Effects of phosphine ligands in nickel-catalyzed decarbonylation reactions of lactone†
Received
2nd August 2023
, Accepted 29th August 2023
First published on 29th August 2023
Abstract
Due to the ubiquity of carbonyl compounds and the abundance of nickel on the earth, nickel-catalyzed decarbonylation has garnered increasing attention in recent years. This type of reaction has seen significant developments in various aspects; however, certain challenges concerning reactivity, selectivity, and transformation efficiency remain pressing and demand urgent resolution. In this study, we employed DFT calculations to investigate the mechanism of nickel-catalyzed decarbonylation reactions involving lactones, as well as the effects of phosphine ligands. Mechanically, Ni(0) first activates the C(acyl)–O bond of the lactone, followed by a decarbonylation step, and ultimately results in reductive elimination under carbonyl coordination to yield the product. Through a comprehensive examination of the electronic and steric effects of the phosphine ligands, we deduced that the electronic effect of the ligand plays a dominant role in the decarbonylation reaction. By enhancing the electron-withdrawing ability of the ligand, the energy barrier of the entire reaction can be significantly reduced. The obtained insights should be valuable for understanding the detailed mechanism and the role of phosphine ligands in nickel catalysis. Moreover, they offer crucial clues for the rational design of more efficient catalytic reactions.
Introduction
The carbonyl group is one of the most ubiquitous functional groups in organic compounds (e.g., acyl chlorides, anhydrides, esters, amides, aldehydes, and ketones), and it can be seen simultaneously in many natural and synthetic compounds. Among the transformation reactions of the carbonyl compounds, decarbonylation reactions have significant implications in organic synthesis.1–3 They can be used to selectively remove carbonyl groups from complex organic molecules without affecting other functional groups, allowing for the synthesis of hydrocarbon compounds that would be difficult to access through other methods.
Transition-metal-catalyzed decarbonylation has become a hot topic since 2010, because it provides a novel and creative synthetic strategy using carbonyl groups as “traceless handles”.4–7 For several years, chemists have been researching the utilization of various transition-metal catalysts, such as ruthenium,8–14 palladium,15–19 rhodium,20–23 and nickel,24–28 to facilitate decarbonylation reactions. These approaches have demonstrated significant success in the field of chemistry. Notably, nickel-based catalysts serve as prime examples of this success due to the abundance and affordability of nickel, which aligns with the principles of contemporary green chemistry.29,30 As shown in Scheme 1a, Ni-catalyzed decarbonylation reaction typically involves the following steps: (a) the oxidative addition of Ni to C–R1 or C–R2 bond leads to the formation of intermediate I or II; (b) the following decarbonylation of either intermediate I or II forms intermediate III; (c) intermediate III can directly undergo reductive elimination to yield R1–R2 product. Alternatively, it engages in transmetalation or C–H activation with another substrate to form intermediate V, which then undergoes reductive elimination to afford R1–R3 product.31,32
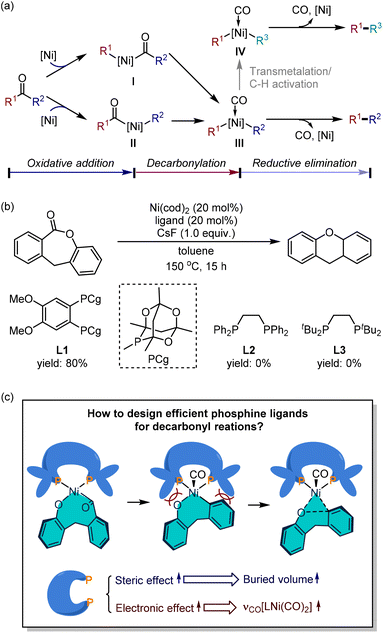 |
| Scheme 1 General mechanism of Ni-catalyzed decarbonylation reactions and the influence of phosphine ligands. | |
Although significant progress has been achieved in Ni-catalyzed decarbonylation reactions over the past few decades, some pending issues, such as reactivity, selectivity, and transformation efficiency, still need to be resolved urgently. It can be found that the choice of phosphine ligand has a great influence on the reaction.33–37 For example, Rueping et al. reported that the first example of ligand-controlled nickel catalyzed selective cross-coupling reaction of aromatic ester and alkyl organoboron.33 When monodentate phosphorus ligands (i.e., PnBu3 and PCy3) were used, the nickel complex favors activation of the C(acyl)–O bond to generate the ketone product. However, the nickel complex with bidentate dcype ligand favors the C(aryl)–C bond cleavage in the oxidation addition step, leading to the alkylated product via a decarbonylative process. In addition, Itami and Houk reported that phosphine ligands could result in the switchable chemoselectivity of Ni-catalyzed C(aryl)–O and C(acyl)–O activation of aryl esters.35,38 Therefore, a comprehensive theoretical investigation into the roles of phosphine ligands in Ni-catalyzed decarbonylation reactions is highly desirable.
