DOI:
10.1039/D3NR03016D
(Review Article)
Nanoscale, 2023,
15, 14284-14300
Anti-inflammatory potential of platinum nanozymes: mechanisms and perspectives
Received
23rd June 2023
, Accepted 31st July 2023
First published on 1st August 2023
Abstract
Inflammation is a complex process of the body in response to pathogen infections or dysregulated metabolism, involving the recruitment and activation of immune system components. Repeated dangerous stimuli or uncontrolled immune effector mechanisms can result in tissue injury. Reactive Oxygen Species (ROS) play key roles in physiological cell signaling as well as in the destruction of internalized pathogens. However, aberrant ROS production and release have deleterious effects on the surrounding environment, making ROS regulation a priority to reduce inflammation. Most of the current anti-inflammatory therapies rely on drugs that impair the release of pro-inflammatory mediators. Nevertheless, increasing the enzymatic activity to reduce ROS levels could be an alternative or complementary therapeutic approach to decrease inflammation. Nanozymes are nanomaterials with high catalytic activity that mimic natural enzymes, allowing biochemical reactions to take place. Such functional particles typically show different and regenerable oxidation states or catalytically reactive surfaces offering long-term activity and stability. In this scenario, platinum-based nanozymes (PtNZs) exhibit broad and efficient catalytic functionalities and can reduce inflammation mainly through ROS scavenging, e.g. by catalase and superoxide dismutase reactions. Dose-dependent biocompatibility and immune compatibility of PtNZs have been shown in different cells and tissues, both in vitro and in vivo. Size/shape/surface engineering of the nanozymes could also potentiate their efficacy to act at different sites and/or steps of the inflammation process, such as cytokine removal or specific targeting of activated leukocytes. In the present review, we analyze key inflammation triggering processes and the effects of platinum nanozymes under exemplificative inflammatory conditions. We further discuss potential platinum nanozyme design and improvements to modulate and expand their anti-inflammatory action.
1. Introduction
The human body continually receives internal and external dangerous stimuli potentially inducing pathological states. External physical and chemical factors, such as UV rays or smoke particulate, hit skin and mucosal cells, eventually cause damage. In addition, microbes like viruses and bacteria penetrate and colonize different tissues inducing harmful effects. Pathogens also dysregulate cellular signaling, eliciting the aberrant production of reactive molecular mediators (Fig. 1). Similarly, many abnormal biochemical pathways can be activated by erroneous transcriptional indications of mutated genes, as shown in genetic disease outcomes. All these factors often induce the overproduction of reactive oxygen species (ROS) triggering inflammation, which in turn enhances them in a feedforward mechanism.
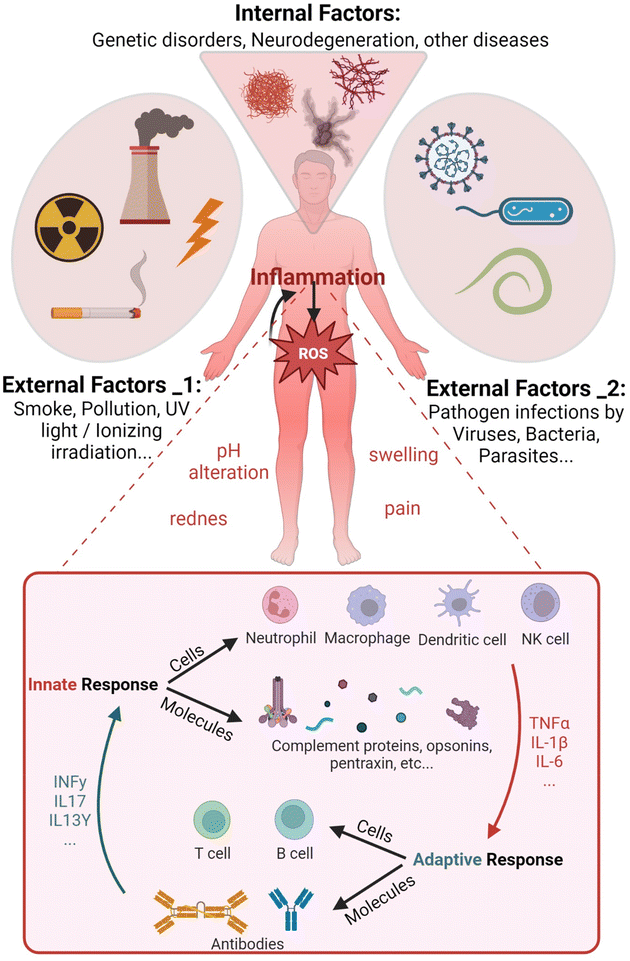 |
| Fig. 1 Schematic illustrating examples of several external and internal causes potentially able to trigger inflammation reactions in humans. Abnormally high ROS levels can induce or can be induced by inflammation. (Inset) Scheme of the innate immune cellular and molecular immune response and its main cross-talk mediators with the adaptive immune system during inflammation. | |
Inflammation is defined as the recruitment of leukocytes and specific plasma proteins towards sites of infection or tissue damage, causing pathological alterations in the body homeostasis.1 Inflammation involves diverse and fast responding mediators including specific leukocytes and molecules, altogether described as the innate immune system (IIS) (see Fig. 1 inset). The adjective “innate” is useful to distinguish a non-specific native immune response driven by phagocytic cells like neutrophils and macrophages from the fine-tuned lymphocyte-mediated “adaptive” immunity.2 However, the cross-talk between innate and adaptive systems is fundamental for a complete response towards pathogens. Major changes from the physiological state characterize inflamed tissues. Particularly, pH alterations of the extracellular fluid occur as a result of infiltration and activation of effector cells in the tissue (i.e. extracellular acidosis).3 The extracellular concentration of released molecules like antibodies, activated complement components and cytokines increases together with the protein expression on the immune cell surfaces.4
ROS are central signaling molecules primarily involved in the regulation of physiological homeostasis5 as well as in the progression of inflammatory disorders.6 Cell damage and unbalanced metabolism are often due to an increased ROS concentration, a process known as oxidative stress.7 Indeed, oxidative stress elicits immune system activation in many ways as a consequence of damaged lipid or protein structures, either inside cells or in the extracellular media. Therefore, antioxidant agents regulating ROS activity can be promising anti-inflammatory candidates for novel therapies in addition to steroidal and non-steroidal drugs. In particular, non-steroidal anti-inflammatory drugs (NSAIDs) aim at impairing pro-inflammatory mediator pathways by inhibiting enzymatic synthesis of prostaglandins, which are commonly associated with the side effects affecting the gastrointestinal, cardiovascular and renal systems, despite the several billion doses consumed annually worldwide.8,9
Some nanomaterials (NMs) can exhibit superior antioxidant properties. Among others (e.g., carbon dots,10 cerium oxide,11 metal oxides,12,13 gold NMs14), platinum nanoparticles (PtNPs) are emerging due to their remarkable catalytic activity and ROS scavenging ability in cells and tissues.15,16 Indeed, PtNPs can mimic natural enzymes such as catalase (CAT), superoxide dismutase (SOD), and peroxidases (POD); for this reason, they are also called “nanoenzymes” or “nanozymes” (NZs).17,18 PtNZs hold great potential and may represent valuable substitutes for natural enzymes as a treatment for pathological conditions characterized by ROS-mediated inflammation. Their efficiency depends on different features, including size, shape, and surface chemistry.19 The latter is extremely important for biomedical purposes and the tissue environment where PtNZs should work. In the case of inflammatory disorders, PtNZ immune compatibility and specific extracellular or intracellular localization are crucial to catalyze ROS reduction and decrease the immune reaction. Applications of PtNZs have been, so far, mainly explored in vitro,16 although some experiments in vivo confirmed ROS scavenging and anti-inflammatory activities.20,21
In the present review article, we analyze the main mechanisms generating inflammation, ROS involvement, and the anti-inflammatory actions of PtNZs at different levels of the immune reactions. We evaluate possible strategies to amplify PtNZ effects by modification of the NP features, which could potentiate ROS modulation and increase their efficiency.
2. Mechanisms triggering cellular inflammatory responses and ROS overproduction
2.1. Intracellular ROS generation
Inflammation is linked to excessive intracellular ROS synthesis overwhelming the capacity of the cell to remove ROS before being damaged.22 ROS production occurs at different intracellular locations depending on the cellular metabolic and functional activity (Fig. 2A). The biological process by which ROS are generated and released is also defined as “respiratory burst” or oxidative stress. Some authors refer to “oxidative eustress” when the production of signaling ROS does not overcome physiological concentrations (i.e. 1–10 nM H2O2) and “oxidative distress” when supraphysiological concentrations occur (i.e. >100 nM H2O2).23 Different organelles act as the cellular sites for ROS synthesis catalyzed by membrane-bound enzymes, releasing ROS in their inner side or in the cytosol. NADPH oxidases (NOXs) are the main multi-subunit protein complexes reducing molecular oxygen into ROS at cell membranes and within phagosomes24 (Fig. 2A (1), (2)). ROS perform several physiological functions including the regulation of cellular signaling and immunity, besides playing a key role in wiping out foreign pathogens.25 Metabolic dysregulation of energy consumption mechanisms, like oxidation of fatty acids in obese people, lead to boosted mitochondrial activity and peroxisomal oxidase activation, both contributing to inflammation by different mechanisms26 (Fig. 2A (1), (3)). In the mitochondrial electron transport chain (ETC) enzymatic cascade, from membrane-embedded four carriers (from complex I to complex IV), 1–2% of the electrons leak out and react with O2 producing ˙O2− in the cytosol27 that compromise intracellular molecules if not properly buffered by antioxidants.
