.9–66.2 ppm K−1. In particular, CpODA-anti-CANAL-4 displayed a CTE of 23.9 ppm K−1 given its high Tg (459 °C) and light coloration. For the given diamines, the CTE values of the CPIs were in the sequence of HBPDA > 6FDA > HPMDA > BTA > CpODA, varying with their chain rigidity, linearity, and average interchain distances. The flexible cyclohexyl units in the HBPDA-derived CPIs and the bent hexafluoroisopropylidene segments in 6FDA-derived CPIs accounted for their higher CTE due to the decrease in chain linearity and rigidity.21,44,45 In contrast, the CPIs derived from HPMDA, BTA, and CpODA displayed better dimensional stability due to the absence of flexible segments. Particularly, the combined effects of trans-conformation, additional dipole–dipole interactions caused by the ketone group, and restricted chain motions originating from the spiro-center led to the lowest CTE values of the CpODA-derived CPIs for certain diamines. Moreover, the cis-conformation of BTA and HPMDA gave rise to relatively higher CTE of the resulting CPIs compared to the CpODA-derived ones because of the reduced chain rectilinearity.23 For certain dianhydrides, the CANAL-2-derived CPIs displayed inferior dimensional stability compared to the CANAL-4-derived ones, likely due to the enlarged interchain distances caused by the two ortho-methyl substituents. Similarly, the more compact chain packing in the anti-CANAL-4-derived CPIs accounted for their apparently lower CTE values compared to their counterparts from syn-CANAL-4 (Fig. 4C).
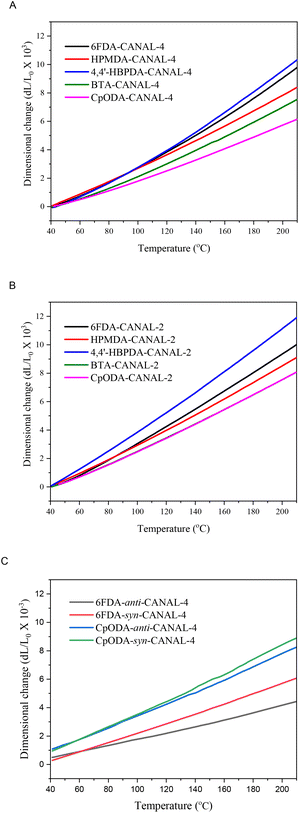 |
| Fig. 4 TMA curves of the N2BC-containing CPIs (A: CANAL-4-derived CPIs; B: CANAL-2-derived CPIs; and C: CPIs from pure regioisomers). | |
Mechanical properties
The tensile strength, modulus, and elongation-at-break of the N2BC-containing CPIs were in the range of 70–119 MPa, 1.5–2.6 GPa, and 7.6–18.3%, respectively. These properties were significantly inferior to those of wholly and semi-aromatic polyimides due to their enlarged interchain distances originating from alicyclic segments and W-shaped contorted N2BC moieties (Table 1).8,41–44,46–48 In general, the CANAL-4-derived CPIs possessed better mechanical properties than the CANAL-2-derived polymers, which can be attributed to their more pronounced inter- and intra-molecular interactions evidenced by lower d-spacing values. For instance, the tensile strength values of CANAL-4-derived CPIs were 11–32% higher than those of CANAL-2-derived ones. Meanwhile, the moduli of CANAL-4-derived CPIs were also 10–50% greater than those of CPIs based on CANAL-2. However, CANAL-4-derived CPIs displayed slightly inferior ductility to CANAL-2-derived ones. Furthermore, the CPIs from pure regioisomers tended to show better mechanical properties than those from the mixture of regioisomers, which can be attributed to their obviously higher molecular weights.
Optical properties
The optical properties of the N2BC-containing CPIs were assessed by using UV-vis spectroscopy (Fig. 5). As listed in Table 2, the values of λcut–off and transmittance at 400 nm (T400) for these CPIs were 281–343 nm and 61–84%, respectively, indicating their excellent optical properties. The 6FDA-derived CPIs exhibited obviously greater λcut–off and lower T400 values compared to those from alicyclic dianhydrides. This was possibly due to their more pronounced CTC formation originating from the stronger electron-withdrawing ability of aromatic dianhydrides. In contrast, the poor electron-accepting properties of the alicyclic dianhydride residues led to complete inhibition of CTC formation in the resulting polyimides. Consequently, the CPIs from alicyclic dianhydrides demonstrated better optical transparency in comparison with the 6FDA-derived polymers.9–11 For the given dianhydrides, the CANAL-4-derived CPIs displayed similar optical properties to the CANAL-2-derived ones.