For the present study, we have chosen the Ni-catalyzed decarbonylation of lactones depicted in Scheme 1b as our theoretical research model, which has been recently reported by the Li research group.39 It is worth mentioning that the lactone substrate is obtained by the Baeyer–Villiger reaction, and the decarbonylation reaction provides an effective strategy for the conversion of the Baeyer–Villiger reaction product. As depicted in Scheme 1b, experimental results demonstrate that employment of ligand L1 allows for an 80% yield in the reaction, while using ligands L2 and L3 yielded no desired product. Herein, the objective of this work is to identify the rate-determining step of the selected reaction and ascertain the specific role of phosphine ligands in the aforementioned three steps. Through a comprehensive analysis of the steric effect and electronic effect illustrated in Scheme 1c, this investigation aims to provide valuable insights that can help the rational design of phosphine ligands for these kinds of reactions.
Computational details
The Gaussian09 program was used to perform DFT calculations,40 and all geometry optimizations were completed at the M06-L41/def2-SVP42/SMDtoluene43 level. Harmonic vibrational frequency calculations verified each transition state has only one imaginary frequency and other stationary points have no imaginary frequencies. GoodVibes program (version 3.2) was used to introduce a correction for the change in standard state from gas phase at 1 atm to a 1 M solution at 423.15 K, and the Grimme's quasi-rigid-rotor-harmonic-oscillator (quasi-RRHO) correction was performed for all structures with a frequency cut-off of 100 cm−1.44–46 We further refined the energy by using single-point energy calculations at the M06-L/def2-TZVPP/SMDtoluene level for the optimized structures. Other DFT methods and basis sets were used to test the reliability of the selected computational level in part 2 of the ESI.† SambVca2.1 was used to calculate the buried volume and topographic steric maps of ligands.47,48 The 3D structures of the molecules were rendered by using the CYLview2.049 and VMD program.50 Atoms-in-molecules (AIM) analysis51 was performed with the Multiwfn program.52
Results and discussion
The reaction starts with the Ni(COD)2 catalyst undergoing ligand exchange, leading to the generation of the active intermediate 2(L1) and exothermically releasing 6.3 kcal mol−1 (Fig. 1). Following this, the oxidative addition of the lactone to Ni takes place, considering two potential activation modes of the lactone: one associated with the C(acyl)–O bond and the other with the C(aryl)–C bond. A comparison of the energy barriers of the transition states for these two oxidative addition pathways reveals that the activation of the C(acyl)–O bond via3-ts(L1) (ΔG‡ = 12.3 kcal mol−1) is more energetically favorable than the activation of the C(aryl)–O bond via7-ts(L1) (ΔG‡ = 21.4 kcal mol−1). Subsequently, intermediate 6(L1) can be obtained through the decarbonylation process from either intermediate 4(L1) or intermediate 8(L1). However, since the relative free energy of 5-ts(L1) is lower than that of 9-ts(L1), the transformation from intermediate 4(L1) to intermediate 6(L1) is deemed to be more energetically favorable. Then the reductive elimination process results in the formation of the 9H-xanthene product from intermediate 6(L1). During this process, we examined the impact of CO coordination. Specifically, intermediate 6(L1) can transform to intermediate 10(L1) with the CO coordination to Ni or dissociate CO to form intermediate 13, preparing for reductive elimination. The calculation results revealed that the energy barrier of transition state 11-ts(L1) is 4.0 kcal mol−1 lower than that of transition state 14-ts(L1), indicating that reductive elimination occurs more favorably in the presence of CO coordination. Finally, intermediate 12(L1) is transformed to reactive intermediate 2(L1) by dissociation of CO and coordinating with reactant 1(L1). The calculation results show that this process is endothermic, with an energy increase of 27.3 kcal. However, it is essential to acknowledge that this value may be an overestimation due to experimental conditions. Particularly, as the reaction takes place within a glove box, it is unlikely that the gaseous CO generated would reversibly regenerate intermediate 12(L1). Furthermore, we considered the possibility of direct oxidative addition of intermediate 12(L1) to the substrate (as shown in Fig. S1†), however, the corresponding energy barrier is as high as 41.3 kcal mol−1, indicating that intermediate 12(L1) needs to dissociate CO before proceeding to the next round of the catalytic cycle. A direct pathway from intermediate 4 to the product via the transition state 16-ts was also considered, but the energy barrier of this pathway is as high as 49.7 kcal mol−1, so it can be ruled out.
