DOI:
10.1039/D2OB01919A
(Communication)
Org. Biomol. Chem., 2023,
21, 75-79
Palladium-catalyzed C(sp3)–H nitrooxylation of masked alcohols†
Received
20th October 2022
, Accepted 24th November 2022
First published on 25th November 2022
Abstract
A palladium-catalyzed β-C(sp3)–H nitrooxylation of aliphatic alcohols with AgNO2 is reported. An 8-formylquinoline-derived oxime is installed as an exo-type directing group for sp3 C–H activation and selectfluor acts as the oxidant. The reaction tolerates a variety of functional groups and shows good selectivity for β-C–H nitrooxylation of alcohols.
Introduction
Nitrate esters are of significant interest to synthetic chemists because they are widespread in pharmaceuticals and energetic materials.1 Examples include vasodilators, such as glyceryl trinitrate (GTN) and isosorbide dinitrate (ISDN), postcoital antifertility agents, antidiabetes agents and so on (Scheme 1a).2 The classical ways to prepare nitrate esters are mainly based on two strategies: one is the nitration of alcohols using a mixture of HNO3/H2SO4,1a,3 which causes pollution to the environment. The other is the reaction of a suitable alkyl halide with silver nitrate.4 Recently, a variety of new methods have been developed, including nucleophilic displacement,1a,4a,b,5 ring opening of epoxides,1a,6 alkene difunctionalization,7 diazo compound trapping8 and radical based hydrogen-atom-transfer (HAT) reactions.9 A photocatalytic approach has also been reported with the help of a novel high-valent iodine derivative.10 Despite the successes achieved in the above-mentioned methods, methods to synthesize organic nitrates in a more efficient and straightforward way are still needed.
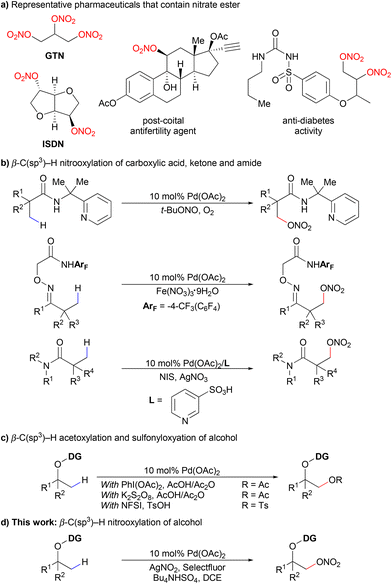 |
| Scheme 1 Pharmaceuticals containing the nitrate group, C–H nitrooxylation reactions and C–H oxidation of alcohols. | |
The strategy of transition metal catalyzed C–H activation has become more and more popular in synthetic chemistry due to its high atom economy and selectivity particularly towards unreactive C–H bonds. Lots of studies on palladium catalyzed C–O bond formation through C–H activation have been reported, mainly including acyloxylation, alkoxylation, or sulfonyloxylation of different substrates.11 In 2020, the Shi group reported the first C(sp3)–H nitrooxylation of carboxylic acids assisted by a bidentate 2-pyridinylisopropyl (PIP) directing group (Scheme 1b).12 Recently, we reported a palladium-catalyzed β-C(sp3)–H nitrooxylation of ketones and amides using practical oxidants (Scheme 1b).13 As far as we know, the direct C(sp3)–H nitrooxylation of alcohols has never been reported via C–H activation.9b
Alcohols are common and ubiquitous structural motifs found in small molecules to complex natural products and materials. The direct C–H bond conversion of alcohols to high value-added molecules is always an attractive choice in synthetic chemistry.14 Various auxiliaries on alcohols have been discovered to promote different C–C and C–X (X = O, N, F and so on) bond formations.15 In terms of C–O bond formation, the Dong group developed β-C(sp3)–H acetoxylation15a and later sulfonyloxylation15b of oxime-masked alcohols via a five-membered exo-palladacycle intermediate. The method was then extended to synthesize cyclic ethers of different ring sizes using a pendant alcohol as an internal nucleophile.15c The Xu group also reported the acetoxylation on primary methyl, methylene, and benzylic C(sp3)–H bonds of alcohol using a different bidentate auxiliary (Scheme 1c).15d Inspired by the above successes, we postulated that the β-C(sp3)–H nitrooxylation of alcohols could be achieved using an appropriate exo-oxime directing group (Scheme 1d).
