DOI:
10.1039/D2NJ03792K
(Paper)
New J. Chem., 2023,
47, 179-191
Resorcinarene-appended octa-substituted alkyl arms: a new strategy to fabricate supramolecular materials for application in liquid crystals and solar cells†
Received
31st July 2022
, Accepted 16th October 2022
First published on 17th October 2022
Abstract
A new series of lower-rim-functionalized octa-substituted resorcin[4]arene-based supramolecular compounds with variable alkoxy side chains (–OC4H9, –OC6H13, –OC8H17, –OC10H21) has been synthesized by a simple two-step procedure. The proposed method is suitable for preparing lower-rim-functionalized resorcinarene-based compounds on a large scale and it is cost-effective as compared to other reported supramesogens. All four functionalized compounds displayed a columnar hexagonal phase (Colh) over a high mesophase temperature range. The optical texture and thermal behaviour of the target compounds were identified by POM and DSC studies and the molecular organization in the mesogenic state was further confirmed by PXRD. The molecular system based on a resorcinarene core with symmetrical substitution of alkyl arms exhibited columnar type self-assembly organization. All four supramolecules with different side alkyl spacers showed high thermal stability. Compound D4 with a decyloxy side chain was further optimized to implicate as optical window and transport layer in solar cells to replace the commercially available toxic Cd-based compound. Herein, we developed thin films of compound D4 on glass and Indium doped Tin oxide glass substartes followed by subjecting the prepared thin films to the characterization tools to explore optical and electical properties. The current–voltage relation shows ohmic-type behaviour. The optical properties display high transmittance in the far visible and NIR regions for all the developed thin films. The derivative showed optical energy band gaps of 1.61 eV, 4.01 eV and 2.27 eV for as deposited and film anneaeld at 100 °C and 200 °C, respectively and findings warrants its suitability for use as an ecofriendly optical window and transport layer in second and third generation solar cells.
1. Introduction
In recent years, researchers have been attracted to the design of molecules to make liquid crystals owing to the constantly increasing demand for organic electronic and optoelectronic devices in the present digital era.1–3 Nowadays, the fabrication of organic solar cells is in great demand as an alternative source for providing energy.4 The essential parameters for materials to implicate in solar cells include efficient absorption of the incident irradiance, charge carrier generation and collection of the generated charge carriers at the electrodes concerned. Further, Cadmium sulphide with wide band gap is has been employed so far as optical window in second generation based CdTe and CIGS solar cell devices to transmit the maximum level of incident light to the respective absorber layers to the devices.5 The CdS could also be applied in third generation devices like perovskite solar cell devices as tranport layer togeher with the conventional in order to enhance device stability. The use of CdS inorganic materials provides a high band-gap and transmittance with the proper alignment of the conduction band level to the work function of the electrode concerned to reduce parasitic resistances.6 However, the CdS-based material layer displays environmental issues due to its toxicity, being especially dangerous when inhaled as dust particles.7–9 Owing to the carcinogenic properties of Cadmium-based compounds, it is necessary to develop non-toxic or carcinogenic compounds (Cd-free) as an improved and green approach for the fabrication of thin film-based optical window and transport layer for application in solar cells.10 Supramolecular organic compound-based solar cells also possess great potential due to their low-cost fabrication method.11 Their properties of light weight, cheapness, flexibility and solution processability make them strong candidates for researchers working in this field.
Supramolecular chemistry is an intense field of research, because it may lead to practical applications to develop cell-mimicking microparticles, optical chemosensors, self-assembly, nano capsules, columnar liquid crystals, antimicrobials, metal ion extraction, HPLC stationary phases, organic light emitting diodes, photoresists, photovoltaics (OPV), and security tests (explosive sensing), which are the subjects of growing societal demand.12–15 Usually, the phase transition of macrocyclic supramolecular compounds is higher as compared to that of normal conventional organic liquid crystalline compounds. In polymeric-based thermotropic liquid crystals, the transition temperature is higher than its decomposition temperature.16 Resorcin[4]arene has a macrocyclic rigid core with 3D-shaped hydrophobic cavities and is a useful building block for various applications.17 Resorcin[4]arene, in the calixarene macrocyclic family, has a rigid core and four resorcine units with eight hydroxyl groups.18 Further, a number of research groups have functionalized the resorcin[4]arene core with various types of functional group and reported various applications.19–24 Self-assembled resorcinarene-based compounds with columnar type liquid crystalline properties have the ability to undergo phase transitions at higher temperature and the mesophase is stabilized at room temperature. To date, very few reports are available on resorcin[4]arene functionalized liquid crystalline materials with variable flexible alkyl chain but rare on lower rim functionalization. In 2015, Yang et al. discovered columnar hexagonal liquid crystals based on resorcin[4]arene functionalized by cholesterol units.25 Later, same group reported a lamellar type columnar mesophase of resorcin[4]arene-linked tryphenylene-based oligomers.26 In our previous study, we have reported stable mesogens over a wide temperature range with a resorcin[4]arene core.27 Study on resorcin[4]arene based derivatives suggest the easy synthesis and functionalization by various linking units and flexible alkoxy side chains, which makes them useful for the researchers who are working on supramolecular compounds, self-assembly, and liquid crystals.
In the present investigation, we report a lower-rim-functionalized octa-substituted resorcin[4]arene core with an alkoxy tail group and the investigation of its liquid crystalline and solar cell applications. We prepared four derivatives (D1–D4) with different alkoxy side chains (–OR) to understand the structure–property relationship towards the molecular systems. The materials with longer alkoxy side chains displayed a higher mesophase temperature range as compared to those with shorter alkoxy side chains. Moreover, we prepared thin films using these supramesogens based on resorcinarene with octa-substituted alkyl arms by thermally annealing in air at two different temperatures (100 °C and 200 °C) and investigated their optical, and electrical properties. The findings warrant implication of the amterials and layer in seocnd and third generation solar cells and a possible replacement for toxic cadmium-based optical window and constituent layers in devices.
2. Materials and methods
1,3-Dimethoxy benzene, 3,4-dihydroxybenzaldehyde, aq. HCl, and anhydrous K2CO3 were purchased from Sigma-Aldrich and Avra and the alkyl bromides (R–Br) from Loba Chemical. The solvents were purified before using in the reaction by the usual established method. The glassware used in the experiment was soaked in 5% HNO3 overnight and cleaned with double-distilled deionized water before use. Thin-layer chromatography (TLC) was carried out using silica gel Si 60-F254 (Merck) for reaction monitoring using dichloromethane
:
methanol (9.5
:
0.5 v/v) as the mobile phase and observed under UV light. Melting points were recorded using an Opti-Melt (automated melting point apparatus). The FT-IR spectra were recorded using a Bruker Alpha (diamond crystal) ATR spectrometer in the range of 4000–400 cm−1. The texture images were obtained using a Nikon Eclipse LV-100 POL optical polarizing microscope (POM) equipped with a LTSE 420 heating stage (Linkam Scientific Instruments, Tadworth, Surrey, UK) for temperature regulation and digital camera. Further, the conformations of the mesophase state were measured employing Shimadzu DSC-50 with heating and cooling rates of 10 °C min−1. The 1H NMR spectra were recorded using a Bruker (Ascend 500 MHz) spectrometer with deuterochloroform (CDCl3) as the solvent. The 13C NMR spectra were recorded using a Bruker (Ascend 500) 126 MHz spectrometer with deuterochloroform (CDCl3) as the solvent. In 1H NMR, chemical shifts are reported in δ ppm.