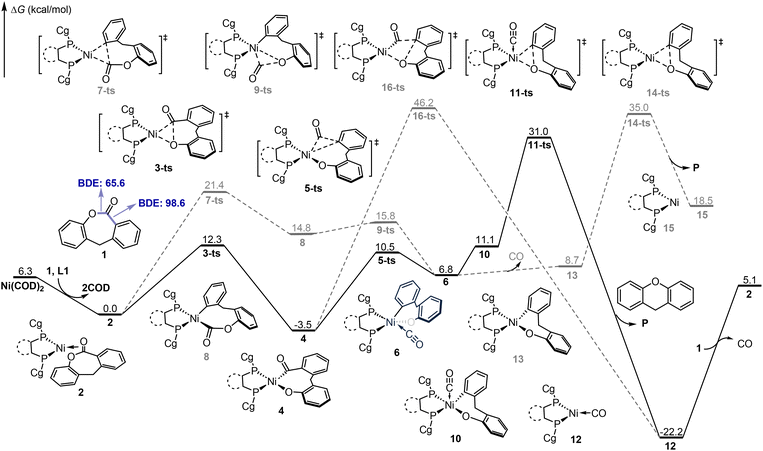 |
| Fig. 1 Free energy profiles for nickel-catalyzed decarbonylation of lactone using L1 as the phosphine ligand. | |
As mentioned above, the carbonyl group acts as an electron-withdrawing group, and its coordination can effectively reduce the energy barrier of reductive elimination. The calculated results align with experimental observations, wherein the additive Cr(CO)6 promotes the reductive elimination process of intermediate 13(L1).39 To gain further insights, AIM analysis was performed for intermediates 10(L1) and 13(L1) in Fig. 2. It was found that the electron density at the bond critical point of the Ni–C bond and Ni–O bond in 10(L1) is smaller than that of the corresponding bond in 13(L1). Consequently, the coordination of carbonyl weakens the strength of the Ni–C bond and Ni–O bond, leading to a reduction in the energy barrier of the reductive elimination transition state.
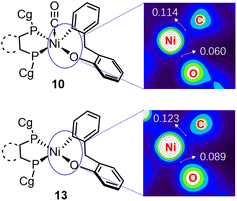 |
| Fig. 2 AIM analysis for intermediates 10(L1) and 13(L1). (Unit: a.u.) | |
The influence of ligands on the Ni-catalyzed decarbonylation reaction can be categorized into steric and electronic effects. Firstly, we focused on the electronic effect of ligands, particularly by selecting ligands with similar steric effects but different electronic properties. As shown in Fig. 3, 1,2-bis(diphenylphosphino)ethane (dppe) ligand (L2) was used as a template ligand, and the electronic effect of the ligand was altered by introducing different substituents (F group and CN group) on the phenyl group of the ligand. As reported in previous literature, buried volume was employed to evaluate the steric effect of ligands,53–56 while the vibration frequency of CO in LnNi(CO)n complexes served as an indicator of the electronic effect of ligands.57,58 Since the added substituents were far away from the substrate in the reaction center, the buried volumes of these different substituent ligands (L2, L4, and L5) are similar, indicating that the steric effects of these ligands are almost the same. In terms of electron effect, the vibration frequency of CO in L2, L4, and L5 are 2081.3 cm−1, 2093.6 cm−1, and 2096.3 cm−1, respectively. As a result, the electron-withdrawing ability of ligands can be ranked as L2 < L4 < L5.
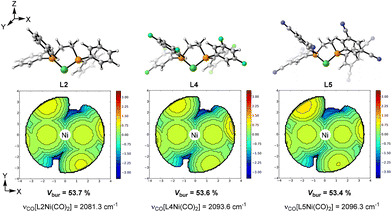 |
| Fig. 3 Comparison of steric and electron effects of ligands L2, L4, and L5. | |
The optimal pathway for the Ni-catalyzed decarbonylation reaction has been elucidated in Fig. 1. Based on this, we present the calculated results of various ligands in Fig. 4. As depicted in Fig. 4, the energy barriers for ligands with different electronic effects exhibit similarities during the oxidative addition step. Thermodynamically, a stronger electron-withdrawing ability corresponds to a higher relative free energy of intermediate 4. For instance, when employing the nitrile-substituted ligand L5, the corresponding intermediate 4(L5) exhibits an energy of 2.1 kcal mol−1 higher than that of intermediate 2(L5). In the subsequent decarbonylation step, the energy barriers required for different ligands are approximately 15.0 kcal mol−1, and the formation of the reductive elimination precursor 10 is consistently around 13.0 kcal mol−1 (relative to intermediate 4). These observations suggest that the electronic effect of the ligand exerts minimal influence on carbonyl insertion process and the formation of the reductive elimination precursor 10. However, during the reductive elimination step, the energy barrier differs among ligands. The dppe ligand demonstrates an energy barrier of 22.9 kcal mol−1, the F-substituted ligand (L4) exhibits 21.5 kcal mol−1, and the nitrile-substituted ligand (L5) significantly reduces the barrier to 16.6 kcal mol−1. These findings indicate that a stronger electron-withdrawing ability of the ligand facilitates the reductive elimination process, suggesting that different ligands could theoretically enhance the reaction rate.