Results and discussion
We started to verify the hypothesis with oxime-derived 2-butanol (1a) as the initial substrate. Fortunately, the desired product 2a was obtained in 62% yield using AgNO2 as the nitrate source (entry 1, Table 1). Other commonly used nitrate reagents were also screened (entries 2–5). Surprisingly, AgNO3 was less efficient than AgNO2. Then, we explored the efficiency of different aldoxime groups (entries 6–8). DG1 was proved to be the best. Many oxidants were used to improve the yield (entries 9–11), and selectfluor showed better oxidation performance. The addition of 1.0 equiv. of tetrabutyl ammonium hydrogen sulfate (TBAHS) improved the yield to 68% (entry 12). Other phase transfer catalysts were also screened as additives for the reaction, but none of them showed better performance than TBAHS (Table 1, entries 13–15).
Table 1 Optimization of reaction conditionsa,b

|
Entry |
DG |
Nitrate reagent |
Oxidant |
Additive |
Yieldb [%] |
Reaction conditions: 1a (0.1 mmol), Pd(OAc)2 (10 mol%), nitrate reagent (3.0 equiv.), oxidant (1.5 equiv.), additive (1.0 equiv.), DCE (1.5 mL), 80 °C, air, 18 h.
Isolated yield.
|
1 |
DG1 |
AgNO2 |
Selectfluor |
— |
62 |
2 |
DG1 |
AgNO3 |
Selectfluor |
— |
34 |
3 |
DG1 |
TBN |
Selectfluor |
— |
Trace |
4 |
DG1 |
Al(NO3)3·9H2O |
Selectfluor |
— |
Trace |
5 |
DG1 |
Fe(NO3)3·9H2O |
Selectfluor |
— |
Trace |
6 |
DG2 |
AgNO2 |
Selectfluor |
— |
Trace |
7 |
DG3 |
AgNO2 |
Selectfluor |
— |
19 |
8 |
DG4 |
AgNO2 |
Selectfluor |
— |
0 |
9 |
DG1 |
AgNO2 |
BQ |
— |
0 |
10 |
DG1 |
AgNO2 |
NFSI |
— |
34 |
11 |
DG1 |
AgNO2 |
AgF |
— |
31 |
12 |
DG1 |
AgNO2 |
Selectfluor |
Bu4NHSO4(TBAHS) |
68 |
13 |
DG1 |
AgNO2 |
Selectfluor |
Me4NHSO4 |
66 |
14 |
DG1 |
AgNO2 |
Selectfluor |
(NH4)2SO4 |
48 |
15 |
DG1 |
AgNO2 |
Selectfluor |
NH4OAC |
49 |
|
With the optimized conditions in hand, we then explored the scope of the alcohol substrates (Table 2). A variety of nitrooxylation products could be achieved in moderate to excellent yields and with good selectivity for primary β-methyl groups. Various secondary and tertiary aliphatic alcohols with different alkyl chain lengths (2a–2d), tertiary alkyl groups (isopropyl, 2f–2g) and cyclic alkyl groups (cyclohexyl, 2h, and cyclopentyl, 2i) were smoothly nitrooxylated. The di-nitrooxylation product (2e′) was observed with the substrate containing three β-methyl groups, while only the mono-nitrooxylation product (2d) was isolated for the substrate containing two β-methyl groups. For phenyl containing alcohol substrates, nitrooxylation products were obtained in moderate yields (2j–2k) without any C–H functionalization or substitution on the phenyl ring. Methoxy (2l), chloro (2m) and ester (2n–2w) groups were all compatible in the nitrooxylation reaction. Different substituents on the aryl ester groups (2n–2s) were allowed in the reaction. Substrates containing naphthyl ester (2t), styrenyl ester (2u), thiophenyl ester (2v) and cyclopropyl ester (2w) all gave moderate to good yields.