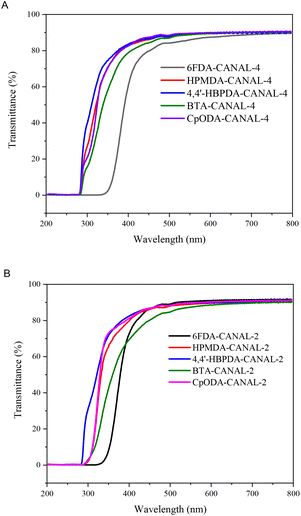 |
| Fig. 5 UV-vis spectra of N2BC-containing CPIs (thickness: 20–30 μm) (A: CANAL-4-derived CPIs and B: CANAL-2-derived CPIs). | |
Table 2 Optical properties of N2BC-containing CPIsa
Polyimide |
T
400 b (%) |
λ
cut-off (nm) |
Lightness (L*) |
Yellowness (b*) |
Redness (a*) |
YI E313 [D65/10]c |
The color parameters were calculated according to a CIE LAB equation, with a film thickness of ∼20–30 μm. L* refers to lightness; 100 means white, while 0 indicates black. Positive a* means red color, and negative a* indicates green color. Positive b* means yellow color, and negative b* indicates blue color.
Transmittance at 400 nm.
Yellowness index.
|
6FDA-CANAL-4 |
61 |
343 |
92.8 |
27.4 |
−8.0 |
40.4 |
HPMDA-CANAL-4 |
83 |
283 |
95.6 |
1.8 |
−0.16 |
3.2 |
HBPDA-CANAL-4 |
84 |
281 |
95.6 |
2.5 |
−0.22 |
3.3 |
BTA-CANAL-4 |
79 |
284 |
95.5 |
2.6 |
−0.24 |
4.7 |
CpODA-CANAL-4 |
84 |
282 |
95.8 |
1.4 |
−0.18 |
2.6 |
6FDA-CANAL-2 |
72 |
332 |
96.2 |
2.7 |
−0.53 |
4.7 |
HPMDA-CANAL-2 |
80 |
294 |
95.8 |
2.6 |
−0.39 |
4.6 |
HBPDA-CANAL-2 |
83 |
284 |
95.0 |
3.6 |
−0.48 |
6.4 |
BTA-CANAL-2 |
73 |
290 |
95.3 |
4.1 |
−0.51 |
7.3 |
CpODA-CANAL-2 |
81 |
292 |
95.7 |
1.8 |
−0.08 |
3.4 |
The lightness (L*), yellowness (b*), redness (a*) and yellowness index (YI) of the N2BC-containing CPIs were measured to evaluate their color intensities. The L*, b*, a*, and YI values of these polymers were 92.8–96.2, 1.4–27.4, −8.0–0.08, and 2.6–40.4, respectively (Table 2 and Fig. S11†). The incorporation of the rigid and contorted W-shaped N2BC segments and the alicyclic moieties interrupted the electronic conjugation, increased interchain distances, suppressed CTC formation, and consequently led to their reduced coloration intensities (Table 2). As expected, the 6FDA-derived CPIs exhibited the deepest coloration due to their highest aromatic contents and more pronounced CT interactions. Particularly, 6FDA-CANAL-4 was pale yellow, with its b* and YI values being 27.4 and 40.4, respectively. The rest of the CPIs from alicyclic dianhydrides showed much lighter color densities, and the differences in their b* and YI values were negligible.
Conclusions
Two N2BC-containing diamines, i.e. CANAL-2 and CANAL-4, were synthesized by CANAL reactions using bromo-anilines and norbornadiene as the starting materials. A mixture of regioisomers was obtained for each diamine as revealed by NMR spectra, and the regioisomers of CANAL-4 were successfully isolated by column chromatography. Two sets of CPIs were then prepared from these CANAL diamines and commercial dianhydrides. The rigid, contorted and W-shaped N2BC moieties imparted exceptionally high Tg, reduced coloration, and favorable thermal and mechanical properties to the resulting CPIs. Notably, these CPIs exhibited Tg values of 418–480 °C, which were even higher than those of yellowish, aromatic polyimides. Furthermore, except for the 6FDA-derived polymers, all the other CPIs displayed a λcut–off <300 nm, T400 >73%, and yellow index <7.3, indicating their excellent optical transparency. Moreover, these CPIs also demonstrated good dimensional stability, reflected by their CTE as low as 23.9 ppm K−1. For certain dianhydrides, the CANAL-4-derived CPIs exhibited lower d-spacing values, lower Tg, and reduced CTE but improved mechanical properties relative to the CANAL-2-derived ones. This can be attributed to their more pronounced inter- and intra-molecular interactions but lower resistance to segmental motions caused by the mono-methylated imide units compared to the di-methylated ones. Moreover, the CPIs from anti-CANAL-4 exhibited lower d-spacing and CTE values than those from syn-CANAL-4, possibly due to their more uniform distribution of methyl substituents. For the given diamines, the CPIs from CpODA displayed the best comprehensive properties due to the rigid and sterically hindered CpODA residues, as well as the additional dipole–dipole interactions caused by the ketone groups. The N2BC-containing CPIs developed in this work show great potential for optical applications due to their outstanding integrated properties, such as high optical transparency, exceptionally high Tg, good dimensional stability, and reasonable mechanical properties.