3. Results and discussion
3.1 Synthesis and characterization
The synthetic route for the final target resorcin[4]arene-based materials is presented in Scheme 1. The resorcin[4]arene core (C) was synthesized by the reaction of 1,3-dimethoxy benzene with 3,4-dihydroxybenzaldehyde in the presence of HCl.28 Further, this rigid core (C) was subjected to O-alkylation using various alkyl bromides (R–Br) in the presence of anhydrous K2CO3 and DMF as a solvent to yield the corresponding resorcinarene-based derivatives (D1–D4).29 The structures of all the molecules were established by 1H NMR, 13C NMR, MALDI TOF MS, and IR spectroscopy. The complete details of the synthesis and characterization are provided in the ESI.†
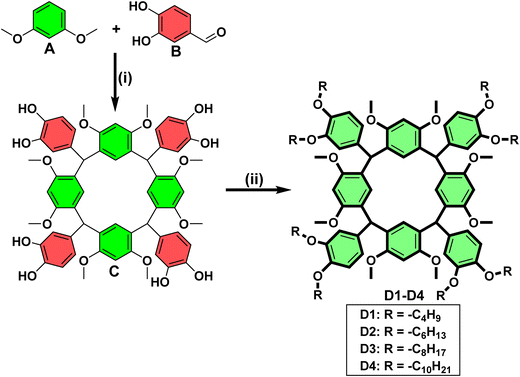 |
| Scheme 1 (i) Aq. HCl, ethanol, reflux, 72 h. (ii) R–Br, anhydrous K2CO3, DMF, reflux, 24 h. | |
3.1.1 General process for the preparation of compound C.
1,3-Dimethoxy benzene (3.82 mmol) was stirred in ethanol, then con. HCl (9.0 M, 0.8 mL) was added dropwise at room temperature. Next, 3,4-dihydroxybenzaldehyde (3.82 mmol) was added dropwise to the reaction mixture. The reaction mixture was then stirred for 72 h at refluxing temperature.28 The reaction was monitored by TLC and on completion, the reaction mixture was cooled to room temperature and put into ice-water. The obtained product was washed with cold methanol and dried in an oven at 50 °C, affording a light-pink solid of octa-O-methoxy resorcin[4]arene (compound C). Yield: 73%, M.P.: 390 °C, FT-IR (diamond crystal) in cm−1; 3462.47 (–OH, phenols), 2930.75 (–C–H, alkane, stretching), 1583, 1505 (–C
C–, aromatic), 1457 (–C–H, alkane, bending), 1197, 1022 (–C–O–, ethers), 810 (
C–H, meta-aromatic bending), 621 (–C–C–, alkane). 1H NMR (500 MHz, CDCl3) δ ppm: 9.89 (s, 8H, –OH), 7.20 (s, 4H, ArH), 7.10 (d, 4H, ArH, J = 7.9 Hz), 7.6 (d, 4H, ArH, J = 7.7 Hz), 6.93 (s, 4H, ArH), 6.88 (s, 4H, ArH), 5.28 (s, 4H, –CH), 3.83 (s, 24H, –OCH3). 13C NMR (126 MHz, CDCl3) δ ppm: 157.77, 147.04, 145.14, 138.61, 132.02, 122.10, 121.71, 115.00, 114.37, 98.02, 55.51, 31.91. MALDI TOF MS for compound C (M + 1) calculated: 1033.0932; found 1034.134.
3.1.2 General process for preparation of compounds D1–D4.
The final target compounds (D1–D4) were prepared by the alkylation of compound C with various alkyl bromides (R–Br). In a round bottom flask (RBF), the core moiety (compound C) (1.031 mmol) and anhydrous K2CO3 were dissolved in DMF followed by stirring the reaction mixture for 30 minutes at room temperature. Then, the respective alkyl bromides (8.4 mole equivalent) were added dropwise in the mixture and heated in an oil bath at reflux temperature for 24 hours. TLC was used to monitor the reaction using dichloromethane
:
methanol (9.5
:
0.5 v/v) as the mobile phase, to ensure that all of the starting ingredients had been consumed. The RBF was then cooled to room temperature, and then quenched in ice-water. The resultant crude residue was refined using methanol trituration.29
Characterization of 2,4,6,8-tetrakis(3,4-dibutoxyphenyl)-14,16,34,36,54,56,74,76-octamethoxy-1,3,5,7(1,3)-tetrabenzenacyclooctaphane (D1).
Yield: 69%, FT-IR (diamond crystal) in cm−1: 2913 (–C–H alkane, stretching), 2874 (–C–H alkane), stretching (methoxy), 1578, 1495 (–C
C– aromatic), 1447, 1399 (–C–H alkane, bending), 1153, 1051, 1036 (–C–O– ethers), 823 (
C–H aromatic bending). 1H NMR (500 MHz, CDCl3) δ ppm: 7.11 (s, 4H, ArH), 6.94 (d, 4H, ArH, J = 7.8 Hz), 6.90 (d, 4H, ArH, J = 7.4 Hz), 6.81 (s, 4H, ArH), 6.62 (s, 4H, ArH) 5.33 (s, 4H, –CH), 4.10 (t, 16H, –OCH2–) 3.76 (s, 24H, –OCH3), 1.81 (m, 16H, –CH2) 1.46 (m, 16H, –CH2), 0.88 (t, 24H, –CH3). 13C NMR (126 MHz, CDCl3) δ ppm: 157.11, 149.59, 149.23, 135.33, 130.59, 121.59, 119.59, 114.15, 113.29, 99.82, 68.29, 58.05, 34.10, 31.93, 29.67, 29.64, 29.60, 29.56, 26.36, 29.11, 26.34, 25.98, 22.70, 14.13. MALDI TOF MS for compound D1 (M + 1) calculated: 1481.9623; found 1482.842.
Characterization of 2,4,6,8-tetrakis(3,4-bis(hexyloxy)phenyl)-14,16,34,36,54,56,74,76-octamethoxy-1,3,5,7(1,3)-tetrabenzenacyclooctaphane (D2).
Brown solid, yield: 66%, FT-IR (diamond crystal) in cm−1: 2912 (–C–H alkane, stretching), 2871 (–C–H alkane), stretching (methoxy), 1580, 1492 (–C
C– aromatic), 1446, 1397 (–C–H alkane, bending), 1158, 1049, 1028 (–C–O– ethers), 822 (
C–H aromatic bending). 1H NMR (500 MHz, CDCl3) δ ppm: 7.10 (s, 4H, ArH), 6.96 (d, 4H, ArH, J = 7.7 Hz), 6.91 (d, 4H, ArH, J = 7.4 Hz), 6.80 (s, 4H, ArH), 6.62 (s, 4H, ArH) 5.31 (s, 4H, –CH), 4.09 (t, 16H, –OCH2–) 3.75 (s, 24H, –OCH3), 1.76 (m, 16H, –CH2) 1.56 (m, 16H, –CH2), 1.31 (m, 32H, –CH2), 0.88 (t, 24H, –CH3). 13C NMR (126 MHz, CDCl3) δ ppm: 156.88, 150.91, 149.78, 136.43, 130.59, 121.31, 119.29, 114.14, 113.35, 99.38, 68.29, 58.48, 14.13. MALDI TOF MS for compound D2 (M + 1); calculated: 1706.3943; found 1707.464.