Table 2 Scope of aliphatic alcoholsa,b
Reaction conditions: substrate (0.1 mmol), AgNO2 (3.0 equiv.), Pd(OAc)2 (10 mol%), selectfluor (1.5 equiv.), TBAHS (1.0 equiv.), DCE (1.5 mL), 80 °C, 18 h.
All yields are isolated yields.
The di-nitrooxylation product 2e′ was isolated in 23% yield.
|
|
The nitrate group in the products could be easily converted into other functional groups through substitution reactions. For example, the reaction with NaN3 converted the alkyl nitrate to an alkyl azide (Scheme 2).9a The aldoxime directing group could be efficiently removed via hydrogenation with commercial Pd/C to deliver 1,2-diol and 1,2-amino alcohol which are important structural motifs in organic synthesis.15b
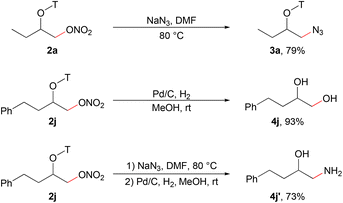 |
| Scheme 2 The conversion of alkyl nitrate and the synthesis of 1,2-diol and 1,2-amino alcohol. | |
Based on our previous work and literature reports,12,13,15b we have proposed a putative Pd(II)/Pd(IV) catalytic cycle for the nitrooxylation reaction (Scheme 3). Substrate coordination followed by C–H bond cleavage gives the bicyclic palladacycle A. The attempt to trap this intermediate to get a stable crystallographic structure failed. Selectfluor could oxidize Ag(I) to Ag(III), which may undergo single electron transfer (SET) to give NO2 radicals.16,17 The NO2 radical could dimerize to form the NO+NO3− ion pair,18 which could oxidize the Pd(II) species A to give the Pd(IV) species B. The nitrosyl-organopalladium(IV) complex B could deliver 2a directly via reductive elimination (the nitrooxylation reaction gave 34% yield of the desired product under a N2 atmosphere, see the ESI†) or react with O2 to afford the O-bound nitrate complex C.19 The more reactive Pd(IV) intermediate C could then undergo reductive elimination to provide 2a.
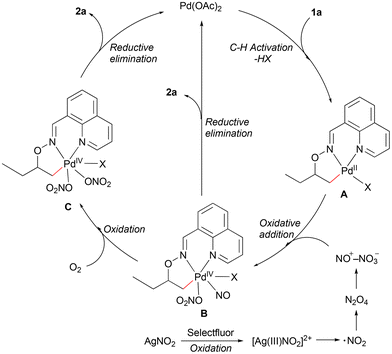 |
| Scheme 3 Plausible mechanism. | |
Conclusions
We have developed a palladium-catalyzed direct alcohol C(sp3)–H nitrooxylation under mild reaction conditions. This protocol was enabled by using selectfluor as the oxidant and AgNO2 as the nitrate source. The reaction shows excellent chemoselectivity, functional group tolerance, and a broad substrate scope. The method provides a new synthetic strategy for the construction of C–O bonds via transition metal catalysis.
Author contributions
Y. X. and R.-B. Z. contributed equally to this work.
Conflicts of interest
The authors declare no competing financial interest.
Acknowledgements
This work was supported by the National Natural Science Foundation of China (NSFC) (Grant No. 21772092 and 22075144). We thank Y.-H. Liu and T.-T. Zhao for preliminary studies on nitrooxylation of alcohols.