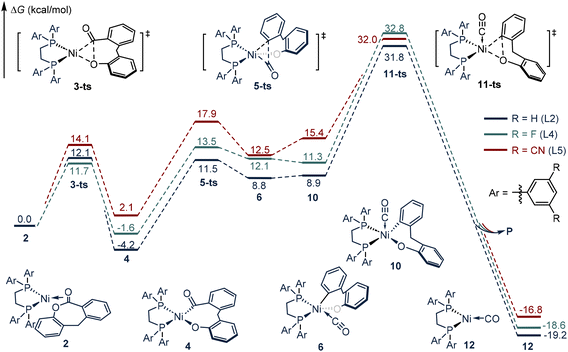 |
| Fig. 4 Comparison of L2, L4 and L5 in nickel-catalyzed decarbonylation of lactone. | |
Subsequently, we conducted a further study on the ligand steric effects by replacing the methyl group of trioxaphosphaadamantane (PCg) group in L1 with a hydrogen atom, resulting in L6. Fig. 5 illustrates that L6 exhibits smaller steric hindrance compared to L1, while possessing a stronger electron-withdrawing ability. Notably, the trends observed with the use of L6 in the oxidative addition step are similar to those observed with L1. During the formation of the reductive elimination precursor 10 through carbonyl deinsertion of intermediate 4, the relative free energy increases by 14.6 kcal mol−1 for L1, while only 6.1 kcal mol−1 for L6 (Fig. 6). In the subsequent reductive elimination step, the computed energy barriers are 19.9 kcal mol−1 for L1 and 19.6 kcal mol−1 for L6, showing a minor difference. Examining the overall free energy profiles presented in Fig. 6, it becomes evident that the energy barrier for the entire reaction is 34.5 kcal mol−1 with L1, whereas L6 reduces it to 25.7 kcal mol−1. Key intermediates and transition states governing the reaction rate are intermediate 4, intermediate 10, and 11-ts, prompting a further investigation of the geometry changes from intermediate 4 to 11-ts in Fig. 7. During the process of intermediate 4 through carbonyl deinsertion to form intermediate 10, the substrate fragments progressively approach the phosphine ligand. Consequently, the use of ligands with greater steric hindrance leads to increased steric repulsion in intermediate 10. Subsequently, during the process of intermediate 10 generating the final product through reductive elimination in transition state 11-ts, the product fragments gradually move away from the ligand. Here, intermediate 10 with higher steric repulsion facilitates the reductive elimination process.
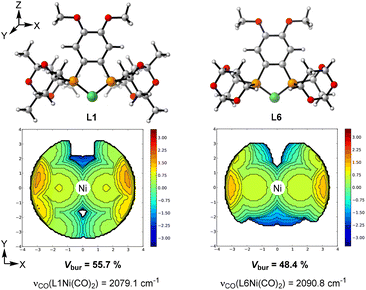 |
| Fig. 5 Comparison of steric and electron effects of ligands L1 and L6. | |
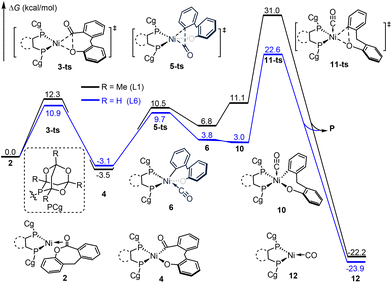 |
| Fig. 6 Comparison of L1 and L6 in nickel-catalyzed decarbonylation of lactone. | |
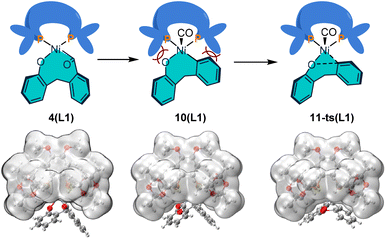 |
| Fig. 7 Geometry changes from intermediate 4(L1) to transition state 11-ts(L1). | |
Therefore, it can be concluded that ligands with large steric hindrance may indeed facilitate the occurrence of reductive elimination; however, this advantage is offset by an increase in the energy of the post-decarbonylation intermediate, rendering it ineffective in promoting the overall reaction. Conversely, ligands characterized by small steric hindrance and strong electron-withdrawing ability demonstrate the capability to significantly accelerate the entire reaction process. This is attributed to their capacity to promote the reductive elimination process, while concurrently reducing the relative energy of the decarbonylated intermediate in comparison to ligands with large steric hindrance.
To verify the above conclusions, we continued to investigate 1,2-bis(ditertiarybutylphosphino)ethane (dtbpe) ligand (L3). As shown in Fig. 8, it is evident that 1,2-bis(dimethylphosphino)ethane (dmpe) ligand (L7) exhibits reduced steric hindrance compared to the dtbpe ligand, yet displays a stronger electron-withdrawing ability. To further assess their respective performance in the decarbonylation reaction, we conducted calculations as depicted in Fig. 9. When employing dtbpe ligand, the entire reaction demonstrated a considerable free energy barrier of 42.7 kcal mol−1. The free energy increment from intermediate 4(L3) to intermediate 10(L3) amounted to 19.3 kcal mol−1, and the energy barrier for reductive elimination was measured at 23.4 kcal mol−1, so the energy barrier of entire reaction is 42.7 kcal mol−1. When using dmpe ligand, the relative free energy of intermediate 4(L7) is increased by 9.0 kcal mol−1 to that of intermediate 10(L7), and the free energy barrier of entire reaction is 34.1 kcal mol−1, which is 8.6 kcal mol−1 lower than that associated with the dtbpe ligand. Therefore, although the bulky dtbpe ligand can facilitate reductive elimination, the formation of intermediate 10(L3) is too difficult. Hence, the utilization of ligands characterized by diminished steric hindrance and enhanced electron-withdrawing ability is more conducive to accelerating the reaction.