Characterization of 2,4,6,8-tetrakis(3,4-bis(octyloxy)phenyl)-14,16,34,36,54,56,74,76-octamethoxy-1,3,5,7(1,3)-tetrabenzenacyclooctaphane (D3).
Light yellow solid, yield: 73% FT-IR (diamond crystal) in cm−1: 2914 (–C–H alkane, stretching), 2848 (–C–H alkane), stretching (methoxy), 1576, 1501 (–C
C– aromatic), 145, 1376 (–C–H alkane, bending), 115, 1095, 1026 (–C–O– ethers), 826 (
C–H aromatic bending). 1H NMR (500 MHz, CDCl3) δ ppm: 7.10 (s, 4H, ArH), 6.94 (d, 4H, ArH, J = 7.8 Hz), 6.90 (d, 4H, ArH, J = 7.3 Hz), 6.80 (s, 4H, ArH), 6.62 (s, 4H, ArH) 5.29 (s, 4H, –CH), 4.09 (t, 16H, –OCH2–) 3.75 (s, 24H, –OCH3), 1.80 (m, 16H, –CH2) 1.46 (m, 16H, –CH2), 1.33 (m, 64H, –CH2), 0.88 (t, 24H, –CH3). 13C NMR (126 MHz, CDCl3) δ ppm: 157.18, 149.56, 148.20, 135.67, 130.59, 121.81, 120.13, 114.14, 112.73, 96.66, 68.28, 56.36, 34.78, 31.94, 29.71, 29.69, 29.67, 29.60, 29.56, 29.37. 29.11, 26.33, 25.99, 22.70, 14.13. MALDI TOF MS for compound D3 (M + 1); calculated: 1930.8232; found 1931.943.
Characterization of 2,4,6,8-tetrakis(3,4-bis(decyloxy)phenyl)-14,16,34,36,54,56,74,76-octamethoxy-1,3,5,7(1,3)-tetrabenzenacyclooctaphane (D4).
Light brown solid, yield: 78% FT-IR (diamond crystal) in cm−1: 291 (–C–H alkane, stretching), 28
506 (–C–H alkane), stretching (methoxy), 157, 1501 (–C
C– aromatic), 1443, 138 (–C–H alkane, bending), 1184, 1087, 102 (–C–O– ethers), 826 (C–H aromatic bending). 1H NMR (500 MHz, CDCl3) δ (ppm): 7.10 (s, 4H, ArH), 6.95 (d, 4H, ArH, J = 7.7 Hz), 6.89 (d, 4H, ArH, J = 7.6 Hz), 6.80 (s, 4H, ArH), 6.62 (s, 4H, ArH) 5.30 (s, 4H, –CH), 4.09 (t, 16H, –OCH2–) 3.7 (s, 24H, –OCH3), 1.82 (m, 16H, –CH2) 1.46 (m, 16H, –CH2), 1.34 (m, 96H, –CH2), 0.88 (t, 24H, –CH3). 13C NMR (126 MHz, CDCl3) δ (ppm): 156.29, 149.25, 148.70, 134.84, 130.46, 121.18, 119.82, 114.92, 113.84, 99.68, 68.23, 56.36, 35.39, 31.76, 30.48, 29.89, 29.47, 29.16, 28.88, 25.02, 22.56, 14.43. MALDI TOF MS for compound D4 (M + 1); calculated: 2155.2564; found 2156.364.
3.1.3 Reaction scheme.
3.2 POM investigation
The mesomorphic behaviour of the octa-substituted lower-rim-functionalized resorcin[4]arene derivatives (D1–D4) was preliminarily investigated using polarizing optical microscopy (POM). The mesophase texture patterns observed at different temperatures for all four materials with variable alkyl chains are shown in Fig. 1. The parent resorcinarene core (C) with free hydroxy group showed non-liquid crystalline properties, which may be due to the absence of any kind of flexible alkyl group to provide flexibility to the central rigid core, so the compound directly reached the isotropic state on heating without exhibiting any liquid crystalline state, while it crystallized on cooling. We studied the flexibility, rigidity, dipole moment and polarizability effect on liquid crystalline properties for all the four supramolecular compounds (D1–D4) with octa-substituted alkoxy side chains towards the central rigid core.30–32 All these supramolecular compounds (D1–D4) displayed columnar type mesogenic behaviour at higher temperatures with broad thermal stability. The resorcinarene-based materials displayed an enantiotropic liquid crystalline property. The textures of the supramolecular mesogens were studied and captured on cooling. All these supramolecular systems displayed typical texture pattern of columnar hexagonal phase at 186. 0 °C, 182.0 °C, 174.0 °C and 170.0 °C, and further transformed into isotropic state at 218.0 °C, 224.0 °C, 230.0 °C and 232.0 °C. Further, POM texture images were captured at 181.0 °C, 182.0 °C, 174.0 °C and 153.0 °C on cooling. To achieve liquid crystalline properties, end-to-end interactions play an important role, which depend on the polarity and degree of unsaturation of the terminal side positions, which affect its polarizability nature.33 The phase behaviour of the synthesized compounds is shown in Fig. 2, which clearly indicates the descending tendency of the mesomorphic properties. The graph shows that the lower alkoxy side chain substituted compounds have lower mesophase stability as compared to the higher alkoxy side chain substituted compounds.
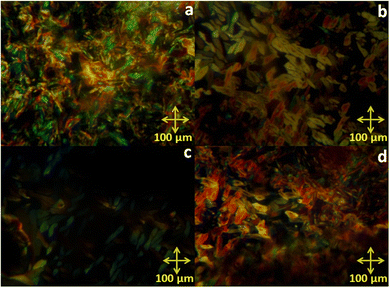 |
| Fig. 1 POM texture images taken during the cooling cycle: D1 at 181.0 °C on crossed polarizers, cooling at 1 K min−1 (a). D2 at 182.0 °C on crossed polarizers, cooling 1 K min−1 (b). D3 at 174.0 °C on crossed polarizers, cooling at 1 K min−1 (c). D4 at 153.0 °C on crossed polarizers, cooling at 1 K min−1 (d). | |
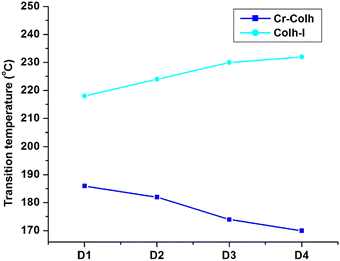 |
| Fig. 2 Phase behaviour of the synthesized resorcinarene-based compounds (D1–D4). | |
The plot of the observed transition temperatures vs. the number of carbon atoms in the alkoxy side chains (Fig. 2) for the alkoxy-substituted liquid crystalline materials display a descending tendency of isotropic to columnar hexagonal phase and columnar hexagonal mesophase to solid crystalline phase temperature with gradually increasing chain length. This may be due to the higher polarizability of the higher alkoxy side chains with more alkyl spacer as compared to lower alkyl chains. Additionally, the mesophase formation requires sufficient alkyl spacers for the molecular system and also effect on its thermal stability, self-assembly type order, and mesophase range. The mesophase thermal stability increased with increasing side arm length. To better understand the mesophase in the synthesized materials, we observed the phase sequence of compound D4 at different temperatures on cooling (Fig. 3).