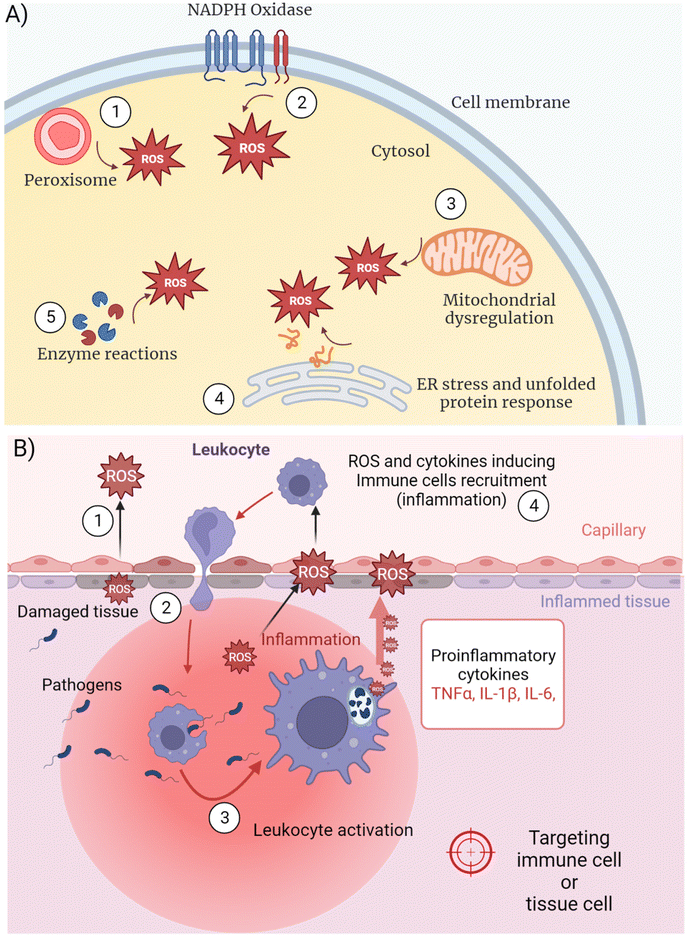 |
| Fig. 2 (A) Schematics of the main organelles involved in the regulation of the ROS level. ROS overproduction and inflammation states can be triggered via organelle dysregulation caused by genetic and external factors. (B) Macroscopic representation of an inflammatory reaction involving immune cells and the surrounding tissue. | |
In general, immune cell activation occurring during inflammatory reactions require increased gene transcription and protein synthesis. The impairment of intracellular physiology depends on many different mechanisms related to the complexity of the cell machinery and diverse chemical reactions. Since all the biochemical pathways need precise enzyme assembly in defined intracellular locations, any modification of the protein organization could dramatically alter cell homeostasis. Together with the previously described ROS production by cell membrane-localized NOX complexes, peroxisome oxidase and mitochondrial ETC, a fourth major mechanism of oxygen reduction leading to inflammation occurs in the endoplasmic reticulum (ER) (Fig. 2A (4)). The ER is studded with ribosomes translating the mRNAs coming from the nucleus and playing a central role in correct protein synthesis.28 The post-translational polypeptide conformation is an energy-consuming process that requires redox conditions to allow the formation of intramolecular and intermolecular disulphide bridges for precise protein folding.29 Inside the ER, the oxidation of cysteine–thiol groups is catalyzed by two enzymes, protein-disulphide isomerase (PDI) and ER oxidoreductin 1 (ERO1)30 that use O2 as the final recipient of the electrons, ultimately producing ROS. Prolonged cellular stress or fast proliferation and differentiation processes (i.e. leukocyte immune activation) force the cell to synthesize more proteins, resulting in ROS overproduction as a consequence. Moreover, unbalanced antioxidant mechanisms can induce further oxidative stress (Fig. 2A (5)), due to the depletion of reduced glutathione in the ER that competes with the oxidation of protein thiols.31,32 The following accumulation of huge amounts of proteins in the ER, many of which are misfolded because of an improper disulphide-bond formation, stimulates the unfolded protein response (UPR).33 The latter is a set of signaling pathways activating a specific transcriptional program to resolve protein misfolding and eliminate cell injury, including NFκB-mediated transcription of pro-inflammatory mediators. Furthermore, ROS alter the degradation of proteins by causing irreversible changes in the amino acids, such as the hydroxylation of aromatic groups and aliphatic side chains, leading to pro-inflammatory gene expression.34
2.2. ROS release and inflammation
Any type of tissue injury or genetic mutation-induced metabolic dysregulation evokes the production of ROS and several pro-inflammatory mediators to recruit leukocytes, which forward the increased ROS synthesis already in place in the damaged tissue (Fig. 2B). Among ROS, H2O2 can cross the cell membrane damaging it with Fenton reaction-produced radicals,35 besides moving into the extracellular space (Fig. 2B (1)). Endothelial cell-generated ROS released in the bloodstream activate leukocytes, which in turn enhance the inflammatory process (Fig. 2B (2)). Immune cell migration and pro-inflammatory mediators can amplify ROS overproduction leading to systemic and often uncontrolled tissue inflammation. IIS leukocytes can sense pathological modifications of the tissues as damage-associated molecular patterns (DAMPs).36 Nuclear proteins, secondary stress-induced proteins or crystals (e.g. monosodium urate) are recognized by DAMP receptors that initiate an immune response, as well as pattern-recognition receptors (PRRs) binding to pathogen-associated molecular patterns (PAMPs).
Several cells and biochemical mediators of the IIS are diffusely distributed in different tissues. Phagocytes like neutrophils,37 macrophages38 and dendritic cells (DCs)39 (DCs link innate and adaptive immune response) intercept infective microorganisms by recognizing specific molecular structures (PAMPs) by means of PRRs. After entrapping the pathogens within intracellular compartments called phago-lysosomes (phagosomes), phagocytes demolish the microorganisms with specific enzymatic reactions, including ROS production40 (Fig. 2B (3)). Notwithstanding the direct pathogen–immune cell interaction, further amplification of inflammation could be induced by externally damaged cellular structures sensed by another type of IIS leukocyte, the natural killer (NK) cells.41 Although NKs recognize infected or stressed cells and kill them by releasing granules containing enzymes that facilitate signal leading to targeted cell apoptosis, NKs also release pro-inflammatory cytokines that activate monocytes/macrophages enhancing inflammation.42
The well-known intracellular signaling pathway of toll-like receptors (TLRs), a major class of PPRs, can help us in understanding the link between damage/infection and the role of ROS.43 Dimers of TLRs are differentially expressed on the external and inner-endosomal leukocyte membranes. The ones on the cell surface are able to recognize “non-self” external structures, such as the Gram-negative bacterial wall component lipopolysaccharide (LPS), the lipoteichoic acid present on the wall of Gram-positive bacteria or the fungal mannose-rich oligosaccharides. On the other hand, TLRs expressed in the endosomes can distinguish the diverse assembly of viral nucleic acids. Upon ligand binding, TLRs start intracellular pathways stimulating gene transcription44 and activating several enzymes including the phagocyte NOXs.24 TLRs activate NOXs upon ligand binding, likely through direct interaction between their subunits, as an essential step of their signal transduction.45–47
Other important sensors of PAMPs and DAMPs are the cytoplasmic NOD-like receptors (NLRs), in particular the NLRP subfamily, which forms inflammasomes, enzymatic complexes that generate important pro-inflammatory cytokines, such as IL-1β48 (see the following). Inflammasome complexes are directly regulated by ROS mainly produced by the mitochondria (Fig. 2A) during electron transfer.27 Key indications regarding inflammasome activation by mitochondria-derived ROS have been shown by studies on the macrophage NLRP3 cytosolic receptor and its upstream NF-kB transcription factor stimulation pathway.49 In particular, the inhibition of mitochondrial function impairs NLRP3 activation and the following pro-inflammatory cytokine synthesis.
IIS stimulation triggered by PAMPs, DAMPs or aberrant physiological reactions is regulated by mediators that amplify, sustain and finally turn off the response (Fig. 2B (4)). Most of them are soluble cytokines and chemokines released by the same activated IIS phagocytes or by infected/damaged tissue cells.50 Tumor necrosis factor-alpha (TNF-α), interleukin-1β (IL-1β) and interleukin-6 (IL-6) are mostly responsible for generating acute inflammation with potential systemic pathological consequences. TNF-α is strongly released by activated mononuclear phagocytes in response to bacteria and other microbes through PAMPs, cell damage through DAMPs or genetic mutations.51 Upon TNF-receptors’ binding, downstream signal transduction pathways induce further pro-inflammatory gene transcription in other IIS, endothelial and epithelial cells overexpressing IL-1β, IL6 and different chemokines to recruit blood neutrophils (i.e. CXCL1 and CXCL8) and monocytes (i.e. CCL2 and CCL5).
Cytokine-activated endothelia of the postcapillary venules express tissue-specific adhesion molecules stopping leukocytes at the site of inflammation in a multistep process before their extravasation into the underneath tissue.52 Low affinity binding of leukocytes is mediated by selectins up-regulated onto endothelial cells in response to TNF-α, IL-1β or other pro-inflammatory cytokines.53 Whereas selectins bind carbohydrate molecules on neutrophils and monocytes characterizing their “rolling-step”, stable leukocyte adhesion is achieved through another class of heterodimeric proteins named integrins.54 The two non-covalently linked polypeptide chains of integrins modify their conformation from a low-affinity bent state to a chemokine-induced extended structure with high-affinity for the cognate binding molecules on the specific tissue cells. Ultimately, chemokine-mediated leukocyte polarization allows blood extravasation and localization into the inflamed tissue.55 Significant evidence indicates that the leukocyte extravasation process driven by selectins, integrins and chemokines is regulated by ROS production.56 Upon receiving inflammatory stimuli of infection and damage, the ROS produced in endothelial cells induce the expression of selectins through transcription-independent mechanism followed by transcription-dependent integrin up-regulation. The latter is promoted by pro-inflammatory cytokines, such as IL-1β, released by the same endothelial cells and reinforced with the cytokines released by the rolling leukocytes. Actually, TNF-α, IL-1β and IL6 released in the bloodstream act on different organs and are responsible for systemic inflammatory diseases.57 All these cytokines increase the synthesis of prostaglandins in the hypothalamus acting as endogenous pyrogens to induce fever. In addition, very low concentrations of TNF-α and IL-1β are sufficient to up-regulate the production of fibrinogen and pentraxins in hepatocytes.58,59 During severe systemic inflammation, large and prolonged amounts of TNF-α can additionally induce pathological anomalies, such as inhibition of myocardial contractility and vascular muscle tone causing blood pressure fall (i.e. shock) or overexpression of activators of coagulation in endothelial cells inducing thrombosis. Abnormal systemic release of proinflammatory cytokines, commonly defined as “cytokine storm”, is a well known consequence of bacterial-induced septic shock60 and it has recently emphasized its dramatic costs during COVID-19 pandemics, as a distinctive sign of SarsCov2 infection.61
3. Platinum nanozymes
All the inflammatory mechanisms reported above are generally treated with anti-inflammatory molecules with different chemical structures and different targets. The catalytic properties of PtNZs can also be exploited to impair inflammatory processes, especially the ROS mediated ones.
PtNPs already have industrial applications, and in some countries, such as Japan, PtNPs are already employed in commercially available food supplements, skin care creams, and other cosmetic products. Therefore, PtNPs are already one of the most accessible nanomaterials to humans; in Europe, where regulations are more restrictive, there are still some concerns about their safety, and consequently their application is mainly limited to the health-unrelated industry (e.g., automotive).