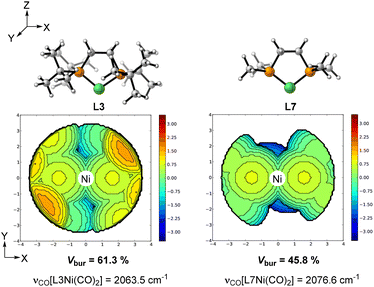 |
| Fig. 8 Comparison of steric and electron effects of ligands L3 and L7. | |
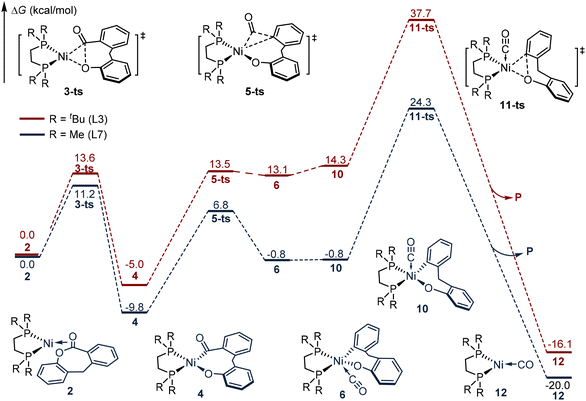 |
| Fig. 9 Comparison of L3 and L7 in nickel-catalyzed decarbonylation of lactone. | |
Conclusions
In summary, our study provides a comprehensive investigation into the mechanism of Ni-catalyzed decarbonylation reactions and the role of phosphine ligands. The most energetically favorable pathway involves Ni(0) initially activating the C(acyl)–O bond of the lactone, followed by a decarbonylation process, and culminating in reductive elimination with the CO coordination, resulting in the formation of the product. AIM analysis reveals that CO coordination weakens the strength of Ni–C and Ni–O bonds, thus reducing the energy barrier of the reductive elimination transition state. Through the analysis of ligand electron effects, we established that ligands with stronger electron-withdrawing abilities facilitate more energetically favorable reductive elimination processes, consequently reducing the overall energy barrier of the reaction. Furthermore, our examination of the steric effects of phosphine ligands demonstrated that ligands with large steric hindrance do not effectively promote the overall decarbonylation process. Although they may facilitate reductive elimination, they also elevate the relative energy of post-decarbonylation intermediate. Consequently, it is the electronic effect, rather than the steric effect, of the ligands that plays a critical role in nickel-catalyzed decarbonylation reactions. Thus, for ligands that fail to promote the reaction, enhancing their electronic effects should be considered to facilitate the reaction. The insights obtained are important for understanding the effects of phosphine ligands in nickel-catalyzed decarbonylation reactions and providing valuable information for the rational design of efficient catalytic systems.
Author contributions
Conceptualization: D. W., H. W., and P. J.; investigation: X. W., P. J., and S. L.; resources: P. J. and S. L.; validation: Y. W. and F. W.; writing – original draft: X. W.; D. W.; software: X. W.; visualization: X. W.; supervision: D. W. and H. W.; writing – review & editing: X. W., D. W., and H. W.
Conflicts of interest
There are no conflicts to declare.
Acknowledgements
We acknowledge the financial support from the National Natural Science Foundation of China (No. 21773214), the Training Plan for Young Key Teachers in Colleges and Universities in Henan Province (2020GGJS016), 111 Project (D20003), and Natural Science Foundation for Excellent Young Scientist in Henan Province (No. 212300410083).
References
- N. Rodríguez and L. J. Goossen, Decarboxylative coupling reactions: a modern strategy for C–C-bond formation, Chem. Soc. Rev., 2011, 40, 5030–5048 RSC.
- C. Liu and M. Szostak, Decarbonylative cross-coupling of amides, Org. Biomol. Chem., 2018, 16, 7998–8010 RSC.
- L. Guo and M. Rueping, Transition-Metal-Catalyzed Decarbonylative Coupling Reactions: Concepts, Classifications, and Applications, Chem. – Eur. J., 2018, 24, 7794–7809 CrossRef CAS PubMed.
- H. Lu, T.-Y. Yu, P.-F. Xu and H. Wei, Selective Decarbonylation via Transition-Metal-Catalyzed Carbon–Carbon Bond Cleavage, Chem. Rev., 2021, 121, 365–411 CrossRef CAS PubMed.
- Ž. Selaković, A. M. Nikolić, V. Ajdačić and I. M. Opsenica, Application of Transition Metal-Catalyzed Decarbonylation of Aldehydes in the Total Synthesis of Natural Products, Eur. J. Org. Chem., 2022, e202101265 CrossRef.
- S. K. Sinha, T. K. Roy, A. Modak and D. Maiti, Enabling the Facile Synthesis of Arenes by Transition Metal Catalyzed Decarbonylation Methodology, Chem. Rec., 2021, 21, 3990–3999 CrossRef CAS PubMed.
- X. Zhang, F. Jordan and M. Szostak, Transition-metal-catalyzed decarbonylation of carboxylic acids to olefins: exploiting acyl C–O activation for the production of high value products, Org. Chem. Front., 2018, 5, 2515–2521 RSC.
- K. Kaneda, H. Azuma, M. Wayaku and S. Tehanishi, Decarbonylation of α-and β-diketones catalyzed by rhodium compounds, Chem. Lett., 1974, 3, 215–216 CrossRef.