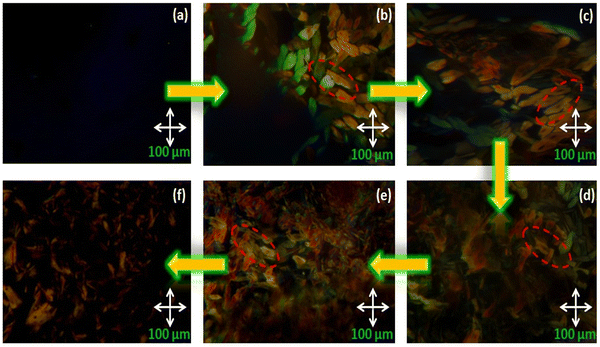 |
| Fig. 3 Phase sequence of compound D4 at different temperatures: isotropic state at 232.0 °C (a); mesophase at 170.0 °C (b); mesophase at 164.0 °C (c); mesophase at 159.0 °C (d); mesophase at 153.0 °C (e); solid crystal at 142.0 °C (f). | |
3.3 Thermal behaviour of the compounds
The thermotropic properties of the synthesized materials were preliminarily studied using DSC. All the four materials with different alkyl chain lengths display two endothermic peaks on heating and cooling, which denote the presence of a mesophase in an enantiotropic manner. Further, the appearance of high enthalpy values in the first observed transition is mainly caused by the melting of the semi-crystalline flexible alkoxy side chain-based compounds, which is also responsible for the exhibition of the mesomorphic transition state. Compounds D1 and D2 with butyloxy and hexyloxy tail groups displayed their first transition peaks for solid crystal to mesophase at 184.2 °C and 181.9 °C on heating, while on further heating the mesophase transforms to isotropic state at 214.7 °C and 209.3 °C, respectively. Likewise, compounds D3 and D4 with octyloxy and decyloxy side chains display their first endothermic peaks at 175.2 °C and 169.5 °C, and on further heating, they show their second endothermic peaks at 226.1 °C and 232.8 °C, respectively, which confirms the existence of a mesophase to isotropic phase transition, as confirmed by POM and XRD analysis. The DSC thermogram of all the four derivatives on heating and cooling are presented in Fig. 4. Further, on cooling, all these derivatives again revealed two endothermic peaks close to where the peak appeared upon heating. D1, D2, D3 and D4 showed their first exothermic peaks at 176.4 °C, 173.8 °C, 170.7 °C and 167.2 °C, respectively, corresponding to the transition from solid to mesomorphic state, and their second exothermic peaks appeared at 209.3 °C, 216.8 °C, 221.4 °C and 227.5 °C, respectively, corresponding to the transition from mesophase to isotropic state. It can be noted that higher alkoxy side chain (–OR) substituted compounds (D3, D4) displayed higher mesophase temperature ranges and mesophase stability owing to the presence of the higher alkoxy side chains, which leads to the more flexibility towards the central octa-side functionalized resorcinarene core and enables the formation of a self-assembly type arrangement of molecules in the mesomorphic transition state.34 Likewise, other factors such as the polarity, polarizability, and dipole moment of the molecules affect their molecular interactions and forces acting behind the arrangement of molecules in the mesomorphic state.35 The transition temperature and associated enthalpy values are mentioned in Table 1. One can observe that the first transition, corresponding to solid crystal to mesophase, exhibits a high enthalpy value, which can be attributed to the melting transition of the crystal to the isotropic phase. The bar graphs clearly express the higher mesophase transition range observed in all the synthesized supramolecules based on the resorcinarene rigid core (Fig. 5). Thus, the present octa-substituted resorcinarene-based compounds behave like thermally stable thermotropic mesogens, which is further supported by the high-temperature POM and XRD analyses. Further, we noted that the present lower-rim-functionalized octa-substituted resorcin[4]arene-based supramolecular materials display better stability and a higher temperature ranges as compared to previously reported upper rim functionalized resorcinarene-based supramolecular compounds, which may be due to the lower rim functionalization of the central core with different moieties, which leads to a more stable, labile and thermo-responsive confirmation for the whole molecular system.
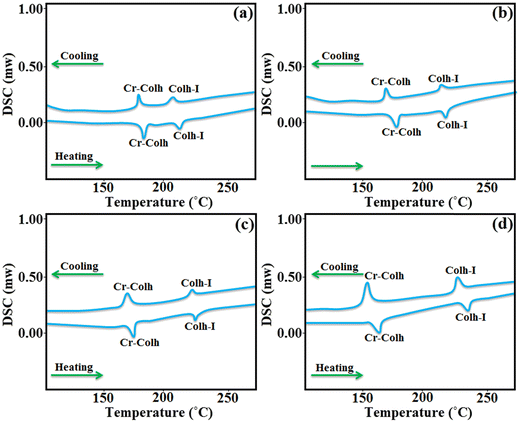 |
| Fig. 4 DSC traces of D1 (a); D2 (b); D3 (c); and D4 (d) on the second heating and cooling cycles (scan rate 10 °C min−1). | |
Table 1 Transition temperaturesa (°C) and enthalpies (kJ mol−1) of the synthesized supramolecules (second heating and cooling scans)
Compound |
Phase sequence |
Heating |
Cooling |
Peak temperatures observed on second heating and cooling cycles at 10 °C min−1.
|
D1
|
Cr 184.2 (22.8) Colh 214.7 (8.3) I |
I 209.3 (3.1) Colh 176.4 (20.1) Cr |
D2
|
Cr 181.9 (26.2) Colh 218.5 (7.3) I |
I 216.8 (5.9) Colh 173.8 (17.6) Cr |
D3
|
Cr 175.2 (19.4) Colh 226.1 (4.6) I |
I 221.4 (7.5) Colh 170.7 (23.5) Cr |
D4
|
Cr 169.5 (21.6) Colh 232.8 (3.3) I |
I 227.5 (9.1) Colh 167.2 (28.7) Cr |
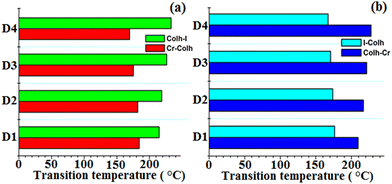 |
| Fig. 5 Bar graphs showing the thermal behaviour of the prepared supramesogens (D1–D4) on heating and cooling. | |
The thermal stability of the synthesized octa-substituted resorcinarene-functionalized derivatives with alkyl arms was studied using TGA. The thermograms of all the four supramolecular compounds (D1–D4) are presented in Fig. 6. All these materials exhibited single-stage type thermal decomposition behaviour. Interestingly, compounds with lower alkoxy side spacers (D1, D2) showed a slightly different type of weight loss as compared to the higher alkoxy side substituted compounds (D3, D4). From all the four synthesized materials, D3 and D4 with octyloxy (–OC8H17) and decyloxy (–OC10H21) tail groups displayed the maximum weight loss of nearly 67% at the range of 370–410 °C (Tmax = 405 °C). However, the remaining residues were completely decomposed at nearly 650 °C. Similarly, compounds D1 and D2 with butyloxy (–OC5H11) and hexyloxy (–OC6H13) arms exhibited 63% weight loss in the temperature range of 362–402 °C and further showed complete degradation at around 630 °C. The decomposition of the materials takes place due to the breaking of the alkoxy side group at the eight-sided terminal end of the resorcinarene core in the lower rim position.36 The obtained results suggest the substitution of higher alkoxy side chains leads to higher weight loss as compared to lower chain substituted compounds. Likewise, the presence of the central resorcinarene core with lower rim substitution is responsible for the higher thermal stability of the supramolecular compounds as compared to upper rim-substituted resorcinarene-based liquid crystalline materials. The TGA study confirmed that all the synthesized lower-rim-substituted materials displayed higher thermal stability of mesophase with a broad temperature range.