3.1. Bio- and immune compatibility of PtNZs
Biocompatibility is a general concept that often leads to wrong public conclusions. In general, a substance that does not induce toxicity or cause permanent tissue damage within a certain dose range can be considered biocompatible.62 Besides the material dose and excluding contaminations related to synthesis methods,63 metal-NP toxicity depends on their size, shape, dispersion, dissolution (i.e., release of ions), and surface chemistry.64 In particular, NP toxicity to immune cells and exacerbation of inflammatory processes (immune compatibility) should be considered for immunological applications.65
Our group assessed the potential toxicity of pure PtNZ suspensions by observing their high intracellular uptake in the endolysosomal compartments and proving their cytocompatibility.64,66,67 Clean/pure PtNZ suspensions, not containing reaction side-products, ions or endotoxins, can be internalized and tolerated at high doses. Unlike other metal-NPs (i.e. AgNPs), PtNPs are stable in the harsh lysosomal environment, a necessary prerequisite for the intracellular application of PtNZ catalytic activity. Further work from our laboratory demonstrated the PtNZ compatibility with monocyte/macrophage or neutrophilic promyelocytic cells,68,69 which play crucial roles in early and late steps of inflammation. In our observations, 5 or 20 nm citrate-coated PtNZs did not decrease the viability of the tested cells up to high concentrations (100 μg mL−1). Interestingly, PtNZs internalized in THP-1 monocytic cells exhibited catalytic activity and modulation of genes involved in bacterial LPS-induced signaling without significantly affecting immune cell performances.69 Furthermore, the same PtNZ concentration did not impair the monocyte-to-macrophage transition in an in vitro cell differentiation model.68 We concluded that 5 and 20 nm citrate-coated PtNZs can be safely used in vitro up to a concentration of 50 μg mL−1. Nevertheless, particle shape-dependent immune activation (as for other NMs) cannot be excluded a priori for PtNPs,70 as certain particle structure have been shown to play a critical role in eliciting an immune response (i.e., acting as an adjuvant).70,71 The biocompatibility of citrate-coated 50 nm PtNPs was also proved in a brain ex vivo model of excitotoxicity.72 The particles did not severely impact the viability of neurons and glia; however, PtNPs induced morphological changes in microglia with the loss of ramification and increased the expression of ionized calcium-binding adaptor molecule-1 (IBA-1). The amoeboid shape and IBA-1 up-regulation of these brain-resident immune cells are usually interpreted as a sign of inflammation. Nonetheless, the authors concluded that PtNPs with a size of 50 nm could be tolerated by the brain parenchyma cells, indicated by the absence of astrogliosis and neuronal damage. They also demonstrated that the particles did not exacerbate kainate-induced cytotoxicity. No indications of proinflammatory cytokine release have been reported, which could discriminate whether the morphological alteration of microglia was due to a pro-inflammatory activation or a membrane adaptation to PtNP contact and internalization. Indeed, the administration of PtNPs can reduce the adhesion of BV-2 murine microglial cell line and increase phagocytosis, which is always accompanied by remarkable cell shape changes.73 Human primary neutrophils and eosinophils have been investigated in a sex-based analysis following exposure to sub-10 nm size PtNPs among different particles.74 The results indicate that PtNPs did not trigger apoptotic cell death up to 100 μg mL−1. Data collected from experiments using primary human blood cells and granulocytes reported in this work confirm the biocompatibility range of NP-size and the maximal concentration to safely apply PtNZs for anti-inflammatory purposes.
In vivo experimental data reported in the literature regarding PtNZ administration refer to diverse administration protocols, doses, routes, and suspensions, which make it difficult to draw conclusions on particle immune compatibility for translational applications. Some pro-inflammatory effects have been documented after a single 1 mg kg−1 dose by intratracheal instillation in mice,75 whereas other publications show anti-inflammatory outcomes by intranasal administration for three days21 or intragastric gavage for 8 days.20 Anyway, no PtNP-induced physiological nor immunological dramatic impairments in vivo have been documented so far in the literature after the release of well characterized particles at reasonable final concentration. Although many viability tests have been performed, as summarized in systematic reviews considering several types of platinum nanomaterial applications in biomedicine,15,64,76 the specific investigation of PtNZ immune-toxicology in vivo remains limited. We deem it important to stress that, when involving therapeutic nanomaterials, a case-by-case study is often required. Although further research will be necessary to better understand in depth the potential noxious effects on the immune system by specific PtNZ sizes, shapes, and surface coatings, significant PtNZ anti-inflammatory effects in specific tissue diseases have been described in the literature (see the following).
3.2. PtNP CAT- and SOD-like activity
From the literature, we could basically distinguish two main types of the so-called NZs. In one type, the NP mainly serves as a carrier/support for the immobilization of small molecular catalysts, often hosted in the ligand shell. In the second type, the NZ (and in particular its surface atoms) are intrinsically able to perform catalytic reactions. In both cases, evaluating potential interference (i.e., interactions with salts or biomolecules) from the biological environment is of utmost importance. In particular when the performance of the NZs totally depends on the availability of the reactive surface (intrinsic NZ property), these aspects must be considered when designing a therapeutic strategy.
PtNPs can present different intrinsic oxidoreductase-like activities (type 2 NZ), including oxidase (OX), peroxidase (POD), CAT, and SOD. While OX-like activity can be considered a pro-oxidative function for PtNPs, CAT and SOD can remove the ROS or reduce their reactivity. While POD enzymes are often considered to act as ROS scavengers since removing a non-specific strong oxidant like H2O2 from the environment forming a milder and specific oxidant (activated enzyme), concerning POD-like activity of NPs presenting radical reaction intermediate there are controversial opinions.
Nevertheless, except for cancer cells presenting a particular metabolism, commonly, in normal healthy cells there is no evidence of PtNPs-induced oxidative damage. In contrast, as previously mentioned, there is now significant in vitro evidence that PtNPs can protect from oxidative stress.
Generated under the control of growth factors and cytokines (by more than 40 enzymes), hydrogen peroxide (H2O2) and superoxide anion radicals (˙O2−), are the key redox signaling agents.5 In the case of abnormally high ROS levels, H2O2 and ˙O2− are most likely to be involved. H2O2 in particular is considered the main signaling unit,23 interacting with protein targets, which engage in metabolic regulation and stress responses. CAT activity catalyzes the decomposition reaction of hydrogen peroxide into molecular oxygen and water, while SOD activity catalyzes the conversion of ˙O2− into oxygen and H2O2.
PtNZs were shown to mimic both these enzymes without the need for specially prepared surface functionalization, which means that SOD and CAT can occur as a tandem reaction directly onto the NP surface (see Fig. 2). A recently published review article delineates some interesting hypotheses behind CAT mechanism of different nanozymes.77 However, there is still no clear univocal understanding of the mechanism behind PtNZ CAT77 and SOD activity.
In vitro results, apart from a few exceptions, showed an energy-dependent uptake of the PtNZs, following an endolysosomal internalization pathway.78 Interestingly, the intracellular ROS scavenging activity occurs in the lysosome, where the PtNZs accumulate, acting similarly to an intracellular microreactor. Furthermore, it has been shown that 5 nm PtNZ activity could be switched off by biomolecular corona formation (protein adsorption) in complex biofluids such as serum. However, once the PtNZs get internalized in the cell, they will end up in lysosomes, where the proteolytic enzymes can digest the protein corona, making the surface atoms (the nanozyme's active sites) available, thus reactivating the PtNZs.78 Notably, the PtNZ activity is higher at physiological temperature (37 °C) compared to room temperature and is boosted at lysosomal pH (4.5–5) compared to the extracellular pH (7.4, i.e., in the bloodstream). Recent results showed that acting from the lysosome (and protecting it), PtNZs also protect other organelles, such as the mitochondria, which are an essential source of ROS production, keeping the intracellular ROS level under control and maintaining a healthy/effective mitochondria–lysosome crosstalk.79 The ability of PtNZ to modulate ROS levels can thus translate into the regulation of inflammatory signaling and pathway, as described in the next section.
3.3 Potential anti-inflammatory mechanisms/actions of PtNZs
The in vitro anti-inflammatory effects of PtNZs have been overlooked, and details regarding their mechanism of action during inflammation are still mostly unknown. Nevertheless, a study on the effect of PtNZs in lipopolysaccharide (LPS)-stimulated macrophages (RAW 264.7 cells) investigated some of the mechanistic aspects showing significantly reduced LPS-induced production of inflammatory mediators other than reduced intracellular levels of ROS.80 Furthermore, PtNPs inhibited the phosphorylation of ERK1/2, Akt, as well as the phosphorylation/degradation of IkB leading to transcriptional activity impairment of nuclear factor kappa B (NFκB). These indications suggest that PtNZs interfere with intracellular pathways converging on pro-inflammatory gene transcription.
In the following we examine the processes of inflammation and the potential key sites of actions of PtNZs. As previously mentioned PtNZs are usually internalized by the endocytosis/micropinocytosis uptake mechanism, moving them to the endolysosomal compartment. Such intracellular organelles can represent the first target (Fig. 3A) for PtNZ anti-inflammatory activity. By quenching the overproduction of ROS in the endolysosome or lipid oxidation in the peroxisomes (Fig. 2A (1)), PtNZs could reduce the possibility to spark inflammation raised by high ROS concentration-induced damage. Pathogen-stimulated endocytosis is mediated by PAMP receptors, which trigger gene transcription through specific proteins (transcription factors) stereotyped by the NFκB family.81 NFκB-induced gene expression generates the production of proinflammatory proteins, such as cytokines,82 chemokines,83 and adhesion molecules,84 as well as a plethora of diverse co-stimulatory molecules.85–87 All these intercellular messengers cooperate to recruit and activate leukocytes at the sites of infection/damage. Furthermore, they modulate tissue physiology to increase the efficacy of leukocyte recruitment by facilitating the enlargement of capillaries and the accumulation of liquids. In fact, together with pain and redness, the swelling indicates the first sign of local inflammation in basic medicine. PtNZs could also interfere with the extracellular compartments decreasing phagocyte activation by scavenging inside or outside the ROS producing cells. PtNZs released in the bloodstream can hardly cross the tight junctions of the endothelium; however, they take advantage of inflammation-induced transient vascular permeability88 and penetrate the tissue reaching the inflammation site (Fig. 3B). PtNZ location at the core of the inflammatory process will increase their catalytic efficacy on extracellular ROS. Exposure to prolonged microbial and/or downstream inflammatory stimuli (i.e., cytokines) may also result in chronic pathological modification of the surrounding tissue. Eventually, epigenetic adaptations of innate immune cells may establish a certain degree of memory that decreases the stimuli-triggered threshold of activation.89 These adapted IIS cells display a faster and stronger response, which does not stop once the infection is erased, leading to chronic responses and uncontrolled production of ROS. The prolonged intracellular localization of PtNZs could beneficially regulate such aberrant ROS synthesis and maintain their levels below a toxic threshold for a long time. It is also worth mentioning that the interaction between phagocytes and extracellular microbes is almost always mediated by the complement system, an ancient enzymatic protein cascade aimed at pathogen opsonization and killing.50,90–92 Other important plasma acute-phase reactants able to bind the surface molecules of bacteria and fungi are pentraxins, a family of multimeric pattern recognition proteins playing several roles in the IIS response.58,59 To the best of our knowledge, specific studies on the interaction between these opsonizing molecules and PtNZs are today missing, although it would be crucial to understand possible adsorption properties for in vivo applications.