References
-
(a) R. Boschan, R. T. Merroxv and R. W. V. Dolah, Chem. Rev., 1955, 55, 485 CrossRef CAS;
(b) P. G. Wang, M. Xian, X. P. Tang, X. J. Wu, Z. Wen, T. W. Cai and A. J. Janczuk, Chem. Rev., 2002, 102, 1091 CrossRef CAS PubMed;
(c)
P. G. Wang, T. B. Cai and N. Taniguchi, Nitric Oxide Donors: For Pharmaceutical and Biological Applications, Wiley-VCH, Weinheim, 2005 CrossRef;
(d) M. Anzini, A. D. Capua, S. Valenti, S. Brogi, M. Rovini, G. Giuliani, A. Cappelli, S. Vomero, L. Chiasserini, A. Sega, G. Poce, G. Giorgi, V. Calderone, A. Martelli, L. Testai, L. Sautebin, A. Rossi, S. Pace, C. Ghelardini, L. Di Cesare Mannelli, V. Benetti, A. Giordani, P. Anzellotti, M. Dovizio, P. Patrignani and M. Biava, J. Med. Chem., 2013, 56, 3191 CrossRef CAS PubMed;
(e) L. M. Barton, J. T. Edwards, E. C. Johnson, E. J. Bukowski, R. C. Sausa, E. F. C. Byrd, J. A. Orlicki, J. J. Sabatini and P. S. Baran, J. Am. Chem. Soc., 2019, 141, 12531 CrossRef CAS PubMed.
-
(a) F. H. Maniyar, T. Sprenger, T. Monteith, C. Schankin and P. J. Goadsby, Brain, 2014, 137, 232 CrossRef PubMed;
(b) J. M. Tarkin and J. C. Kaski, Drugs Ther., 2016, 30, 367 CAS;
(c) N. Ikeda and Y. Hiroi, Global Health Med., 2019, 1, 83 CrossRef;
(d) R. H. Peters, D. F. Crowe, M. A. Avery, W. K. M. Chong and M. Tanabe, J. Med. Chem., 1989, 32, 2306 CrossRef CAS PubMed;
(e) Y. Tamboli, L. Lazzarato, E. Marini, S. Guglielmo, M. Novelli, P. Beffy, P. Masiello, R. Fruttero and A. Gasco, Bioorg. Med. Chem. Lett., 2012, 22, 3810 CrossRef CAS PubMed.
- K. X. Yang, J. D. Artz, J. Lock, C. Sanchez, B. M. Bennett, A. B. Fraser and G. R. J. Thatcher, J. Chem. Soc., Perkin Trans. 1, 1996, 1073 RSC.
-
(a) G. Cainelli, F. Manescalchi, G. Martelli, M. Panunzio and L. Plessi, Tetrahedron Lett., 1985, 26, 3369 CrossRef CAS;
(b) T. Sueda, M. Shoji and K. Nishide, Tetrahedron Lett., 2008, 49, 5070 CrossRef CAS;
(c) R. N. Ram and V. K. Soni, J. Org. Chem., 2015, 80, 8922 CrossRef CAS PubMed;
(d) M. C. Breschi, V. Calderone, M. Digiacomo, M. Macchia, A. Martelli, E. Martinotti, F. Minutolo, S. Rapposelli, A. Rossello, L. Testai and A. Balsamo, J. Med. Chem., 2006, 49, 2628 CrossRef CAS PubMed;
(e) M. Botta, E. Distrutti, A. Mencarelli, M. C. Parlato, F. Raffi, S. Cipriani and S. Fiorucci, ChemMedChem, 2008, 3, 1580 CrossRef CAS.
-
(a) A. McKillop and M. E. Ford, Tetrahedron, 1974, 30, 2467 CrossRef CAS;
(b) J. R. Hwu, K. A. Vyas, H. V. Patel, C. H. Lin and J. C. Yang, Synthesis, 1994, 471 CrossRef.
-
(a) R. M. A. Pinto, J. A. R. Salvador and C. L. Roux, Tetrahedron, 2007, 63, 9221 CrossRef CAS;
(b) B. Das, M. Krishnaiah, K. Venkateswarlu and V. S. Reddy, Helv. Chim. Acta, 2007, 90, 110 CrossRef CAS;
(c) Y. A. Volkova, O. A. Ivanova, E. M. Budynina, E. B. Averina, T. S. Kuznetsova and N. S. Zefirov, Tetrahedron Lett., 2008, 49, 3935 CrossRef CAS;
(d) H. I. Iranpoor and I. M. Baltork, Synth. Commun., 1990, 20, 2789 CrossRef;
(e) Z. Liu, R. Li, D. Yang and L. Wu, Tetrahedron Lett., 2004, 45, 1565 CrossRef CAS;
(f) P. Guiding, R. W. Millar, N. C. Paul and D. H. Richards, Tetrahedron Lett., 1988, 29, 2731 CrossRef.