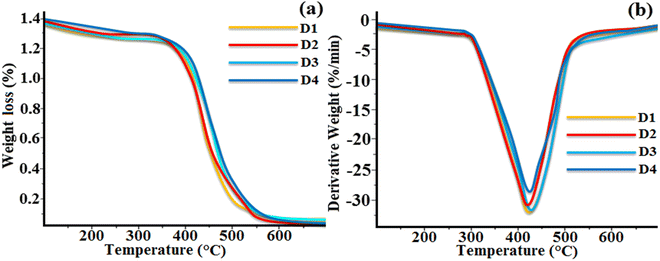 |
| Fig. 6 (a) TGA and (b) DTG spectra of D1–D4 at a heating rate of 20 °C min−1 under N2. | |
3.4 SAXS/WAXS analysis
The presence of a columnar type mesophase was preliminarily examined using POM and DSC analysis and the molecular arrangement pattern in liquid crystalline state was further confirmed by small- and wide-angle X-ray scattering (SAXS/WAXS) study. The XRD investigation of the synthesized target materials were performed at the transition temperatures obtained by POM and DSC analysis. The XRD data for all the four derivatives (D1–D4) is given in Fig. 7. All these materials with variable alkyl chains showed similar diffraction patterns in the lower and higher angle regions. All these derivatives showed a sharp diffuse peak in the lower angle region with two small reflections, and two diffuse signals in the higher angle region, which clearly indicated the presence of a liquid-like order of the aliphatic alkyl chains and π–π stacking behaviour of the central functionalized lower-rim-substituted resorcinarene derivatives with the presence of terminal arms. The SAXS/WAXS pattern of D1 with a butyloxy tail group at 176.0 °C showed three peaks at 4.6°, 7.6° and 8.7° in the small angle region, and d-spacing values were found to be 19.20 Å, 11.64 Å and 10.18 Å, respectively. Similarly, D2 with a hexyloxy tail group displayed three peaks at 4.2°, 7.3° and 8.6° with the d-spacing values calculated to be 21.02 Å, 12.12 Å and 10.30 Å, respectively. The obtained diffraction pattern indicates that the ratio of the observed reflections was found to be 1
:
1/√3
:
1/√4, which clearly confirms the presence of peaks corresponding to (100), (110) and (200) planes found in the hexagonal lattice in columnar hexagonal-type mesogens. Likewise, D3 with an octyloxy tail group exhibited three diffraction peaks at 3.6°, 7.1° and 8.3° and the calculated d-spacings are 24.52 Å, 12.45 Å and 10.67 Å, respectively. As with the other three derivatives, D4 with a decyloxy tail group displayed three diffraction peaks at 3.4°, 6.8° and 8.1° and the d-spacings were calculated to be 25.96 Å, 13.01 Å and 10.92 Å, respectively. Additionally, in the broad angle region, diffraction peaks appeared at 22.8° and 23.7° for D1, 21.9° and 22.8° for D2, 21.8° and 22.6° for D3, and 21.9° and 23.8° for D4. The d-spacing values for the first broad halo (ha) peak were found to be 3.97 Å, 4.12 Å, 4.14 Å and 4.12 Å, respectively, which confirms the molecular arrangement with respect to the central rigid core unit while the d-spacing values of the other weak peaks (hc) were found to be 3.83 Å, 3.97 Å, 4.01 Å and 3.81 Å, respectively. The presence of one broad peak (ha) and one weak peak (hc) in all the four supramolecular compounds are mainly due to presence of π–π stacking behaviour in the formation of the columnar self-assembly with core–core interactions of the resorcinarene-functionalized supramolecular derivatives with alkyl arms.37 Furthermore, the lower-rim-functionalized resorcinarene-based materials have a stable columnar mesophase that is further enhanced by the functionalized outside core inbuilt with conducting arms, which further hold the column matrix in systematic way.
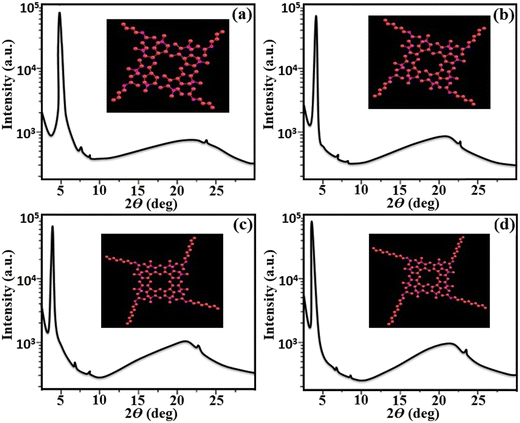 |
| Fig. 7 Diffraction patterns of D1 at 176.0 °C (a), D2 at 173.0 °C (b), D3 at 169.0 °C (c), and D4 at 164.0 °C (d). The insets show the ball and stick models of the target resorcinarene-based compounds. | |
In the literature, similar types of diffraction patterns have been reported for supramolecular LCs with columnar type molecular arrangement with self-assembly nature.38–40 The molecular lengths of the target lower-rim-substituted resorcinarene-based mesogens (D1–D4) were estimated to be 20.07 Å, 21.55 Å, 23.97 Å and 26.17 Å, which is near to the observed molecular length of the eight-side substituted resorcinarene-based target supramolecular systems. Small angle X-ray diffraction study was carried out at the transition temperature, with the obtained d-spacing in the low angle region closely fitting into a hexagonal lattice. The obtained result was further confirmed by the texture pattern observed by POM. The systematic representation of the molecular arrangement in the columnar hexagonal phase with eight-side functionalized alkoxy tail linked resorcinarene derivatives with octyloxy side arm (D4) is shown in Fig. 8. The equatorial reflections indicate the two-dimensional positional order of the respective column, which is further assigned to the hexagonal columnar type of pattern. In addition, this type of supramolecular liquid crystalline compound based on resorcinarene core with stable bowl-shaped confirmation stabilized columnar mesophase at higher temperature with broad thermal stability, which makes this supramolecular system more suitable for the electro-optic device applications.