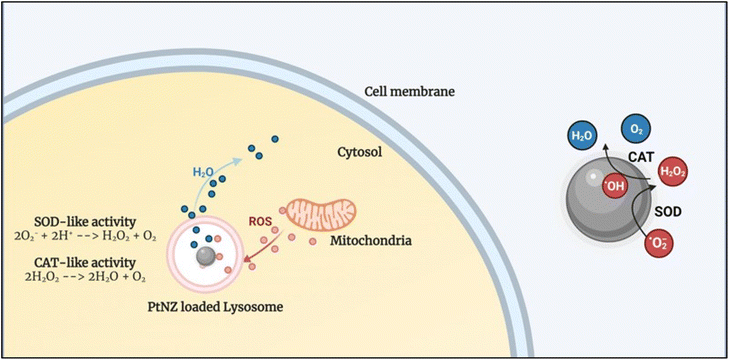 |
| Fig. 3 Schematic showing the intracellular PtNZ CAT-like and SOD-like activity. The PtNZ localized in the lysosome can act as ROS scavengers, preventing ROS from reaching abnormally high levels. | |
In general, most of the ROS produced by mitochondria remain in these intracellular compartments,93 not clearly correlating with the high efficiency results obtained with PtNZs previously described. However, the described physical contact between mitochondria and PtNZ-loaded endolysosomes, shows that the intracellular proximity of ROS and NZs could help to unravel the possible mechanism.79 PtNZs could also directly act within or close to the ER (Fig. 2A (4) and 2A (5)), as a cross-talk between PtNZ-loaded endolysosomes and ER has been reported by the same authors. Further leaking of ROS into the cytosol or the nucleus occurs following the required increased physiological respiration by mitochondria,94 as previously described. In our view, this step of the inflammatory process could be weakened upstream by PtNZs ROS scavenging within endothelial or epithelial cells, the IIS cells preventing their activation (Fig. 2B (1)), and in the extracellular space (Fig. 2B (4)) by reducing the ROS-mediated membrane or protein damage. This crucial endothelium-immune system feed-forward inflammatory step represents the major target for PtNZ-based therapies inhibiting the extravasation process before it occurs. Indeed, the major anti-inflammatory contribution of PtNZ-mediated ROS scavenging could derive from the contemporaneous effect in the extracellular space, where PtNZs are usually delivered, into the endothelium and the IIS cells, where the particles are quickly internalized. Blocking the local self-feeding and uncontrolled inflammation impedes further amplification to systemic reactions. Interpreting the described results, we may also hypothesize that systemically released PtNZs may quench cytokine storms in different diseases contributing to therapeutic reduction of these noxious inflammatory states.
3.4. Anti-inflammatory outcomes of PtNZs in vivo
The evaluation of the anti-inflammatory outcomes has been performed in different tissues after in vivo administration of PtNZs. Hints of significant ROS scavenging and reduction of inflammation have been observed in the skin, lungs, gut and brain following PtNZ administration through different routes, as discussed in detail in the following paragraphs.
3.4.1. Skin.
The sites of inflammation can be reached by different administration routes. The outermost body organ that can be inflamed is the skin. Infected or damaged keratinocytes start and regulate skin inflammation by direct interaction with immune cells and cytokine production.95 Poly-acrylate-PtNPs (PAA-PtNPs) dispersed in gel formulation demonstrated significant protection against ultraviolet B (UVB) and UVC-light exposed skin inflammation by decreasing ROS production and keratinocyte apoptosis in mice.96 Although decreased metabolism and activation of caspase 3, 7, and 9 have been documented using 6 nm but not 60 nm PVP-PtNPs on primary keratinocytes,97 no cell death was observed, nor cell migration performances were affected by both particles. It is also worth mentioning the results obtained with PAPLAL, a mixture of Pd and Pt nanoparticles that has been used in Japan since decades to treat chronic diseases.98 Aging-related skin-thinning associated with lipid peroxidation was suppressed in vitro and, in particular, in vivo by transdermal PAPLAL treatment in Sod1 knockout (KO) mice. PtNPs exhibited marked SOD and CAT activity, whereas the presence of PdNPs in the mixture seemed important to prevent the oxidative deterioration of PtNPs in air. The release of pro-inflammatory cytokines was also observed in the skin of Sod1 −/− mice. Downregulation of inflammation was confirmed later in human keratinocytes treated with the same mixture to inhibit stress-induced oxidative disease pathways by activating antioxidant machinery related to Sod1 expression.99
3.4.2. Lungs.
Beneficial roles of ROS-decreasing PtNZs are emerging for medical treatments in cancer therapy.100 Direct involvement of ROS-mediated regulation of the aggressive invasion by cancer cells has been demonstrated in human lung carcinoma H460 cells.101 In particular, hydroxyl radical up-regulated the caveolin 1 (Cav-1) expression promoting cell migration. This is a clear example of PtNZ-mediated impairment of tissue invasion and cell migration involving ROS-mediated cell adhesion molecules’ dysregulation. Furthermore, PtNZs inhibit the secondary effects due to inflammation-promoted production of ROS that leads to chronic diseases including pulmonary fibrosis and lung cancer.102,103 Actually, the line fluid covering the respiratory epithelium contains several natural antioxidants to protect the wide tissue surface area from the inhaled pathogens, smoke and microparticle-induced insults. Remarkably, intranasal administration of very small (2 nm) PAA-PtNP inhibited pulmonary inflammation in mice exposed to cigarette smoke.21 The administration of PtNZs alone did not affect cell viability nor elicited pulmonary inflammation. Instead, PtNZ treatment impaired smoke-induce neutrophil activation and NFκB-mediated transcription in primed lungs of mice, demonstrating the contemporaneous action in the IIS and the different involved tissues.
3.4.3. Gut.
Albeit platinum anti-inflammatory effects have been described for decades in Japan, and colloidal-Pt is commonly used in anti-bacterial and anti-inflammatory commercial drinkable suspensions, a few studies have been performed to deeply understand the effects of suspensions of sole PtNPs on the gut.20,75,104 We already mentioned the pro-inflammatory results obtained in vivo by intrathecal release of PtNPs.75 In contrast, more recent experiments20 showed anti-inflammatory outcomes of citrate-PtNPs in a dextran sodium sulfate (DSS)-induced colitis model in mice. Protective mechanisms of 5, 30 and 70 nm size PtNPs against DSS-induced intestinal epithelial barrier leakage were observed in a size dependent manner. PtNPs stimulated expressions of colonic tight junction proteins and the heat-shock-protein 25 (Hsp25) enhancing the gut-barrier function. The chaperone role of heat-shock proteins is worth mentioning to maintain a suitable conformation in ROS-mediated protein-unfolding, which we previously described as an important event triggering inflammation. Although the anti-inflammatory effect in the inflamed gut was mainly due to the PtNZ internalization into the epithelium, the contribution of the PtNZs to quench the immune response amplification may also be important. In fact, Zhu and colleagues proved the impairment of colitis-induced high TNF-α and IL-6 levels in the colon as well as in serum, reporting the reduction of inflammation in the gut tissue and systemically. Besides, the authors observed significant attenuation of TLR4-mediated NFκB activation and of the following ROS-induced pro-inflammatory cytokine release, in murine monocyte/macrophage RAW264.7 cells stimulated with LPS. They confirmed the impairment of inflammatory pathways by internalized PtNPs in immune cells playing a central role in systemic inflammation (see the following). Tunçer et al. abrogated H2O2-induced oxidative stress related cytotoxicity in human Caco-2 colorectal adenocarcinoma cells using colloidal 7–8 nm PtNPs.104 The therapeutic effects of PVP-PtNPs below 5 nm size integrated within biocompatible metal–organic frameworks (MOFs) with an Mn-porphyrin [5,10,15,20-tetrakis(4-carboxyphenyl)-porphyrinato]-Mn(III) chloride (TCPP-Mn, mimicking the active site of Mn SOD), named Pt@PCN222-Mn105 have been proved in two different murine models of bowel inflammatory diseases in vivo. ˙O2− dismutation into H2O2 by SOD and the CAT-mediated catalysis in H2O and O2 are naturally occurring in different intracellular compartments, namely, dismutation in the cytosol and mitochondria and catalysis in the peroxisome/lysosome. The authors have designed a nano-complex named Pt@PCN222-Mn to allow for SOD and CAT cascade reactions to occur in the same compartment, so increasing ˙O2− scavenging efficiency. It is interesting to note that PtNZs alone were more efficient in SOD and CAT activity in vitro, as reported in the results as positive controls suggesting a beneficial CAT-like role of PtNZs in gut cell damage and inflammation.
3.4.4. Brain.
Significant improvement of the damaged brain after ischemic stroke was firstly demonstrated by administrating 2–3 nm PtNPs in C57/Bl6 mice following transient middle cerebral artery occlusion (tMCAO).106 Two aspects of this work highlight PtNZ therapeutic potential, namely their localization into the brain by release in the bloodstream and the inhibition of matrix metalloproteinase 9 (MMP-9). MMP-9 is regulated by ROS and considered a marker of acute inflammation and a key mediator of the blood–brain barrier (BBB) disruption.107 4.0 μmol kg−1 PtNPs resuspended in physiological saline solution were injected into the tail vein of mice and reached the infarcted brain cortex facilitated by local damage of the BBB. This in vivo route of delivery proves the remarkable capacity of the particles to be considered for future therapies. The authors reasonably presumed that PtNZ-mediated scavenging of superoxide anions led to the reduction of MMP-9 and collagen IV degradation in the cerebral cortex, correlating with the significant recovery of the motor function of tMCAO-treated mice in behavioral tests. Amelioration of the motor cortex inflammation and function was confirmed by the same authors in subsequent experiments using thrombolytic agent tissue plasminogen activator (tPA) to worsen cerebral infarct-dependent motor functions.108 These data together with the above-cited results of rat organotypic hippocampal cultures under physiological or excitotoxic conditions72 indicate PtNZs efficacy for neuro-inflammatory diseases. Indeed, anti-inflammatory effects on microglia have been observed using PtNZs combined with other metals in neuroinflammatory models.109 Although acidosis is a hallmark of inflammation and IIS phagocyte activation,3 prolonged and repeated exposure to some inflammatory stimuli may restore neutral pH, as IIS cells become tolerant to those stimuli, downregulating pro-inflammatory cytokine production. Clear evidence of this phenomenon has been described for microglia in Alzheimer's disease's plaques, and LPS repeated stimulation.110,111 This condition leading to chronic inflammation and ROS production would require SOD and CAT activity at neutral pH. Efficient trimetallic NZs (triM-NZs) composed of Pt, Pd, and molybdenum (Mo) were synthesized to restore ROS, as well as reactive nitrogen species (RNS) in the LPS-induced brain inflammation model.109 Both ROS and RNS are present in inflammation-induced brain injury. PtPdMo-NZ demonstrated high catalytic selectivity and activity at neutral pH overcoming the enzymatic performances of the single-noble metal NZs. Mo doping of PtPd catalytic particles in a Pt
:
Pd
:
Mo ratio of approximately 6
:
9
:
1 induces lattice deformation and exposure of more active sites that increase the efficiency of trim-NZs. The hollow cubic shape of roughly 13 nm with uneven internal reactive surfaces highly increases the number of active sites. Like other PtNZs, PVP conjugation of the trim-NZ surfaces improves stability in the biological environment allowing ROS scavenging in the described in vitro brain inflammation model. All these pieces of evidence show the possible translational applications of PtNZs to different neuroinflammatory pathologies.