-
(a) T. Taniguchi, A. Yajima and H. Ishibashi, Adv. Synth. Catal., 2011, 353, 2643 CrossRef CAS;
(b) R. Bag, D. Sar and T. Punniyamurthy, ACS Omega, 2017, 2, 6278 CrossRef CAS PubMed.
- S. Thurow, A. A. G. Fernandes, Y. Quevedo-Acosta, M. F. de Oliveira, M. G. de Oliveira and I. D. Jurberg, Org. Lett., 2019, 21, 6909 CrossRef CAS PubMed.
-
(a) S. Kamijo, Y. Amaoka and M. Inoue, Tetrahedron Lett., 2011, 52, 4654 CrossRef CAS;
(b) J. Z. Ni, J. Ozawa, K. Oisaki and M. Kanai, Org. Biomol. Chem., 2016, 14, 4378 RSC.
- R. Calvo, A. L. Tellier, T. Nauser, D. Rombach, D. Nater and D. Katayev, Angew. Chem., Int. Ed., 2020, 59, 17162 CrossRef CAS.
- For selected examples of palladium catalyzed C–O bond formation through C–H activation, see:
(a) J. L. Bras and J. Muzart, Eur. J. Org. Chem., 2018, 1176 CrossRef;
(b) S. Moghimi, M. Mahdavi, A. Shafiee and A. Foroumadi, Eur. J. Org. Chem., 2016, 3282 CrossRef CAS;
(c) I. B. Krylov, V. A. Vil’ and A. O. Terent'ev, Beilstein J. Org. Chem., 2015, 11, 92 CrossRef;
(d) Y. Q. Li, Q. L. Yang, P. Fang, T. S. Mei and D. Y. Zhang, Org. Lett., 2017, 19, 2905 CrossRef CAS;
(e) M. H. Li, M. Shang, H. Xu, X. Wang, H. X. Dai and J. Q. Yu, Org. Lett., 2019, 21, 540 CrossRef CAS PubMed;
(f) Z. Zhuang, A. N. Herron, Z. L. Fan and J. Q. Yu, J. Am. Chem. Soc., 2020, 142, 6769 CrossRef CAS;
(g) K. K. Ghosh, A. Uttry, A. Koldemir, M. Ong and M. V. Gemmeren, Org. Lett., 2019, 21, 7154 CrossRef CAS;
(h) Z. Ren, J. E. Schulz and G. B. Dong, Org. Lett., 2015, 17, 2696 CrossRef CAS;
(i) J. Kumar, A. Gupta and S. Bhadra, Org. Biomol. Chem., 2019, 17, 3314 RSC;
(j) F. Li, Y. R. Zhou, H. Yang, Z. Q. Wang, Q. Q. Yu and F. L. Zhang, Org. Lett., 2019, 21, 3692 CrossRef CAS.
- B. Li, Y. Q. Han, X. Yang and B. F. Shi, Org. Lett., 2020, 22, 9719 CrossRef CAS PubMed.
- Y. Xue, S. P. Han, C. Jiang and J. Q. Yu, ACS Catal., 2021, 11, 14188 CrossRef CAS.
-
(a) Y. Xu and G. B. Dong, Chem. Sci., 2018, 9, 1424 RSC;
(b) F. Mo, J. R. Tabor and G. B. Dong, Chem. Lett., 2014, 43, 264 CrossRef CAS.