- M. Murakami, H. Amii and Y. Ito, Selective activation of carbon–carbon bonds next to a carbonyl group, Nature, 1994, 370, 540–541 CrossRef CAS.
- N. Chatani, Y. Ie, F. Kakiuchi and S. Murai, Ru3(CO)12-Catalyzed Decarbonylative Cleavage of a C−C Bond of Alkyl Phenyl Ketones, J. Am. Chem. Soc., 1999, 121, 8645–8646 CrossRef CAS.
- Z.-Q. Lei, H. Li, Y. Li, X.-S. Zhang, K. Chen, X. Wang, J. Sun and Z.-J. Shi, Extrusion of CO from Aryl Ketones: Rhodium(I)-Catalyzed C–C Bond Cleavage Directed by a Pyridine Group, Angew. Chem., Int. Ed., 2012, 51, 2690–2694 CrossRef CAS PubMed.
- A. Dermenci, R. E. Whittaker, Y. Gao, F. A. Cruz, Z.-X. Yu and G. Dong, Rh-catalyzed decarbonylation of conjugated ynones via carbon–alkyne bond activation: reaction scope and mechanistic exploration via DFT calculations, Chem. Sci., 2015, 6, 3201–3210 RSC.
- P. P. Kaishap, G. Duarah, B. Sarma, D. Chetia and S. Gogoi, Ruthenium(II)-Catalyzed Synthesis of Spirobenzofuranones by a Decarbonylative Annulation Reaction, Angew. Chem., Int. Ed., 2018, 57, 456–460 CrossRef CAS PubMed.
- S. A. Patra, R. Dinda, P. Das Pattanayak and D. Mohapatra, Recent advancements on decarbonylation reactions assisted by Ru-complexes: Synthetic and mechanistic approach, Dalton Trans., 2022, 51, 8571–8582 RSC.
- J. Tsuji and K. Ohno, Organic syntheses by means of noble metal compounds. XXXIV. Carbonylation and decarbonylation reactions catalyzed by palladium, J. Am. Chem. Soc., 1968, 90, 94–98 CrossRef CAS.
- S. Matsubara, Y. Yokota and K. Oshima, Palladium-Catalyzed Decarboxylation and Decarbonylation under Hydrothermal Conditions: Decarboxylative Deuteration, Org. Lett., 2004, 6, 2071–2073 CrossRef CAS PubMed.
- S. Borthakur, S. Baruah, B. Sarma and S. Gogoi, Pd(II)-Catalyzed Synthesis of Alkylidene Phthalides via a Decarbonylative Annulation Reaction, Org. Lett., 2019, 21, 2768–2771 CrossRef CAS PubMed.
- T. Zhou, C.-L. Ji, X. Hong and M. Szostak, Palladium-catalyzed decarbonylative Suzuki–Miyaura cross-coupling of amides by carbon–nitrogen bond activation, Chem. Sci., 2019, 10, 9865–9871 RSC.
- H. Cao, X. Liu, F. Bie, Y. Shi, Y. Han, P. Yan, M. Szostak and C. Liu, General and practical intramolecular decarbonylative coupling of thioesters via palladium catalysis, Org. Chem. Front., 2021, 8, 1587–1592 RSC.
- R. Zeng and G. Dong, Rh-Catalyzed Decarbonylative Coupling with Alkynes via C–C Activation of Isatins, J. Am. Chem. Soc., 2015, 137, 1408–1411 CrossRef CAS PubMed.
- R. Zeng, P.-h. Chen and G. Dong, Efficient Benzimidazolidinone Synthesis via Rhodium-Catalyzed Double-Decarbonylative C–C Activation/Cycloaddition between Isatins and Isocyanates, ACS Catal., 2016, 6, 969–973 CrossRef CAS PubMed.
- W. Guo, S. Wu, T. Wang, Q. Xie, Y. Duan, S. Luo, J. Wang and X. Yu, Rhodium-catalyzed decarbonylative cycloadditions of 1H-indene-1,2,3-triones and alkynes via direct C–C bond activation: divergent synthesis of indenones and quinones, Org. Chem. Front., 2018, 5, 1613–1621 RSC.
- Z.-Q. Lei, F. Pan, H. Li, Y. Li, X.-S. Zhang, K. Chen, X. Wang, Y.-X. Li, J. Sun and Z.-J. Shi, Group Exchange between Ketones and Carboxylic Acids through Directing Group Assisted Rh-Catalyzed Reorganization of Carbon Skeletons, J. Am. Chem. Soc., 2015, 137, 5012–5020 CrossRef CAS PubMed.
- R. J. Somerville and R. Martin, Forging C−C Bonds Through Decarbonylation of Aryl Ketones, Angew. Chem., Int. Ed., 2017, 56, 6708–6710 CrossRef CAS.
- Y. Kajita, S. Matsubara and T. Kurahashi, Nickel-Catalyzed Decarbonylative Addition of Phthalimides to Alkynes, J. Am. Chem. Soc., 2008, 130, 6058–6059 CrossRef CAS PubMed.