4. Toward PtNZ anti-inflammatory therapeutics: hypothesis and perspectives
Concerning the geometrical features, as per other nanomaterials that should be exploited for their enzyme-like activity in the biological environment, PtNPs presenting small and ultrasmall sizes (i.e., core size of about 2–10 nm) or assembly of those opening up112 in the biological target might be a better option since a high surface/volume ratio allows maximizing the catalytic performances per material weight concentration while keeping energy-dependent cell internalization processes (necessary for the precision medicine approach). PtNPs with a certain shape, such as porous or spiky ones, exhibit a higher surface/volume ratio than the corresponding compact spherical NPs, and combined with potential shape-dependent PtNP uptake might present unique properties that should be explored in this field.71,113,114 If aiming for future clinical translation, it is essential to produce highly controlled PtNP shapes and objectively categorize them in order to analyze and link their geometrical features (especially for complex shapes) with their catalytic and biological properties.115 Shape metrology methods have been recently reported, and it has been shown that even little differences in the nanostructure could elicit significantly different biological identity and immune responses. Certainly, the colloidal stability of PtNZs in the biological media of interest should be considered as a critical aspect since it affects size/shape, surface atom availability, cellular uptake, and intracellular nanozyme efficacy.71
The biomolecular corona, spontaneously forms once the nanomaterials come in contact with biological fluids, this should be avoided or exploited depending on the target within the inflamed tissue. An artificial protein corona (controlled biological identity) containing targeting moieties such as specific antibodies or other biomolecules recognizable by the cells target involved in the inflammation process could be an asset, providing enhanced PtNZ internalization and consequent corona degradation and catalytic boost.
It must be stressed that, while in the context of this study the concepts of nanoparticle's biological identity and biological recognition have been concisely discussed for practical reasons, they involve quite sophisticated nano-bio interplay mechanisms, with strict requirements and many complex characterization methodologies to be properly investigated and understood, as recently summarized elsewhere.116 For example, other than one protein in the biomolecular corona mediating the receptor-mediated uptake, it is essential that key epitopes are exposed and available for the interaction (recognition), which translates into the fact that the orientation of the protein and thus a certain control over the biomolecular corona organization rather than bare composition would be needed. All this is challenging but would unlock the expected unique biological control of nanomaterials.
Nevertheless, in some cases, avoiding biomolecular corona and lysosomal accumulation might be useful to target other organelles potentially responsible for pathological ROS concentration production due to genetic/metabolic disorders such as mitochondria and ER (see Fig. 2A). Ultrasmall PtNPs might be a better option in this case. If properly functionalized, these NPs can nearly eliminate the formation of a long-term biomolecular corona and improve off-cell targeting.107,108 However, enzyme-like activity of ultrasmall PtNPs in complex media, such as blood or other biomolecule-rich interstitial fluids, still needs to be investigated. It must be considered that covalently bound molecular targeting ligands could hamper the PtNPs activity by surface atoms sequestration.
It is currently hard to predict whether there is a generally preferable cell targeting strategy for PtNZ anti-inflammatory applications; this aspect will probably depend on the biological tissue we aim to protect or the triggering factors behind chronic inflammation. In some cases, it might be convenient to target epithelial cells known to be exposed to certain risk factors (i.e., light irradiation), limiting immune cell recruitment, while in others it might be more effective to target the innate immune cells directly to keep a para-inflammation state (low activation level).
Neurodegenerative diseases, for instance, are often characterized by aberrant ROS concentration and chronic inflammation. Activated microglia (innate immune cells in the brain) are considered an important therapeutic target, since they play a key role in maintaining homeostasis between oxidative and antioxidant species.117 Microglia are sensitive to variations in ROS concentrations and activate the secretion of a large amount of pro-inflammatory factors, which can strongly intensify the inflammation state leading to neuronal death. Targeting microglia PtNZ could be employed as an anti-inflammatory agent for the treatment of a broad spectrum of neurodegenerative diseases (Parkinson's, Alzheimer's, etc.…). This aspect has been recently investigated employing other metallic NZs, and the potential of PtNZs should be definitely studied in this context.118 For example, linking selected immune mediators to PtNZ, such as chemokines could allow their specific delivery and the recognition by leukocyte subsets, as monocytes/macrophages.119–121 Nevertheless, extra care must be taken when targeting immune cells since stimulation and suppression of the immune reaction are related to fine regulation of the signaling units. Avoiding uncontrolled endotoxins contaminations (lipopolysaccharide can strongly adsorb onto the NP surface) will be of primary importance.114,122 The choice of the cellular target for anti-inflammatory therapy will depend on the specific inflammatory conditions (disease, infection, and exposure to environmental factors) and the underlying mechanisms involved. Adaptive immune system cells such as T cells, which are critical mediators of the immune response, including inflammation, should also be considered a potential target. For example, in chronic autoimmune inflammatory diseases, such as rheumatoid arthritis,123 mutated short-lived tissue-invasive T-lymphocytes play a key role in maintaining inflammation and their action could be quenched by PtNZs.
Despite some differences, analogous strategies can be adopted in the case of chronic inflammation induced by pathogen infections. As we reported, the main defense mechanism to counteract bacterial infections relies on the endocytosis of opsonized microorganisms and their ROS-mediated destruction in the lysosomes. Paradoxically, some pathogenic bacteria lack catalase and produce high levels of ROS inhibiting the host pro-inflammatory triggering process, and then facilitating tissue colonization by bacteria.124 In particular, strains like Streptococcus pneumoniae or Streptococcus oralis produce hydrogen peroxide (H2O2) through pyruvate oxidase (SpxB)-mediated oxidation of pyruvate to form acetyl phosphate, CO2 and H2O2.125 A high concentration of H2O2 within phagocytes inhibits inflammasome assembly, an enzymatic complex that allows the formation of IL1 cytokine family through caspase-1 mediated proteolysis of IL1 precursors,126 reducing the pro-inflammatory mechanisms necessary to kill the bacteria. From this perspective, PtNZ-mediated scavenging could reduce the production of peroxide radicals restoring an efficient immune response acting as an inflammatory regulator. Tailored opsonization of the particle could facilitate PtNZ lysosomal colocalization with the bacteria. For instance, PtNZs could be previously incubated with a calibrated concentration of the same glycoproteins exposed to the bacterial wall and released in the infection site. PtNZ-adsorbed bacterial molecules will enhance the recognition by TLRs expressed on the phagocytes and their endocytosis. As mentioned above, the formation of a corona is a well-known event following NP dispersion in a biological fluid.127 The adsorbed biomolecules can dictate tissue localization and cellular uptake of the particles through receptor-mediated internalization.128 Covering the surface with proteins can be considered as a general method to functionalize NPs for receptor recognition.129 In particular, PtNZ adsorption of selected immune mediators, such as chemokines,130 could allow their specific delivery and recognition by leukocyte subsets, as monocytes/macrophages in the case of bacterial infection.
Several strategies can be planned to implement PtNZ modulation of inflammatory responses. Albeit many studies have shown their ROS scavenging activity in diverse tissues, the administration routes, NP dose, shape and surface functionalization could improve their efficiency to act at the different stages of local and systemic inflammation. These improvements will allow the therapeutic use of PtNZs to reduce inflammation in several infections and autoimmune diseases.
Conflicts of interest
There are no conflicts to declare.
References
- R. Medzhitov, Origin and physiological roles of inflammation, Nature, 2008, 454, 428–435, DOI:10.1038/NATURE07201.
- F. A. Bonilla and H. C. Oettgen, Adaptive immunity, J. Allergy Clin. Immunol., 2010, 125(2), S33–S40, DOI:10.1016/J.JACI.2009.09.017.
- K. Rajamäki, T. Nordström, K. Nurmi, K. E. O. Åkerman, P. T. Kovanen, K. Öörni and K. K. Eklund, Extracellular acidosis is a novel danger signal alerting innate immunity via the NLRP3 inflammasome, J. Biol. Chem., 2013, 288, 13410–13419, DOI:10.1074/JBC.M112.426254.
- R. Scrivo, M. Vasile, I. Bartosiewicz and G. Valesini, Inflammation as “common soil” of the multifactorial diseases, Autoimmun. Rev., 2011, 10, 369–374, DOI:10.1016/j.autrev.2010.12.006.
- H. Sies and D. P. Jones, Reactive oxygen species (ROS) as pleiotropic physiological signalling agents, Nat. Rev. Mol. Cell Biol., 2020, 21, 363–383, DOI:10.1038/S41580-020-0230-3.
- D. Fokam and D. Hoskin, Instrumental role for reactive oxygen species in the inflammatory response, Front. Biosci., Landmark Ed., 2020, 25, 1110–1119, DOI:10.2741/4848.
- A. Dandekar, R. Mendez and K. Zhang, Cross talk between ER stress, oxidative stress, and inflammation in health and disease, Methods Mol. Biol., 2015, 1292, 205–214, DOI:10.1007/978-1-4939-2522-3_15.
- S. Bindu, S. Mazumder and U. Bandyopadhyay, Non-steroidal anti-inflammatory drugs (NSAIDs) and organ damage: A current perspective, Biochem. Pharmacol., 2020, 180, 114147, DOI:10.1016/J.BCP.2020.114147.
- N. K. Panchal and E. Prince Sabina, Non-steroidal, anti-inflammatory drugs (NSAIDs): A current insight into its molecular mechanism eliciting organ toxicities, Food Chem. Toxicol., 2023, 172, 113598, DOI:10.1016/J.FCT.2022.113598.
- C. Dong, X. Ma, Y. Huang, Y. Zhang and X. Gao, Carbon dots nanozyme for anti-inflammatory therapy via scavenging intracellular reactive oxygen species, Front. Bioeng. Biotechnol., 2022, 10, 1439, DOI:10.3389/FBIOE.2022.943399.
- B. C. Nelson, M. E. Johnson, M. L. Walker, K. R. Riley and C. M. Sims, Antioxidant Cerium Oxide Nanoparticles in Biology and Medicine, Antioxidants, 2016, 5(2), 15, DOI:10.3390/ANTIOX5020015.
- X. Ge, Z. Cao and L. Chu, The Antioxidant Effect of the Metal and Metal-Oxide Nanoparticles, Antioxidants, 2022, 11(4), 791, DOI:10.3390/ANTIOX11040791.
- Q. Liu, A. Zhang, R. Wang, Q. Zhang and D. Cui, A Review on Metal- and Metal Oxide-Based Nanozymes: Properties, Mechanisms, and Applications, Nano-Micro Lett., 2021, 13, 154, DOI:10.1007/S40820-021-00674-8.
- J. Lou-Franco, B. Das, C. Elliott and C. Cao, Gold Nanozymes: From Concept to Biomedical Applications, Nano-Micro Lett., 2020, 13, 10, DOI:10.1007/S40820-020-00532-Z.
- A. Abed, M. Derakhshan, M. Karimi, M. Shirazinia, M. Mahjoubin-Tehran, M. Homayonfal, M. R. Hamblin, S. A. Mirzaei, H. Soleimanpour, S. Dehghani, F. F. Dehkordi and H. Mirzaei, Platinum Nanoparticles in Biomedicine: Preparation, Anti-Cancer Activity, and Drug Delivery Vehicles, Front. Pharmacol., 2022, 13, 797804, DOI:10.3389/FPHAR.2022.797804.