Conflicts of interest
There are no conflicts to declare.
Acknowledgements
The authors are thankful to the Key Research & Development Program of Zhejiang Province (No. 2021C01186 and 2021C01126) and Key Technical Development Program of Ningbo City (2021Z091) for the financial support.
References
- P. K. Tapaswi and C. S. Ha, Macromol. Chem. Phys., 2019, 220, 1800313 CrossRef.
- M. Hasegawa, Polymers, 2017, 9, 520 CrossRef PubMed.
- S. Nakano, N. Saito, K. Miura, T. Sakano, T. Ueda, K. Sugi, H. Yamaguchi, I. Amemiya, M. Hiramatsu and A. Ishida, J. Soc. Inf. Disp., 2012, 20, 493–498 CrossRef CAS.
- H. Yamaguchi, T. Ueda, K. Miura, N. Saito, S. Nakano, T. Sakano, K. Sugi, I. Amemiya, M. Hiramatsu and A. Ishida, SID Int. Symp. Dig. Tech. Pap., 2012, 43, 1002–1005 CrossRef.
- M. C. Choi, Y. Kim and C. S. Ha, Prog. Polym. Sci., 2008, 33, 581–630 CrossRef CAS.
- J. M. Liu, T. M. Lee, C. H. Wen and C. M. Leu, J. Soc. Inf. Disp., 2011, 19, 63–69 CrossRef CAS.
- Y. Zhuang, J. G. Seong and Y. M. Lee, Prog. Polym. Sci., 2019, 92, 35–88 CrossRef CAS.
- H. J. Ni, J. G. Liu, Z. H. Wang and S. Y. Yang, J. Ind. Eng. Chem., 2015, 28, 16–27 CrossRef CAS.
- M. Hasegawa and K. Horie, Prog. Polym. Sci., 2001, 26, 259–335 CrossRef CAS.
-
S. Ando, Optical Properties of Polyimides, in The Latest Polyimides: Fundamentals and Applications, ed. S. Ando, M. Ueda, M. Kakimoto, M. Kochi, T. Takeichi, M. Hasegawa and R. Yokota, NTS, Tokyo, Japan, 2nd edn, 2010, pp. 102–128 Search PubMed.
-
T. Matsumoto, Alicyclic polyimides, in The Latest Polyimides: Fundamentals and Applications, ed. S. Ando, M. Ueda, M. Kakimoto, M. Kochi, T. Takeichi, M. Hasegawa and R. Yokota, NTS, Tokyo, Japan, 2nd edn, 2010, pp. 231–246 Search PubMed.
- T. Matsumoto, D. Mikami, T. Hashimoto, M. Kaise, R. Takahashi and S. Kawabata, J. Phys.: Conf. Ser., 2009, 187, 012005 CrossRef.
- H. Suzuki, T. Abe, K. Takaishi, M. Narita and F. Hamada, J. Polym. Sci., Part A: Polym. Chem., 2000, 38, 108–116 CrossRef CAS.
- M. Hasegawa, M. Horiuchi and Y. Wada, High Perform. Polym., 2007, 19, 175–193 CrossRef CAS.
- M. Hasegawa, High Perform. Polym., 2001, 13, S93–S106 CrossRef CAS.
- M. Hasegawa and M. Koyanaka, High Perform. Polym., 2003, 15, 47–64 CrossRef CAS.
- L. Zhai, S. Yang and L. Fan, Polymer, 2012, 53, 3529–3539 CrossRef CAS.
- M. Hasegawa, D. Hirano, M. Fujii, M. Haga, E. Takezawa, S. Yamaguchi, A. Ishikawa and T. Kagayama, J. Polym. Sci., Part A: Polym. Chem., 2013, 51, 575–592 CrossRef CAS.
- M. Hasegawa, M. Fujii, J. Ishii, S. Yamaguchi, E. Takezawa, T. Kagayama and A. Ishikawa, Polymer, 2014, 55, 4693–4708 CrossRef CAS.
- R. Lian, X. Lei, Y. Xiao, S. Xue, G. Xiong, Z. Zhang, D. Yan and Q. Zhang, Polym. Chem., 2021, 12, 4803–4811 RSC.
- X. Hu, H. Mu, Y. Wang, Z. Wang and J. Yan, Polymer, 2018, 134, 8–19 CrossRef CAS.