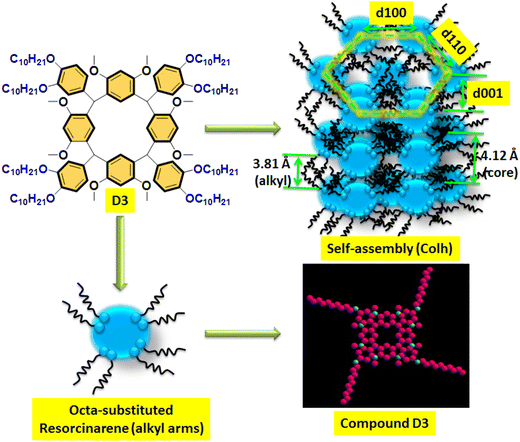 |
| Fig. 8 Proposed mechanism of the columnar hexagonal-type molecular arrangement in D4 with self-assembly nature. | |
3.5 Thin film deposition
The supramolecular liquid crystal based thin films of D4 were fabricated using solution processed spin coating and ex situ drop cast recipes where spin coating is undertaken for one coat at 3500 rpm for 10 minutes on ITO and glass substrates. The spin coat is followed by drop casting through a medicals syringe where entire surface of the film is filled by target liquid followed by dessication at room temperature till dryness. Prior to the deposition of the thin films, the ITO and glass substrates of dimesions 10 mm × 10 mm × 1 mm were cleaned with acetone and isopropyl alcohol to minimize contamination and remove any impurities present on the substrate surface along with explicit adhesion. After that, the as-deposited thin films were annealed in air ambient at temperature 100 °C and 200 °C. The thin films of alkyl arm-functionalized resorcinarene-based mesogens were employed as optical window in a CdTe-based solar cell device to achieve high efficiency with lower toxicity.41 The preparation of the thin films and the proposed device architecture are shown in Fig. 9 where the optimized films could be employed as either optical window in second geneartion or transport layer in third generation devices where optimization of the other constituents need to be done in accordance with the device design and conductivity nature of the corresponding absorber counterpart.
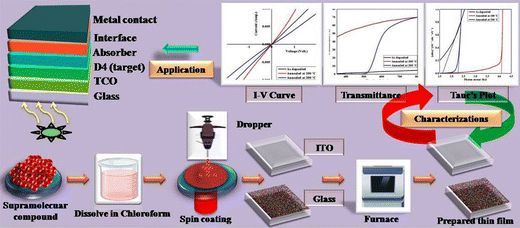 |
| Fig. 9 The preparation of the supramesogen (D4)-based thin films and the anticipated device structure where the optimized layers could be employed as optical window in second and transport layer in third generation devices based upon the conductivity nature which need to be explored in accordance with device design. | |
3.6 Optical study
Liquid crystalline compounds have a wide range of properties, including thermal stability, mobility, charge-transport and anisotropic nature, those make them more advanced as compared to solid crystalline materials and other inorganic toxic salts.45 Thus, our aim is to measure the physical properties of the present synthesized lower-rim-functionalized resorcinarene-based derivatives in order to implicate these in target applied domain where operative optical absorbance and transmittance together with effectual electrical conductivity are important. For application as the buffer layer in a solar cell, the decyloxy-linked resorcinarene-based material is found suitable owing to its thermal stability, mobility, optical and liquid crystalline properties. In order to understand implication of optimized supramolecular materials with decyloxy tail group (–OC10H21) as optical window and transport layers in target applied domain with environmental aspects, associated thin films are deposited on glass and ITO substrates. The absorbance and transmittance spectra were measured in the wavelength range of 350–800 nm followed by evaluating the extinction coefficient and optical energy band-gap of the processed thin film layers in order to optimize for the target application domain. The absorbance and transmittance spectra of the as-deposited and annealed thin films are presented in Fig. 10. As visible in Fig. 10a, the absorbance spectra for the pristine and 100 °C annealed thin films slightly descend in the entire wavelength range. For the 200 °C annealed films, it varied in nearly UV and visible regions within 360–480 nm, further rapidly descended upto 600 nm and after that it seems to slightly decrease, and at last it behaves in a constant way in the higher visible region. The right edge of the spectrum is red-shifted may be attributed to the size effect which impacts the spin–orbit coupling and restrains the exciting positions.42 For 200 °C annealed films, more rapid red shift in absorption is visble with a broad intense peak at 405 nm owing to its mesomorphic conditions which lead to higher mobility as compared to the as-deposited and 100 °C conditions. Additionally, the prisitine resorcinarene-based thin films showed a lower band gap as compared to its annealed films. Further, it could affect and move towards red shift when it links with octa-substituted decyloxy side chain towards the symmetrical side of rigid resorcinarene core. The red shift with respect to higher absorption in both UV and visible regions is a key factor for the fabrication of solar cells.43 The present synthesized resorcinarene-based liquid crystal films exhibit a blue shift in band gap for the 100 °C and 200 °C processed films, while with respect to 100 °C, it red shifted for the 200 °C annealed films. The synthesized compounds have a good electronic nature with the presence of π-electron cloud-based molecular system with peripheral alkoxy side chains, which is responsible for the reactivity of the present synthesized supramolecular compounds. In addition, it can also be calibrated for the absorption of light at a particular wavelength by synthesizing organic compounds with a number of different types of light-absorbing units. Importantly, the optical absorption coefficients of organic-based materials are higher compared to those of inorganic materials, so a small amount of this kind of material absorbs a high amount of energy.44 The extinction coefficient of the D4-based thin films with doped conditions is illustrated in Fig. 11. As visible for the 200 °C, it shows a descending tendency up to 550 nm wavelength region and then slightly decreased in the higher visible region may be due to surface roughness and associated pores present in the prepared thin films.45 The extinction coefficient of the pristine films is higher compared to annealed films with increasing trend across the entire region of probe. For films annealed at 100 °C, it remain almost constant and as stated it decreased when annealed at 200 °C which may be attributed towards isotropic liquid state of the processed material.
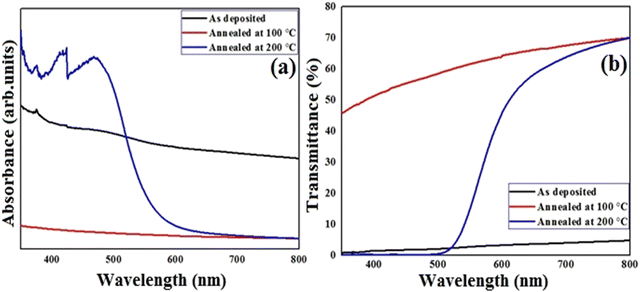 |
| Fig. 10 Absorbance (a) and transmittance (b) spectra of pristine and air-annealed supramesogen (D4)-based thin films. | |
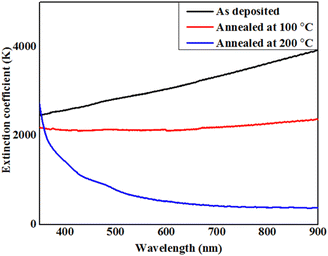 |
| Fig. 11 Behaviour of extinction coefficient with wavelength for prepared supramesogen (D4)-based thin films. | |
The optical transmittance spectra of the pristine and annealed films are presented with wavelength in Fig. 10b where almost reciprocal trend is obtained with respect to the absorbance. The as-deposited supramolecular compound-based thin films displayed lower transmittance which augmeneted higher for film annealed at 100 °C, later transmittance is found to be varied for film annealed at 200 °C with wavelength. For these films, transmittance is enhanced in far visible and near infrared regions. The observed variation in transmittance behaviour may be due to change in crystallinity and grain growth together with surface morphology of the films. The optical energy band-gap of the pristine and annealed resorcinarene-based doped thin films was determined employing the emperial Tauc's relation. The Tauc's plots for the selected material with the decyloxy tail and room-temperature mesogenic properties is shown in Fig. 12. The optical band gaps analyzed by Tauc's plots were found to be 1.61 eV, 4.08 eV and 2.27 eV for the pristine lower rim-functionalized resorcinarene-based and annealed films. The synthesized resorcinarene-based materials showed good electronic behaviour and reactivity owing to the active site of the molecular system and further the effect of temperature may impact the band-gap, suggesting the blue shift in band gap after annealing.46 On the other hand, the increasing alkyl chain on the terminal end towards the molecular systems may impact the optical energy band-gap which further could be affected by the active sites of the central substituted resorcinarene-based rigid core. However, the band-gap is increased with annealing revealed to the thermal stability of the supramolecular compounds. The stable confirmation is more suitable to fulfill the need for a window layer material having a non-toxic nature. The prepared supramolecular functionalized resorcinarene compounds with stable columnar hexagonal mesophase with obtained band-gap could be good candidates to apply as the window layer in solar cell applications as compared to toxic metal salt of inorganic materials while prior the device development, as stated the individual optimization also plays crucial role in accordance with device design for decent performance if obtains.