- T. Shiba, T. Kurahashi and S. Matsubara, Nickel-Catalyzed Decarbonylative Alkylidenation of Phthalimides with Trimethylsilyl-Substituted Alkynes, J. Am. Chem. Soc., 2013, 135, 13636–13639 CrossRef CAS PubMed.
- T. Morioka, A. Nishizawa, T. Furukawa, M. Tobisu and N. Chatani, Nickel-Mediated Decarbonylation of Simple Unstrained Ketones through the Cleavage of Carbon–Carbon Bonds, J. Am. Chem. Soc., 2017, 139, 1416–1419 CrossRef CAS PubMed.
- T.-T. Zhao, W.-H. Xu, Z.-J. Zheng, P.-F. Xu and H. Wei, Directed Decarbonylation of Unstrained Aryl Ketones via Nickel-Catalyzed C—C Bond Cleavage, J. Am. Chem. Soc., 2018, 140, 586–589 CrossRef CAS PubMed.
- S. Z. Tasker, E. A. Standley and T. F. Jamison, Recent advances in homogeneous nickel catalysis, Nature, 2014, 509, 299–309 CrossRef CAS PubMed.
- V. P. Ananikov, Nickel: The “Spirited Horse” of Transition Metal Catalysis, ACS Catal., 2015, 5, 1964–1971 CrossRef CAS.
- L. Guo and M. Rueping, Decarbonylative Cross-Couplings: Nickel Catalyzed Functional Group Interconversion Strategies for the Construction of Complex Organic Molecules, Acc. Chem. Res., 2018, 51, 1185–1195 CrossRef CAS PubMed.
- S. C. Lee, L. Guo, H. F. Yue, H. H. Liao and M. Rueping, Nickel-Catalyzed Decarbonylative Silylation, Borylation, and Amination of Arylamides via a Deamidative Reaction Pathway, Synlett, 2017, 28, 2594–2598 CrossRef CAS.
- A. Chatupheeraphat, H.-H. Liao, W. Srimontree, L. Guo, Y. Minenkov, A. Poater, L. Cavallo and M. Rueping, Ligand-Controlled Chemoselective C(acyl)–O Bond vs C(aryl)–C Bond Activation of Aromatic Esters in Nickel Catalyzed C(sp2)–C(sp3) Cross-Couplings, J. Am. Chem. Soc., 2018, 140, 3724–3735 CrossRef CAS PubMed.
- C.-L. Ji and X. Hong, Factors Controlling the Reactivity and Chemoselectivity of Resonance Destabilized Amides in Ni-Catalyzed Decarbonylative and Nondecarbonylative Suzuki-Miyaura Coupling, J. Am. Chem. Soc., 2017, 139, 15522–15529 CrossRef CAS PubMed.
- X. Hong, Y. Liang and K. N. Houk, Mechanisms and Origins of Switchable Chemoselectivity of Ni-Catalyzed C(aryl)–O and C(acyl)–O Activation of Aryl Esters with Phosphine Ligands, J. Am. Chem. Soc., 2014, 136, 2017–2025 CrossRef CAS PubMed.
- T. Morioka, S. Nakatani, Y. Sakamoto, T. Kodama, S. Ogoshi, N. Chatani and M. Tobisu, Nickel-catalyzed decarbonylation of N-acylated N-heteroarenes, Chem. Sci., 2019, 10, 6666–6671 RSC.
- S. Nakatani, Y. Ito, S. Sakurai, T. Kodama and M. Tobisu, Nickel-Catalyzed Decarbonylation of Acylsilanes, J. Org. Chem., 2020, 85, 7588–7594 CrossRef CAS PubMed.
- K. Muto, J. Yamaguchi, A. Lei and K. Itami, Isolation, Structure, and Reactivity of an Arylnickel(II) Pivalate Complex in Catalytic C–H/C–O Biaryl Coupling, J. Am. Chem. Soc., 2013, 135, 16384–16387 CrossRef CAS PubMed.
- Q. H. Luu and J. Li, A C-to-O atom-swapping reaction sequence enabled by Ni-catalyzed decarbonylation of lactones, Chem. Sci., 2022, 13, 1095–1100 RSC.
-
M. J. Frisch, G. W. Trucks, H. B. Schlegel, G. E. Scuseria, M. A. Robb, J. R. Cheeseman, G. Scalmani, V. Barone, G. A. Petersson, H. Nakatsuji, X. Li, M. Caricato, A. V. Marenich, J. Bloino, B. G. Janesko, R. Gomperts, B. Mennucci, H. P. Hratchian, J. V. Ortiz, A. F. Izmaylov, J. L. Sonnenberg, D. Williams-Young, F. Ding, F. Lipparini, F. Egidi, J. Goings, B. Peng, A. Petrone, T. Henderson, D. Ranasinghe, V. G. Zakrzewski, J. Gao, N. Rega, G. Zheng, W. Liang, M. Hada, M. Ehara, K. Toyota, R. Fukuda, J. Hasegawa, M. Ishida, T. Nakajima, Y. Honda, O. Kitao, H. Nakai, T. Vreven, K. Throssell, J. A. Montgomery, Jr., J. E. Peralta, F. Ogliaro, M. J. Bearpark, J. J. Heyd, E. N. Brothers, K. N. Kudin, V. N. Staroverov, T. A. Keith, R. Kobayashi, J. Normand, K. Raghavachari, A. P. Rendell, J. C. Burant, S. S. Iyengar, J. Tomasi, M. Cossi, J. M. Millam, M. Klene, C. Adamo, R. Cammi, J. W. Ochterski, R. L. Martin, K. Morokuma, O. Farkas, J. B. Foresman and D. J. Fox, Gaussian 09, Revision B.01, Gaussian, Inc., Wallingford CT, 2010 Search PubMed.