- D. Pedone, M. Moglianetti, E. De Luca, G. Bardi and P. P. Pompa, Platinum nanoparticles in nanobiomedicine, Chem. Soc. Rev., 2017, 46, 4951–4975, 10.1039/C7CS00152E.
- Z. Yu, R. Lou, W. Pan, N. Li and B. Tang, Nanoenzymes in disease diagnosis and therapy, Chem. Commun., 2020, 56, 15513–15524, 10.1039/D0CC05427E.
- B. Yang, Y. Chen and J. Shi, Reactive
Oxygen Species (ROS)-Based Nanomedicine, Chem. Rev., 2019, 119, 4881–4985, DOI:10.1021/ACS.CHEMREV.8B00626.
- Y. Lu, C. Cao, X. Pan, Y. Liu and D. Cui, Structure design mechanisms and inflammatory disease applications of nanozymes, Nanoscale, 2022, 15, 14–40, 10.1039/D2NR05276H.
- S. Zhu, M. Zeng, G. Feng and H. Wu, Platinum Nanoparticles As A Therapeutic Agent Against Dextran Sodium Sulfate-Induced Colitis In Mice, Int. J. Nanomed., 2019, 14, 8361–8378, DOI:10.2147/IJN.S210655.
- S. Onizawa, K. Aoshiba, M. Kajita, Y. Miyamoto and A. Nagai, Platinum nanoparticle antioxidants inhibit pulmonary inflammation in mice exposed to cigarette smoke, Pulm. Pharmacol. Ther., 2009, 22, 340–349, DOI:10.1016/J.PUPT.2008.12.015.
- L. Sun, X. Wang, J. Saredy, Z. Yuan, X. Yang and H. Wang, Innate-adaptive immunity interplay and redox regulation in immune response, Redox Biol., 2020, 37, 101759, DOI:10.1016/J.REDOX.2020.101759.
- H. Sies, Hydrogen peroxide as a central redox signaling molecule in physiological oxidative stress: Oxidative eustress, Redox Biol., 2017, 11, 613–619, DOI:10.1016/j.redox.2016.12.035.
- D. C. Thomas, How the phagocyte NADPH oxidase regulates innate immunity, Free Radicals Biol. Med., 2018, 125, 44–52, DOI:10.1016/j.freeradbiomed.2018.06.011.
- K. Brieger, S. Schiavone, F. J. Miller and K. H. Krause, Reactive oxygen species: from health to disease, Swiss Med. Wkly., 2012, 142(3334), w13659, DOI:10.4414/SMW.2012.13659.
- A. Fernández-Sánchez, E. Madrigal-Santillán, M. Bautista, J. Esquivel-Soto, Á. Morales-González, C. Esquivel-Chirino, I. Durante-Montiel, G. Sánchez-Rivera, C. Valadez-Vega and J. A. Morales-González, Inflammation, oxidative stress, and obesity, Int. J. Mol. Sci., 2011, 12, 3117–3132, DOI:10.3390/IJMS12053117.
- D. E. Handy and J. Loscalzo, Redox regulation of mitochondrial function, Antioxid. Redox Signaling, 2012, 16, 1323–1367, DOI:10.1089/ARS.2011.4123.
- D. S. Schwarz and M. D. Blower, The endoplasmic reticulum: structure, function and response to cellular signaling, Cell. Mol. Life Sci., 2016, 73(1), 79–94, DOI:10.1007/s00018-015-2052-6.
- B. P. Tu and J. S. Weissman, Oxidative protein folding in eukaryotes: mechanisms and consequences, J. Cell Biol., 2004, 164, 341–346, DOI:10.1083/JCB.200311055.
- B. P. Tu and J. S. Weissman, The FAD- and O(2)-dependent reaction cycle of Ero1-mediated oxidative protein folding in the endoplasmic reticulum, Mol. Cell, 2002, 10, 983–994, DOI:10.1016/S1097-2765(02)00696-2.
- A. R. Frand, J. W. Cuozzo and C. A. Kaiser, Pathways for protein disulphide bond formation, Trends Cell Biol., 2000, 10, 203–210, DOI:10.1016/S0962-8924(00)01745-1.
- J. W. Cuozzo and C. A. Kaiser, Competition between glutathione and protein thiols for disulphide-bond formation, Nat. Cell Biol., 1999, 1, 130–135, DOI:10.1038/11047.
- K. Zhang and R. J. Kaufman, From endoplasmic-reticulum stress to the inflammatory response, Nature, 2008, 454(7203), 455–462, DOI:10.1038/nature07203.
- M. Pajares, N. Jiménez-Moreno, I. H. K. Dias, B. Debelec, M. Vucetic, K. E. Fladmark, H. Basaga, S. Ribaric, I. Milisav and A. Cuadrado, Redox control of protein degradation, Redox Biol., 2015, 6, 409–420, DOI:10.1016/J.REDOX.2015.07.003.
- S. Pesakhov, R. Benisty, N. Sikron, Z. Cohen, P. Gomelsky, I. Khozin-Goldberg, R. Dagan and N. Porat, Effect of hydrogen peroxide production and the Fenton reaction on membrane composition of Streptococcus pneumoniae, Biochim. Biophys. Acta, 2007, 1768, 590–597, DOI:10.1016/J.BBAMEM.2006.12.016.
- J. Zindel and P. Kubes, DAMPs, PAMPs, and LAMPs in Immunity and Sterile Inflammation, Annu. Rev. Pathol., 2020, 15, 493–518, DOI:10.1146/annurev-pathmechdis-012419-032847.
- G. L. Burn, A. Foti, G. Marsman, D. F. Patel and A. Zychlinsky, The Neutrophil., Immunity, 2021, 54, 1377–1391, DOI:10.1016/J.IMMUNI.2021.06.006.
- Y. Oishi and I. Manabe, Macrophages in inflammation, repair and regeneration, Int. Immunol., 2018, 30, 511–528, DOI:10.1093/INTIMM/DXY054.
- S. Balan, M. Saxena and N. Bhardwaj, Dendritic cell subsets and locations, Int. Rev. Cell Mol. Biol., 2019, 348, 1–68, DOI:10.1016/BS.IRCMB.2019.07.004.
- M. Herb and M. Schramm, Functions of ROS in Macrophages and Antimicrobial Immunity, Antioxidants, 2021, 10(2), 313, DOI:10.3390/antiox10020313.
- E. Vivier, E. Tomasello, M. Baratin, T. Walzer and S. Ugolini, Functions of natural killer cells, Nat. Immunol., 2008, 9, 503–510, DOI:10.1038/NI1582.
- B. Zitti and Y. T. Bryceson, Natural killer cells in inflammation and autoimmunity, Cytokine Growth Factor Rev., 2018, 42, 37–46, DOI:10.1016/J.CYTOGFR.2018.08.001.
- T. Kawai and S. Akira, Toll-like receptors and their crosstalk with other innate receptors in infection and immunity, Immunity, 2011, 34, 637–650, DOI:10.1016/J.IMMUNI.2011.05.006.
- K. H. Lim and L. M. Staudt, Toll-like receptor signaling, Cold Spring Harbor Perspect. Biol., 2013, 5(1), a011247, DOI:10.1101/CSHPERSPECT.A011247.
- C.-S. Yang, D.-M. Shin, K.-H. Kim, Z.-W. Lee, C.-H. Lee, S. G. Park, Y. S. Bae and E.-K. Jo, NADPH Oxidase 2 Interaction with TLR2 Is Required for Efficient Innate Immune Responses to Mycobacteria via Cathelicidin Expression, J. Immunol., 2009, 182, 3696–3705, DOI:10.4049/JIMMUNOL.0802217.
- H. S. Park, J. N. Chun, H. Y. Jung, C. Choi and Y. S. Bae, Role of NADPH oxidase 4 in lipopolysaccharide-induced proinflammatory responses by human aortic endothelial cells, Cardiovasc. Res., 2006, 72(3), 447–455, DOI:10.1016/j.cardiores.2006.09.012.
- H. S. Park, H. Y. Jung, E. Y. Park, J. Kim, W. J. Lee and Y. S. Bae, Cutting Edge: Direct Interaction of TLR4 with NAD(P)H Oxidase 4 Isozyme Is Essential for Lipopolysaccharide-Induced Production of Reactive Oxygen Species and Activation of NF-κB, J. Immunol., 2004, 173, 3589–3593, DOI:10.4049/JIMMUNOL.173.6.3589.
- J. M. Platnich and D. A. Muruve, NOD-like receptors and inflammasomes: A review of their canonical and non-canonical signaling pathways, Arch. Biochem. Biophys., 2019, 670, 4–14, DOI:10.1016/J.ABB.2019.02.008.
- N. Kelley, D. Jeltema, Y. Duan and Y. He, The NLRP3 Inflammasome: An Overview of Mechanisms of Activation and Regulation, Int. J. Mol. Sci., 2019, 20(13), 3328, DOI:10.3390/IJMS20133328.
-
A. K. Abbas, A. H. Lichtman and S. Pillai, Cellular and Molecular Immunology, Elsevier, 10th edn, 2021, ISBN 9780323757485 Search PubMed.
- N. Parameswaran and S. Patial, Tumor necrosis factor-α signaling in macrophages, Crit. Rev. Eukaryotic Gene Expression, 2010, 20(2), 87–103, DOI:10.1615/critreveukargeneexpr.v20.i2.10.
- M. P. Bevilacqua, Endothelial-leukocyte adhesion molecules, Annu. Rev. Immunol., 1993, 11, 767–804, DOI:10.1146/annurev.iy.11.040193.004003.
- K. Ley, The role of selectins in inflammation and disease, Trends Mol. Med., 2003, 9, 263–268, DOI:10.1016/S1471-4914(03)00071-6.
- C. G. Gahmberg, L. Valmu, S. Fagerholm, P. Kotovuori, E. Ihanus, L. Tian and T. Pessa-Morikawa, Leukocyte integrins and inflammation, Cell. Mol. Life Sci., 1998, 54, 549–555, DOI:10.1007/S000180050183.
- J. Middleton, A. M. Patterson, L. Gardner, C. Schmutz and B. A. Ashton, Leukocyte extravasation: chemokine transport and presentation by the endothelium, Blood, 2002, 100, 3853–3860, DOI:10.1182/BLOOD.V100.12.3853.
- S. Kokura, R. E. Wolf, T. Yoshikawa, D. N. Granger and T. Y. Aw, Molecular mechanisms of neutrophil-endothelial cell adhesion induced by redox imbalance, Circ. Res., 1999, 84, 516–524, DOI:10.1161/01.RES.84.5.516.
- B. Bistrian, Systemic response to inflammation, Nutr. Rev., 2007, 65, 170–172, DOI:10.1301/NR.2007.DEC.S170-S172.
- L. Deban, B. Bottazzi, C. Garlanda, Y. M. De La Torre and A. Mantovani, Pentraxins: Multifunctional proteins at the interface of innate immunity and inflammation, BioFactors, 2009, 35, 138–145, DOI:10.1002/biof.21.