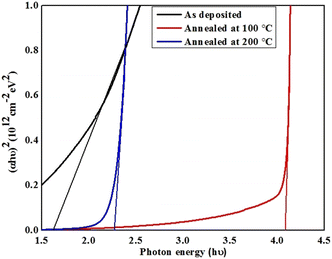 |
| Fig. 12 Tauc's plots for pristine and air-annealed supramesogen (D4)-based thin films. | |
3.7 Electrical properties
The transverse current–voltage (I–V) curves for the as-deposited/pristine and annealed resorcinarene-based thin films are given in Fig. 13. The current–voltage curves for the resorcinarene-based thin films clearly demonstrates the linear dependence of current on voltage in both the forward and reverse directions, which revealed to the ohmic behaviour of contacts existed between the thin films surface and ITO substrate layer. Herein, the contacts are formed by adhesive silver paste by using thin copper wires with negligible resistance one on fims surface and another on ITO where the ITO part was masked by thin tape and its removal was followed by cleaning with acetone. For 100 °C annealed films, the current increased revealed to increment in the conductivity of these resorcinarene-based thin films. Later, the conductivity is decreased for the film annealed at 200 °C. The observed increment in conductivity of the resorcinarene-based thin film may be due to the enhancement in the crystal size and the reduction of crystal defects, while contamination during the annealing process might lead to a reduction in conductivity.47
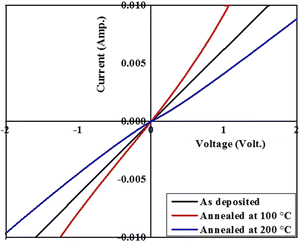 |
| Fig. 13 The current–voltage curves of the as-deposited and annealed D4-based thin films. | |
4. Conclusion
In summary, we have designed and successfully synthesized resorcinarene-linked alkyl arms-based supramolecular mesogens (D1–D4) by the lower rim functionalization of the resorcinarene core by eight-side appended alkyl arms. These supramolecular materials were prepared in good yield and confirmed by using FT-IR, 1H-NMR, 13C-NMR and MALDI TOF analysis. All the four lower-rim-functionalized resorcinarene derivatives with alkyl arms displayed enantiotropic columnar mesophase with a broad temperature range. The structure–property relationship was studied by varying the alkyl side chain on the terminal end to understand the effect on the liquid crystalline properties. This work clearly illustrates the stable confirmation based on the lower rim functionalization of the resorcinarene core with a simple two-step method for the preparation of supramolecular materials with stable columnar mesophase by aquiring broad thermal stability, making them strong candidates for the target applied domain, which could further impacts the economic cost of the devices. Further, to tune the properties, the selected material with high alkyl chain length (D4) was used to prepare a thin film and their optical and electrical behaviour were studied for optical window and constituent layer applications in solar cell devices. The resorcinarene-functionalized material-based thin film exhibited ohmic-type behaviour for all the pristine and annealed films. The prepared films exhibited suitable electrical conductivity and optical energy band gap, which clearly suggests implication of resorcinarene-based mesogenic substance in device technology. These supramolecular LCs with stable crown conformation and non-toxic nature make them strong candidates to implicate as the optical window in second and transport layer in third generation devices which is not only important for the environment but for operators in the manufacturing process too. Furthermore, the flexibility of these organic-based soft materials makes them suitable for cost-effective fabrication of organic solar cells in large volume while the other constituents need optimization separately in accordance with device architecture for all the suggested generations.
Conflicts of interest
There are no conflicts to declared.
Acknowledgements
The authors gratefully acknowledge the Department of Chemistry, School of Science, Gujarat University, Ahmedabad for providing the necessary lab and infrastructure facility to carry out this work. Vinay S. Sharma gratefully acknowledges the Human Resource Development Group-Council of Scientific & Industrial Research (CSIR), New Delhi for Research Associate Fellowship (File No. 09/070(0075)/2020-EMR-I). Suryajit L. Rathod gratefully acknowledges the Human Resource Development Group-Council of Scientific & Industrial Research (CSIR) New Delhi for CSIR-NET JRF Fellowship (File No. 09/070(0070)/2019-EMR-I). The authors are thankful to the Center of Excellence, IIT Mandi and IIT Indore for providing analytical and spectral services. We thank Dr Sunil Kumar (Department of Chemistry, MS University, Vadodara) for providing valuable comments on the XRD analysis. The Department of Physics, Mohanlal Sukhadia University, Udaipur is acknowledged for thin film development and characterization facilities.
References
-
(a) V. Marappan, Y. C. Hsu, J. T. Lin and C. W. Chang,
et al.
, Chem. – Asian J., 2010, 5, 87 CrossRef;
(b) A. C. Mayer, S. R. Scully, B. E. Hardin and M. W. Rowell,
et al.
, Mater. Today, 2007, 10, 28 CrossRef CAS.
- H. K. Bisoyi and Q. Li, Chem. Rev., 2021, 122, 4887 CrossRef.
-
(a) K. Pal, A. A. Aljabali, S. Kralj, S. Thomas and F. G. de Souza, Chemosphere, 2021, 263, 128104 CrossRef CAS PubMed;
(b) H. Q. Chen, X. Y. Wang, H. K. Bisoyi, L. J. Chen and Q. Li, Langmuir, 2021, 37(13), 3789 CrossRef CAS.
- M. Ans, K. Ayub, I. A. Bhatti and J. Iqbal, RSC Adv., 2019, 9(7), 3605 RSC.
- L. Y. Lin, L. Q. Jiang, Y. Qiu and B. D. Fan, J. Phys. Chem. Solids, 2018, 122, 19 CrossRef CAS.
- A. Haddout, A. Raidou and M. Fahoume, Appl. Phys. A, 2019, 125(2), 1 CrossRef CAS.
- S. Ghosh, P. Das, B. Bairy, R. Ghosh, S. Dam and M. B. Sen, J. Mater. Sci., 2021, 56(30), 16928 CrossRef CAS.
- D. E. Barry, D. F. Caffrey and T. Gunnlaugsson, Chem. Soc. Rev., 2016, 45, 3244 RSC.
- D. W. Zhang, M. Li and C. F. Chen, Chem. Soc. Rev., 2020, 49, 1331 RSC.
- D. Boosagulla, S. Mandati, P. Misra, R. Allikayala and B. V. Sarada, Superlattices Microstruct., 2021, 160, 107060 CrossRef CAS.
-
(a) H. K. Bisoyi and Q. Li, Prog. Mater. Chem., 2019, 104, 1 CAS;
(b) M. S. Abusaif, M. Fathy, M. A. Abu-Saied, A. A. Elhenawy, A. B. Kashyout, M. R. Selim and Y. A. Ammar, J. Mol. Struct., 2021, 1225, 129297 CrossRef CAS.