- Y. Zhao and D. G. Truhlar, A new local density functional for main-group thermochemistry, transition metal bonding, thermochemical kinetics, and noncovalent interactions, J. Chem. Phys., 2006, 125, 194101 CrossRef PubMed.
- F. Weigend and R. Ahlrichs, Balanced basis sets of split valence, triple zeta valence and quadruple zeta valence quality for H to Rn: Design and assessment of accuracy, Phys. Chem. Chem. Phys., 2005, 7, 3297–3305 RSC.
- A. V. Marenich, C. J. Cramer and D. G. Truhlar, Universal Solvation Model Based on Solute Electron Density and on a Continuum Model of the Solvent Defined by the Bulk Dielectric Constant and Atomic Surface Tensions, J. Phys. Chem. B, 2009, 113, 6378–6396 CrossRef CAS PubMed.
- G. Luchini, J. Alegre-Requena, I. Funes-Ardoiz and R. Paton, GoodVibes: automated thermochemistry for heterogeneous computational chemistry data [version 1; peer review: 2 approved with reservations], F1000Research, 2020, 9, 291 Search PubMed.
- S. Grimme, Supramolecular Binding Thermodynamics by Dispersion-Corrected Density Functional Theory, Chem. – Eur. J., 2012, 18, 9955–9964 CrossRef CAS PubMed.
- Y.-P. Li, J. Gomes, S. M. Sharada, A. T. Bell and M. Head-Gordon, Improved Force-Field Parameters for QM/MM Simulations of the Energies of Adsorption for Molecules in Zeolites and a Free Rotor Correction to the Rigid Rotor Harmonic Oscillator Model for Adsorption Enthalpies, J. Phys. Chem. C, 2015, 119, 1840–1850 CrossRef CAS.
- L. Falivene, Z. Cao, A. Petta, L. Serra, A. Poater, R. Oliva, V. Scarano and L. Cavallo, Towards the online computer-aided design of catalytic pockets, Nat. Chem., 2019, 11, 872–879 CrossRef CAS PubMed.
- L. Falivene, R. Credendino, A. Poater, A. Petta, L. Serra, R. Oliva, V. Scarano and L. Cavallo, SambVca 2. A Web Tool for Analyzing Catalytic Pockets with Topographic Steric Maps, Organometallics, 2016, 35, 2286–2293 CrossRef CAS.
-
C. Y. Legault, CYLview20, https://www.cylview.org, (accessed February 2023) Search PubMed.
- W. Humphrey, A. Dalke and K. Schulten, VMD: Visual molecular dynamics, J. Mol. Graphics, 1996, 14, 33–38 CrossRef CAS PubMed.
- R. F. W. Bader, Atoms in molecules, Acc. Chem. Res., 1985, 18, 9–15 CrossRef CAS.
- T. Lu and F. Chen, Multiwfn: A multifunctional wavefunction analyzer, J. Comput. Chem., 2012, 33, 580–592 CrossRef CAS PubMed.
- C. A. Tolman, Steric effects of phosphorus ligands in organometallic chemistry and homogeneous catalysis, Chem. Rev., 1977, 77, 313–348 CrossRef CAS.
- M.-N. Birkholz, Z. Freixa and P. W. N. M. van Leeuwen, Bite angle effects of diphosphines in C–C and C–X bond forming cross coupling reactions, Chem. Soc. Rev., 2009, 38, 1099–1118 RSC.
- H. Clavier and S. P. Nolan, Percent buried volume for phosphine and N-heterocyclic carbene ligands: steric properties in organometallic chemistry, Chem. Commun., 2010, 46, 841–861 RSC.
- S. H. Newman-Stonebraker, S. R. Smith, J. E. Borowski, E. Peters, T. Gensch, H. C. Johnson, M. S. Sigman and A. G. Doyle, Univariate classification of phosphine ligation state and reactivity in cross-coupling catalysis, Science, 2021, 374, 301–308 CrossRef CAS PubMed.
- C. A. Tolman, Electron donor-acceptor properties of phosphorus ligands. Substituent additivity, J. Am. Chem. Soc., 1970, 92, 2953–2956 CrossRef CAS.
- T. R. Kégl, N. Pálinkás, L. Kollár and T. Kégl, Computational Characterization of Bidentate P-Donor Ligands: Direct Comparison to Tolman's Electronic Parameters, Molecules, 2018, 23, 3176 CrossRef PubMed.
Footnote |
† Electronic supplementary information (ESI) available: Computational results of less-favored pathways, calculated energies, and Cartesian coordinates of stationary points. See DOI: https://doi.org/10.1039/d3ob01216f |
|
This journal is © The Royal Society of Chemistry 2023 |