- K. Daigo, A. Inforzato, I. Barajon, C. Garlanda, B. Bottazzi, S. Meri and A. Mantovani, Pentraxins in the activation and regulation of innate immunity, Immunol. Rev., 2016, 274, 202–217, DOI:10.1111/imr.12476.
- B. G. Chousterman, F. K. Swirski and G. F. Weber, Cytokine storm and sepsis disease pathogenesis, Semin. Immunopathol., 2017, 39, 517–528, DOI:10.1007/S00281-017-0639-8.
- B. Hu, S. Huang and L. Yin, The cytokine storm and COVID-19, J. Med. Virol., 2021, 93, 250–256, DOI:10.1002/JMV.26232.
- D. F. Williams, On the mechanisms of biocompatibility, Biomaterials, 2008, 29, 2941–2953, DOI:10.1016/j.biomaterials.2008.04.023.
- M. Jeyaraj, S. Gurunathan, M. Qasim, M.-H. Kang and J.-H. Kim, A Comprehensive Review on the Synthesis, Characterization, and Biomedical Application of Platinum Nanoparticles, Nanomaterials, 2019, 9(12), 1719, DOI:10.3390/nano9121719.
- D. Pedone, M. Moglianetti, E. De Luca, G. Bardi and P. P. Pompa, Platinum nanoparticles in nanobiomedicine, Chem. Soc. Rev., 2017, 46(16), 4951–4975, 10.1039/c7cs00152e.
- F. Gatto and G. Bardi, Metallic nanoparticles: General research approaches to immunological characterization, Nanomaterials, 2018, 8(10), 753, DOI:10.3390/nano8100753.
- M. Moglianetti, E. De Luca, D. Pedone, R. Marotta, T. Catelani, B. Sartori, H. Amenitsch, S. F. Retta and P. P. Pompa, Platinum nanozymes recover cellular ROS homeostasis in an oxidative stress-mediated disease model, Nanoscale, 2016, 8, 3739–3752, 10.1039/C5NR08358C.
- A. Turco, M. Moglianetti, S. Corvaglia, S. Rella, T. Catelani, R. Marotta, C. Malitesta and P. P. Pompa, Sputtering-Enabled Intracellular X-ray Photoelectron Spectroscopy: A Versatile Method To Analyze the Biological Fate of Metal Nanoparticles, ACS Nano, 2018, 12, 7731–7740, DOI:10.1021/ACSNANO.8B01612.
- F. Gatto, R. Cagliani, T. Catelani, D. Guarnieri, M. Moglianetti, P. P. Pompa and G. Bardi, PMA-induced THP-1 macrophage differentiation is not impaired by citrate-coated platinum nanoparticles, Nanomaterials, 2017, 7(10), 332, DOI:10.3390/nano7100332.
- F. Gatto, M. Moglianetti, P. Pompa, G. Bardi, F. Gatto, M. Moglianetti, P. P. Pompa and G. Bardi, Platinum Nanoparticles Decrease Reactive Oxygen Species and Modulate Gene Expression without Alteration of Immune Responses in THP-1 Monocytes, Nanomaterials, 2018, 8, 392, DOI:10.3390/nano8060392.
- S. Gurunathan, M. Jeyaraj, H. La, H. Yoo, Y. Choi, J. T. Do, C. Park, J. H. Kim and K. Hong, Anisotropic Platinum Nanoparticle-Induced Cytotoxicity, Apoptosis, Inflammatory Response, and Transcriptomic and Molecular Pathways in Human Acute Monocytic Leukemia Cells, Int. J. Mol. Sci., 2020, 21(2), 440, DOI:10.3390/ijms21020440.
- W. Zhang, H. Lopez, L. Boselli, P. Bigini, A. Perez-Potti, Z. Xie, V. Castagnola, Q. Cai, C. P. Silveira, J. M. De Araujo, L. Talamini, N. Panini, G. Ristagno, M. B. Violatto, S. Devineau, M. P. Monopoli, M. Salmona, V. A. Giannone, S. Lara, K. A. Dawson and Y. Yan, A Nanoscale Shape-Discovery Framework Supporting Systematic Investigations of Shape-Dependent Biological Effects and Immunomodulation, ACS Nano, 2022, 16, 1547–1559, DOI:10.1021/acsnano.1c10074.
- M. Gulino, S. D. Santos and A. P. Pêgo, Biocompatibility of Platinum Nanoparticles in Brain ex vivo Models in Physiological and Pathological Conditions, Front. Neurosci., 2021, 15, 787518, DOI:10.3389/fnins.2021.787518.
- Z. Elmazoglu, H. Kayhan, A. Santamaría, E. Rangel-López, P. K. Uğur, A. Ceylan, M. Aschner and Ç. Karasu, Platinum nanoparticles Protect Against Lipopolysaccharide-Induced Inflammation in Microglial BV-2 Cells via Decreased Oxidative Damage and Increased Phagocytosis, Neurochem. Res., 2021, 46, 3325–3341, DOI:10.1007/S11064-021-03434-7.
- M. Vanharen, I. Durocher, A. Saafane and D. Girard, Evaluating the Apoptotic Cell Death Modulatory Activity of Nanoparticles in Men and Women Neutrophils and Eosinophils, Inflammation, 2022, 45, 387–398, DOI:10.1007/S10753-021-01553-5.
- E. J. Park, H. Kim, Y. Kim and K. Park, Intratracheal instillation of platinum nanoparticles may induce inflammatory responses in mice, Arch. Pharmacal Res., 2010, 33, 727–735, DOI:10.1007/S12272-010-0512-Y.
- K. Bloch, K. Pardesi, C. Satriano and S. Ghosh, Bacteriogenic Platinum Nanoparticles for Application in Nanomedicine, Front. Chem., 2021, 9, 624344, DOI:10.3389/fchem.2021.624344.
- D. Xu, L. Wu, H. Yao and L. Zhao, Catalase-Like Nanozymes: Classification, Catalytic Mechanisms, and Their Applications, Small, 2022, 18(37), e2203400, DOI:10.1002/smll.202203400.
- G. Tarricone, V. Castagnola, V. Mastronardi, L. Cursi, D. Debellis, D. Z. Ciobanu, A. Armirotti, F. Benfenati, L. Boselli and P. P. Pompa, Catalytic Bioswitch of Platinum Nanozymes: Mechanistic Insights of Reactive Oxygen Species Scavenging in the Neurovascular Unit, Nano Lett., 2023, 23(10), 4660–4668, DOI:10.1021/acs.nanolett.3c01479.
- V. Migliaccio, N. Blal, M. De Girolamo, V. Mastronardi, F. Catalano, I. Di Gregorio, L. Lionetti, P. P. Pompa and D. Guarnieri, Inter-Organelle Contact Sites Mediate the Intracellular Antioxidant Activity of Platinum Nanozymes: A New Perspective on Cell-Nanoparticle Interaction and Signaling, ACS Appl. Mater. Interfaces, 2023, 15(3), 3882–3893, DOI:10.1021/acsami.2c22375.
- M. U. Rehman, Y. Yoshihisa, Y. Miyamoto and T. Shimizu, The anti-inflammatory effects of platinum nanoparticles on the lipopolysaccharide-induced inflammatory response in RAW 264.7 macrophages, Inflammation Res., 2012, 61, 1177–1185, DOI:10.1007/s00011-012-0512-0.
- S. Mitchell, J. Vargas and A. Hoffmann, Signaling via the NFκB system, Wiley Interdiscip. Rev.: Syst. Biol. Med., 2016, 8, 227–241, DOI:10.1002/WSBM.1331.
- C. A. Feghali and T. M. Wright, Cytokines in acute and chronic inflammation, Front. Biosci., 1997, 2, d12–26, DOI:10.2741/a171.
- P. Proost, A. Wuyts and J. Van Damme, The, role of chemokines in inflammation, Int. J. Clin. Lab. Res., 1996, 26, 211–223, DOI:10.1007/BF02602952.
- C. Weber, L. Fraemohs and E. Dejana, The role of junctional adhesion molecules in vascular inflammation, Nat. Rev. Immunol., 2007, 7, 467–477, DOI:10.1038/NRI2096.
- L. Grosche, I. Knippertz, C. König, D. Royzman, A. B. Wild, E. Zinser, H. Sticht, Y. A. Muller, A. Steinkasserer and M. Lechmann, The CD83 Molecule – An Important Immune Checkpoint, Front. Immunol., 2020, 11, 721, DOI:10.3389/fimmu.2020.00721.
- T. So and N. Ishii, The TNF–TNFR family of co-signal molecules, Adv. Exp. Med. Biol., 2019, 1189, 53–84, DOI:10.1007/978-981-32-9717-3_3.
- A. J. Coyle and J. C. Gutierrez-Ramos, The role of ICOS and other costimulatory molecules in allergy and asthma, Springer Semin. Immunopathol., 2004, 25, 349–359, DOI:10.1007/S00281-003-0154-Y.
- K. E. M. Hellenthal, L. Brabenec and N. M. Wagner, Regulation and Dysregulation of Endothelial Permeability during Systemic Inflammation, Cells, 2022, 11(12), 1935, DOI:10.3390/cells11121935.
- G. Hajishengallis, X. Li, I. Mitroulis and T. Chavakis, Trained Innate Immunity and Its Implications for Mucosal Immunity and Inflammation, Adv. Exp. Med. Biol., 2019, 1197, 11–26, DOI:10.1007/978-3-030-28524-1_2.
- M. Gadjeva, The complement system. Overview, Methods Mol. Biol., 2014, 1100, 1–9 CrossRef CAS PubMed.
- P. Conigliaro, P. Triggianese, E. Ballanti, C. Perricone, R. Perricone and M. S. Chimenti, Complement, infection, and autoimmunity, Curr. Opin. Rheumatol., 2019, 31(5), 532–541, DOI:10.1097/BOR.0000000000000633.
- R. B. Pouw and D. Ricklin, Tipping the balance: intricate roles of the complement system in disease and therapy, Semin. Immunopathol., 2021, 43, 757–771, DOI:10.1007/s00281-021-00892-7.
- M. Mittal, M. R. Siddiqui, K. Tran, S. P. Reddy and A. B. Malik, Reactive Oxygen Species in Inflammation and Tissue Injury, Antioxid. Redox Signal., 2014, 20, 1126, DOI:10.1089/ARS.2012.5149.
- M. P. Murphy, How mitochondria produce reactive oxygen species, Biochem. J., 2009, 417, 1–13, DOI:10.1042/BJ20081386.
- P. Seiringer, S. Eyerich, K. Eyerich, D. Dittlein, A. C. Pilz, E. Scala, J. Ring, H. Behrendt, A. Cavani and C. Traidl-Hoffmann, Keratinocytes Regulate the Threshold of Inflammation by Inhibiting T Cell Effector Functions, Cells, 2021, 10(7), 1606, DOI:10.3390/cells10071606.