-
(a) Z. Yu, H. K. Bisoyi, X. M. Chen and Z. Z. Nie,
et al.
, Angew. Chem., Int. Ed., 2022, 134(16), e202200466 Search PubMed;
(b) X. Zhou, S. W. Kang, S. Kumar and Q. Li, Liq. Cryst., 2009, 36(3), 269 CrossRef CAS;
(c) X. Zhou, S. W. Kang, S. Kumar and R. R. Kulkarni,
et al.
, Chem. Mater., 2008, 20(11), 3551 CrossRef CAS.
- G. Olivo, G. Capocasa, D. D. Giudice, O. Lanzalunga and S. D. Stefano, Chem. Soc. Rev., 2021, 50(13), 7681 RSC.
- R. Lu, X. Zhang, X. Cheng, Y. Zhang, X. Zan and L. Zhang, Front. Chem., 2020, 8, 583484 CrossRef CAS.
- R. Adam, M. Mon, R. Greco, L. H. Kalinke and A. V. Moya,
et al.
, J. Am. Chem. Soc., 2019, 141(26), 10350 CrossRef CAS PubMed.
- A. I. Akhmetshina, E. K. Ignateva, T. R. Deberdeev, L. K. Karimova and Y. N. Yuminova,
et al.
, Polym. Sci., Ser. D, 2019, 12(4), 427 CrossRef CAS.
- L. Mahalakshmi, P. P. Das and T. N. G. Row, J. Chem. Sci., 2008, 120(1), 39 CrossRef CAS.
- Y. Zhou, K. Jie, R. Zhao and F. Huang, Adv. Mater., 2000, 32(20), 1904824 CrossRef.
- P. Timmerman, V. Willem and R. N. David, Tetrahedron, 1996, 52, 2663 CrossRef CAS.
- D. N. Lande, S. A. Bhadane and S. P. Gejji, J. Phys. Chem. A, 2017, 121(8), 1814 CrossRef CAS.
- K. Twum, K. Rissanen and N. K. Beyeh, Chem. Rec., 2021, 21(2), 386 CrossRef CAS.
- K. Jie, Y. Zhou, Y. Yao and F. Huang, Chem. Soc. Rev., 2015, 44(11), 3568 RSC.
- D. Sémeril and D. Matt, Coord. Chem. Rev., 2014, 279, 58 CrossRef.
- J. A. Gajjar, R. H. Vekariya and H. M. Parekh, Synth. Commun., 2020, 50(17), 2545 CrossRef CAS.
- H. Gua, F. Yang, W. Liu and J. Lai, Tetrahedron Lett., 2015, 56, 866 CrossRef.
- H. Tang, H. Guo, F. Yang and S. Zhu, Liq. Cryst., 2017, 44(10), 1566 CrossRef CAS.
-
(a) J. Gajjar, R. H. Vekariya, V. S. Sharma and H. Parekh, Mol. Cryst. Liq. Cryst., 2018, 668, 48 CrossRef CAS;
(b) J. Gajjar, R. H. Vekariya, V. S. Sharma and S. Kher,
et al.
, Mol. Cryst. Liq. Cryst., 2021, 715, 37 CrossRef CAS.
- D. Moore, G. W. Watson, T. Gunnlaugsson and S. E. Matthews, New J. Chem., 2008, 32(6), 994 RSC.
- F. Potet, A. N. Lorinc, S. Chaigne and C. R. Hopkins,
et al.
, J. Biol. Chem., 2012, 287(47), 39613 CrossRef CAS PubMed.
- V. S. Sharma, A. S. Sharma and R. H. Vekariya, New J. Chem., 2018, 42, 15044 RSC.
- M. Skarabot, N. J. Mottram, S. Kaur and C. T. Imrie, ACS Omega, 2022, 7(11), 9785 CrossRef CAS.
- Z. Y. Han, S. Q. Kun, J. T. Kang and Y. G. Jia, J. Mol. Struct., 2022, 1263, 133147 CrossRef CAS.
- M. Hagar, H. A. Ahmed, T. H. El-Sayed and R. Alnoman, J. Mol. Liq., 2019, 285, 96 CrossRef CAS.
- Y. Yuan, J. Li, L. He and H. Zhang, Eur. Polym. J., 2018, 105, 7 CrossRef CAS.
-
(a) U. H. Jadeja, V. S. Sharma, V. Prajapat and A. Shah,
et al.
, Mol. Cryst. Liq. Cryst., 2019, 680(1), 46 CrossRef CAS;
(b) C. A. Yang, Q. Tan, G. Zhong and H. Xie,
et al.
, Polymer, 2010, 51(2), 422 CrossRef CAS.
- C. P. Chai, X. Q. Zhu, P. Wang and M. Q. Ren, Macromolecules, 2007, 40(26), 9361 CrossRef CAS.
- F. Yang, H. Guo, J. Xie and J. Lin, Eur. J. Org. Chem., 2011, 5141 CrossRef CAS.
- V. S. Sharma, A. S. Sharma and R. H. Vekariya, J. Mol. Liq., 2018, 271, 319 CrossRef CAS.
- F. Yang, H. Guo and J. Vicens, J. Incl. Phenom. Macrocycl. Chem., 2014, 80(3), 177 CrossRef CAS.
- S. Jiang, J. Qiu, Y. Chen, H. Guo and F. Yang, Dyes Pigm., 2018, 159, 533 CrossRef CAS.
- D. Agrawal, S. L. Patel, Himanshu, S. Chander, M. D. Kannan and M. S. Dhaka, Optik, 2019, 199, 163307 CrossRef CAS.
- D. Suthar, Himanshu, S. L. Patel, S. Chander, M. D. Kannan and M. S. Dhaka, Solid State Sci., 2020, 107, 106346 CrossRef CAS.
- M. Vadivel, S. Singh, D. P. Singh, V. A. Raghunathan and S. Kumar, J. Phys. Chem. B, 2021, 125, 10364 CrossRef CAS PubMed.
- M. Shaban and A. M. Sayed, Mater. Sci. Semicond. Process., 2016, 41, 323 CrossRef CAS.
-
(a) A. Purohit, S. Chander, A. Sharma, S. P. Nehra and M. S. Dhaka, Opt. Mater., 2015, 49, 51 CrossRef CAS;
(b) L. Li, S. W. Kang, J. Harden and Q. Sun,
et al.
, Liq. Cryst., 2008, 35(3), 233 CrossRef CAS;
(c) Q. Sun, L. Dai, X. Zhou, L. Li and Q. Li, Appl. Phys. Lett., 2007, 91(25), 253505 CrossRef.
- J. Wu, W. Walukiewicz, W. Shan and K. M. Yu,
et al.
, J. Appl. Phys., 2003, 94(7), 4457 CrossRef CAS.
-
(a) Y. C. Lin, C. Y. Hsu, S. K. Hung and C. Wen, Ceram. Int., 2013, 39, 5795 CrossRef CAS;
(b) A. Purohit, S. Chander, A. Sharma, S. P. Nehra and M. S. Dhaka, Opt. Mater., 2015, 49, 51 CrossRef CAS;
(c) Z. Zhang, J. Li, H. Zhang, X. Pan and E. Xie, J. Alloys Compd., 2013, 549, 88 CrossRef CAS.
|
This journal is © The Royal Society of Chemistry and the Centre National de la Recherche Scientifique 2023 |