-
(a) Z. Ren, F. Y. Mo and G. B. Dong, J. Am. Chem. Soc., 2012, 134, 16991 CrossRef CAS PubMed;
(b) Y. Xu, G. Yan, Z. Ren and G. B. Dong, Nat. Chem., 2015, 7, 829 CrossRef CAS PubMed;
(c) S. J. Thompson, D. Q. Thach and G. B. Dong, J. Am. Chem. Soc., 2015, 137, 11586 CrossRef CAS;
(d) B. X. Wang, Y. J. Mao, H. Y. Hao, Q. Z. Wu, K. Zhou, S. J. Lou and D. Q. Xu, Chem. Commun., 2019, 55, 7049 RSC;
(e) L. Jin, X. Zeng, S. Li, X. Hong, G. Qiu and P. Liu, Chem. Commun., 2017, 53, 3986 RSC;
(f) T. Kang, H. Kim, J. G. Kim and S. Chang, Chem. Commun., 2014, 50, 12073 RSC;
(g) Y. Dong and G. Liu, J. Org. Chem., 2017, 82, 3864 CrossRef CAS;
(h) Y. J. Mao, S. J. Lou, H. Y. Hao and D. Q. Xu, Angew. Chem., Int. Ed., 2018, 57, 14085 CrossRef CAS PubMed;
(i) G. Q. Xia, Z. Zhuang, L. Y. Liu, S. L. Schreiber, B. Melillo and J. Q. Yu, Angew. Chem., Int. Ed., 2020, 59, 7783 CrossRef CAS;
(j) G. Q. Xia, J. Weng, L. Y. Liu, P. Verma, Z. Q. Li and J. Q. Yu, Nat. Chem., 2019, 11, 571 CrossRef CAS.
-
(a) F. Yin, Z. T. Wang, Z. D. Li and C. Z. Li, J. Am. Chem. Soc., 2012, 134, 10401 CrossRef CAS;
(b) Z. D. Li, Z. T. Wang, L. Zhu, X. Q. Tan and C. Z. Li, J. Am. Chem. Soc., 2014, 136, 16439 CrossRef CAS.
-
(a) Y. K. Liu, S. J. Lou, D. Q. Xu and Z. Y. Xu, Chem. – Eur. J., 2010, 16, 13590 CrossRef CAS PubMed;
(b) W. Zhang, S. Lou, Y. Liu and Z. Xu, J. Org. Chem., 2013, 78, 5932 CrossRef CAS PubMed;
(c) W. Zhang, D. Wu, J. Zhang and Y. Liu, Eur. J. Org. Chem., 2014 Search PubMed;
(d) Z. Fan, J. Ni and A. Zhang, J. Am. Chem. Soc., 2016, 138, 8470 CrossRef CAS.
-
(a) A. Shoberu, C. K. Li, Z. K. Tao, G. Y. Zhang and J. P. Zou, Adv. Synth. Catal., 2019, 361, 2255 CrossRef CAS;
(b) P. Astolfi, M. Panagiotaki and L. Greci, Eur. J. Org. Chem., 2005, 3052 CrossRef CAS;
(c) B. C. Challis and S. A. Kyrtopoulos, J. Chem. Soc., Perkin Trans. 2, 1978, 1296 RSC;
(d) S. Oae, Y. H. Kiu, D. Fukushima and K. Shinhama, J. Chem. Soc., Perkin Trans. 1, 1978, 913 RSC;
(e) C. H. Bibart and G. E. Ewing, J. Chem. Phys., 1974, 61, 1284 CrossRef CAS;
(f) B. W. McLelland, G. Gunderson and K. Hudberg, J. Chem. Phys., 1972, 56, 4541 CrossRef.
-
(a) S. L. Zultanski and S. S. Stahl, J. Organomet. Chem., 2015, 793, 263 CrossRef CAS;
(b) J. Cámpora, P. Palma, D. del Río and E. Carmona, Organometallics, 2003, 22, 3345 CrossRef;
(c) B. Galliker, R. Kissner, T. Nauser and W. H. Koppenol, Chem. – Eur. J., 2009, 15, 6161 CrossRef CAS PubMed.
|
This journal is © The Royal Society of Chemistry 2023 |
Click here to see how this site uses Cookies. View our privacy policy here.