- Y. Yoshihisa, A. Honda, Q. L. Zhao, T. Makino, R. Abe, K. Matsui, H. Shimizu, Y. Miyamoto, T. Kondo and T. Shimizu, Protective effects of platinum nanoparticles against UV-light-induced epidermal inflammation, Exp. Dermatol., 2010, 19(11), 1000–1006, DOI:10.1111/j.1600-0625.2010.01128.x.
- P. Konieczny, A. G. Goralczyk, R. Szmyd, L. Skalniak, J. Koziel, F. L. Filon, M. Crosera, A. Cierniak, E. K. Zuba-Surma, J. Borowczyk, E. Laczna, J. Drukala, E. Pyza, D. Semik, O. Woznicka, A. Klein and J. Jura, Effects triggered by platinum nanoparticles on primary keratinocytes, Int. J. Nanomed., 2013, 8, 3963–3975, DOI:10.2147/IJN.S49612.
- S. Shibuya, Y. Ozawa, K. Watanabe, N. Izuo, T. Toda, K. Yokote and T. Shimizu, Palladium and Platinum Nanoparticles Attenuate Aging-Like Skin Atrophy via Antioxidant Activity in Mice, PLoS One, 2014, 9(10), e109288, DOI:10.1371/journal.pone.0109288.
- G. Tsuji, A. Hashimoto-Hachiya, M. Takemura, T. Kanemaru, M. Ichihashi and M. Furue, Palladium and Platinum Nanoparticles Activate AHR and NRF2 in Human Keratinocytes-Implications in Vitiligo Therapy, J. Invest. Dermatol., 2017, 137, 1582–1586, DOI:10.1016/J.JID.2017.02.981.
- X. Wang, X. He, C. Liu, W. Zhao, X. Yuan and R. Li, Progress and perspectives of platinum nanozyme in cancer therapy, Front. Chem., 2022, 10, 1092747, DOI:10.3389/fchem.2022.1092747.
- S. Luanpitpong, S. J. Talbott, Y. Rojanasakul, U. Nimmannit, V. Pongrakhananon, L. Wang and P. Chanvorachote, Regulation of lung cancer cell migration and invasion by reactive oxygen species and caveolin-1, J. Biol. Chem., 2010, 285, 38832–38840, DOI:10.1074/JBC.M110.124958.
- N. Azad, Y. Rojanasakul and V. Vallyathan, Inflammation and lung cancer: Roles of reactive oxygen/nitrogen species, J. Toxicol. Environ. Health, Part B, 2008, 11, 1–15, DOI:10.1080/10937400701436460.
- V. Castranova, Signaling pathways controlling the production of inflammatory mediators in response to crystalline silica exposure: Role of reactive oxygen/nitrogen species, Free Radicals Biol. Med., 2004, 37, 916–925, DOI:10.1016/j.freeradbiomed.2004.05.032.
- S. Tunçer, M. Çolakoğlu, S. Ulusan, G. Ertaş, Ç. Karasu and S. Banerjee, Evaluation of colloidal platinum on cytotoxicity, oxidative stress and barrier permeability across the gut epithelium, Heliyon, 2019, 5(3), e01336, DOI:10.1016/j.heliyon.2019.e01336.
- Y. Liu, Y. Cheng, H. Zhang, M. Zhou, Y. Yu, S. Lin, B. Jiang, X. Zhao, L. Miao, C. W. Wei, Q. Liu, Y. W. Lin, Y. Du, C. J. Butch and H. Wei, Integrated cascade nanozyme catalyzes in vivo ROS scavenging for anti-inflammatory therapy, Sci. Adv., 2020, 6(29), eabb2695, DOI:10.1126/sciadv.abb2695.
- M. Takamiya, Y. Miyamoto, T. Yamashita, K. Deguchi, Y. Ohta, Y. Ikeda, T. Matsuura and K. Abe, Neurological and pathological improvements of cerebral infarction in mice with platinum nanoparticles, J. Neurosci. Res., 2011, 89, 1125–1133, DOI:10.1002/jnr.22622.
- Y. Gasche, J. C. Copin, T. Sugawara, M. Fujimura and P. H. Chan, Matrix metalloproteinase inhibition prevents oxidative stress-associated blood-brain barrier disruption after transient focal cerebral ischemia, J. Cereb. Blood Flow Metab., 2001, 21, 1393–1400, DOI:10.1097/00004647-200112000-00003.
- M. Takamiya, Y. Miyamoto, T. Yamashita, K. Deguchi, Y. Ohta and K. Abe, Strong neuroprotection with a novel platinum nanoparticle against ischemic stroke- and tissue plasminogen activator-related brain damages in mice, Neuroscience, 2012, 221, 47–55, DOI:10.1016/j.neuroscience.2012.06.060.
- X. Mu, J. Wang, Y. Li, F. Xu, W. Long, L. Ouyang, H. Liu, Y. Jing, J. Wang, H. Dai, Q. Liu, Y. Sun, C. Liu and X. D. Zhang, Redox Trimetallic Nanozyme with Neutral Environment Preference for Brain Injury, ACS Nano, 2019, 13(2), 1870–1884, DOI:10.1021/acsnano.8b08045.
- G. J. Harry and A. D. Kraft, Neuroinflammation and Microglia: Considerations and approaches for neurotoxicity assessment, Expert Opin. Drug Metab. Toxicol., 2008, 4, 1265, DOI:10.1517/17425255.4.10.1265.
- M. A. Ajmone-Cat, A. Nicolini and L. Minghetti, Prolonged exposure of microglia to lipopolysaccharide modifies the intracellular signaling pathways and selectively promotes prostaglandin E2 synthesis, J. Neurochem., 2003, 87, 1193–1203, DOI:10.1046/j.1471-4159.2003.02087.x.
- X. Zhang, Y. Liu, S. Gopalakrishnan, L. Castellanos-Garcia, G. Li, M. Malassiné, I. Uddin, R. Huang, D. C. Luther, R. W. Vachet and V. M. Rotello, Intracellular Activation of Bioorthogonal Nanozymes through Endosomal Proteolysis of the Protein Corona, ACS Nano, 2020, 14(4), 4767–4773, DOI:10.1021/acsnano.0c00629.
- J. Wang, H. J. Chen, T. Hang, Y. Yu, G. Liu, G. He, S. Xiao, B. r. Yang, C. Yang, F. Liu, J. Tao, M. X. Wu and X. Xie, Physical activation of innate immunity by spiky particles, Nat. Nanotechnol., 2018, 13(11), 1078–1086, DOI:10.1038/s41565-018-0274-0.
- L. Talamini, M. B. Violatto, Q. Cai, M. P. Monopoli, K. Kantner, Ž Krpetić, A. Perez-Potti, J. Cookman, D. Garry, C. P. Silveira, L. Boselli, B. Pelaz, T. Serchi, S. Cambier, A. C. Gutleb, N. Feliu, Y. Yan, M. Salmona, W. J. Parak, K. A. Dawson and P. Bigini, Influence of Size and Shape on the Anatomical Distribution of Endotoxin-Free Gold Nanoparticles, ACS Nano, 2017, 11(6), 5519–5529, DOI:10.1021/acsnano.7b00497.
- L. Boselli, H. Lopez, W. Zhang, Q. Cai, V. A. Giannone, J. Li, A. Moura, J. M. de Araujo, J. Cookman, V. Castagnola, Y. Yan and K. A. Dawson, Classification and biological identity of complex nano shapes, Commun. Mater., 2020, 1, 1–12, DOI:10.1038/s43246-020-0033-2.
- K. A. Dawson and Y. Yan, Current understanding of biological identity at the nanoscale and future prospects, Nat. Nanotechnol., 2021, 16, 229–242, DOI:10.1038/S41565-021-00860-0.
- Q. Li and B. A. Barres, Microglia and macrophages in brain homeostasis and disease, Nat. Rev. Immunol., 2018, 18(4), 225–242, DOI:10.1038/nri.2017.125.
- H. Liu, Y. Han, T. Wang, H. Zhang, Q. Xu, J. Yuan and Z. Li, Targeting Microglia for Therapy of Parkinson’s Disease by Using Biomimetic Ultrasmall Nanoparticles, J. Am. Chem. Soc., 2020, 142(52), 21730–21742, DOI:10.1021/jacs.0c09390.
- A. Pisani, R. Donno, A. Gennari, G. Cibecchini, F. Catalano, R. Marotta, P. P. Pompa, N. Tirelli and G. Bardi, CXCL12-PLGA/Pluronic Nanoparticle Internalization Abrogates CXCR4-Mediated Cell Migration, Nanomaterials, 2020, 10, 1–19, DOI:10.3390/nano10112304.
- A. Pisani, R. Donno, G. Valenti, P. P. Pompa, N. Tirelli and G. Bardi, Chemokine-Decorated Nanoparticles Target Specific Subpopulations of Primary Blood Mononuclear Leukocytes, Nanomaterials, 2022, 12(20), 3560, DOI:10.3390/nano12203560.
- G. Bardi, Chemokines and nanomaterials: interaction for useful immune-applications, Explor. Immunol., 2022, 2, 637–647, DOI:10.37349/EI.2022.00073.
- Y. Li and D. Boraschi, Endotoxin contamination: A key element in the interpretation of nanosafety studies, Nanomedicine, 2016, 11(3), 269–287, DOI:10.2217/nnm.15.196.
- C. M. Weyand and J. J. Goronzy, The immunology of rheumatoid arthritis, Nat. Immunol., 2021, 22, 10–18, DOI:10.1038/S41590-020-00816-X.
- S. F. Erttmann and N. O. Gekara, Hydrogen peroxide release by bacteria suppresses inflammasome-dependent innate immunity, Nat. Commun., 2019, 10(1), 3493, DOI:10.1038/s41467-019-11169-x.
- C. D. Pericone, S. Park, J. A. Imlay and J. N. Weiser, Factors contributing to hydrogen peroxide resistance in Streptococcus pneumoniae include pyruvate oxidase
(SpxB) and avoidance of the toxic effects of the fenton reaction, J. Bacteriol., 2003, 185(23), 6815–6825, DOI:10.1128/JB.185.23.6815-6825.2003.
- M. Lamkanfi and V. M. Dixit, Mechanisms and functions of inflammasomes, Cell, 2014, 157, 1013–1022, DOI:10.1016/J.CELL.2014.04.007.
- M. Hadjidemetriou and K. Kostarelos, Evolution of the nanoparticle corona, Nat. Nanotechnol., 2017, 12, 288–290, DOI:10.1038/nnano.2017.61.
- V. Francia, K. Yang, S. Deville, C. Reker-Smit, I. Nelissen and A. Salvati, Corona Composition Can Affect the Mechanisms Cells Use to Internalize Nanoparticles, ACS Nano, 2019, 13, 11107–11121, DOI:10.1021/ACSNANO.9B03824.
- R. Cagliani, F. Gatto and G. Bardi, Protein Adsorption: A Feasible Method for Nanoparticle Functionalization?, Materials, 2019, 12(12), 1991, DOI:10.3390/ma12121991.
- G. Bardi, Chemokines and nanomaterials: interaction for useful immune-applications, Explor. Immunol., 2022, 2, 637–647, DOI:10.37349/EI.2022.00073.
|
This journal is © The Royal Society of Chemistry 2023 |