- A. Shiotani, H. Shimazaki and M. Matsuo, Macromol. Mater. Eng., 2001, 286, 434–441 CrossRef CAS.
- H. Ozawa, E. Ishiguro, Y. Kyoya, Y. Kikuchi and T. Matsumoto, polymers, 2021, 13, 2824 CrossRef CAS PubMed.
- M. Kusama, T. Matsumoto and T. Kurosaki, Macromolecules, 1994, 27, 1117–1123 CrossRef CAS.
- T. Matsumoto, Macromolecules, 1999, 32, 4933–4939 CrossRef CAS.
- R. Kimura and T. Matsumoto, Kobunshi Ronbunshu, 2011, 68, 127–131 CrossRef CAS.
- T. Matsumoto, E. Ishiguro and S. Komatsu, J. Photopolym. Sci. Technol., 2014, 27, 167–171 CrossRef.
- X. Hu, J. Yan, Y. Wang, H. Mu, Z. Wang, H. Cheng, F. Zhao and Z. Wang, Polym. Chem., 2017, 8, 6165–6172 RSC.
- J. Miao, X. Hu, X. Wang, X. Meng, Z. Wang and J. Yan, Polym. Chem., 2020, 11, 6009–6016 RSC.
- Y. Zhuang, R. Orita, E. Fujiwara, Y. Zhang and S. Ando, Macromolecules, 2019, 52, 3813–3824 CrossRef CAS.
- S. Liu, Z. Jin, Y. C. Teo and Y. Xia, J. Am. Chem. Soc., 2014, 136, 17434–17437 CrossRef CAS.
- H. W. H. Lai, S. Liu and Y. Xia, J. Polym. Sci., Part A: Polym. Chem., 2017, 55, 3075–3081 CrossRef CAS.
- H. W. H. Lai, Y. C. Teo and Y. Xia, ACS Macro Lett., 2017, 6, 1357–1361 CrossRef CAS.
- H. W. H. Lai, F. M. Benedetti, Z. Jin, Y. C. Teo, A. X. Wu, M. G. De Angelis, Z. P. Smith and Y. Xia, Macromolecules, 2019, 52, 6294–6302 CrossRef CAS.
- H. W. H. Lai, F. M. Benedetti, J. M. Ahn, A. M. Robinson, Y. Wang, I. Pinnau, Z. P. Smith and Y. Xia, Science, 2022, 375, 1390–1392 CrossRef CAS.
- D. A. Alentiev and M. V. Bermeshev, Polym. Rev., 2021, 1–38 Search PubMed.
- X. Ma, H. W. H. Lai, Y. Wang, A. Alhazmi, Y. Xia and I. Pinnau, ACS Macro Lett., 2020, 9, 680–685 CrossRef CAS PubMed.
- X. Hu, J. Miao, Y. Pang, J. Zhao, Y. Lu, H. Guo, Z. Wang and J. Yan, Polym. Chem., 2022, 13, 2842–2849 RSC.
- M. A. Abdulhamid, H. W. H. Lai, Y. Wang, Z. Jin, Y. C. Teo, X. Ma, I. Pinnau and Y. Xia, Chem. Mater., 2019, 31, 1767–1774 CrossRef CAS.
- D. F. Sanders, Z. P. Smith, R. Guo, L. M. Robeson, J. E. McGrath, D. R. Paul and B. D. Freeman, Polymer, 2013, 54, 4729–4761 CrossRef CAS.
- J. Lin and D. C. Sherrington, Adv. Polym. Sci., 1994, 111, 177–219 CrossRef CAS.
- C. E. Sroog, Prog. Polym. Sci., 1991, 16, 561–694 CrossRef CAS.
- M. Ding, Prog. Polym. Sci., 2007, 32, 623–668 CrossRef CAS.
- A. E. Feiring, B. C. Auman and E. R. Wonchoba, Macromolecules, 1993, 26, 2779–2784 CrossRef CAS.
- T. Matsuura, N. Yamada, S. Nishi and Y. Hasuda, Macromolecules, 1993, 26, 419–423 CrossRef CAS.
- Y. Zhou, S. Zhang, F. Zheng and Q. Lu, Macromolecules, 2021, 54, 9307–9318 CrossRef CAS.
- J. He, H. Yang, F. Zheng and S. Yang, Polymers, 2022, 14, 649 CrossRef CAS.
- M. Hasegawa, Y. Watanabe, S. Tsukuda and J. Ishii, Polym. Int., 2016, 65, 1063–1073 CrossRef CAS.
Footnote |
† Electronic supplementary information (ESI) available: Experimental section, 13C NMR spectra of monomers, 1H NMR and FT-IR spectra, TGA curves, images, molecular weights, and solubility for the polymers. See DOI: https://doi.org/10.1039/d2py00833e |
|
This journal is © The Royal Society of Chemistry 2023 |