DOI:
10.1039/D3NA00642E
(Paper)
Nanoscale Adv., 2023,
5, 5799-5809
Oxidation engineering triggered peroxidase-like activity of VOxC for detection of dopamine and glutathione†
Received
14th August 2023
, Accepted 27th September 2023
First published on 28th September 2023
Abstract
MXenes, two-dimensional nanomaterials, are gaining traction in catalysis and biomedicine. Yet, their oxidation instability poses significant functional constraints. Gaining insight into this oxidation dynamic is pivotal for designing MXenes with tailored functionalities. Herein, we crafted VOxC nanosheets by oxidatively engineering V4C3 MXene. Interestingly, while pristine V4C3 displays pronounced antioxidant behavior, its derived VOxC showcases enhanced peroxidase-like activity, suggesting the crossover between antioxidant and pro-oxidant capability. The mixed valence states and balanced composition of V in VOxC drive the Fenton reaction through multiple pathways to continually generate hydroxyl radicals, which was proposed as the mechanism underlying the peroxidase-like activity. Furthermore, this unique activity rendered VOxC effective in dopamine and glutathione detection. These findings underscore the potential of modulating MXenes' oxidation state to elicit varied catalytic attributes, providing an avenue for the judicious design of MXenes and derivatives for bespoke applications.
1. Introduction
MXenes, two-dimensional (2D) metal carbides and nitrides, are at the forefront of research due to their potential in energy conversion, catalysis, and biomedicine.1–5 Typically, MXenes are derived by selectively etching element A from the MAX phase. The MAX phase conforms to the general formula Mn+1AXn, where M represents an early transition metal, A belongs to groups 13 or 14 of the periodic table, X is either C or N, and n can be 1, 2, or 3.6 Monolayer or few-layered MXenes possess distinctive features: (1) foremost among them is the abundance of hydrophilic functionalities such as –OH, –O, and –X (where X could be F or Cl). This not only grants MXenes excellent aqueous dispersibility but also facilitates facile chemical modifications, broadening their potential for tailored applications;7,8 (2) MXenes host an array of transition metals with mixed valences—typically between 3 and 5. This versatile valence pattern optimizes charge-carrier transfer and offers a broad spectrum of redox potentials, further enhancing MXenes' functional scope;9–12 (3) the majority of MXenes are environmentally benign and display tunable physicochemical properties that hinge upon their elemental makeup. This adaptability accentuates their potential for diverse applications while aligning with sustainability goals.13–16 Given the inherently low valence of metals in MXenes, oxidation during preparation and storage becomes inevitable. This spontaneous process can aptly be termed “auto-oxidation".17,18 Oxidation significantly alters the physical and chemical properties of MXenes, consequently impacting their functional performance in practical applications. While some studies have addressed the oxidation stability of MXenes and proposed methods to prevent it, the influence of auto-oxidation-induced transformations on MXenes' catalytic behavior remains an area warranting deeper exploration and research.19
The intrinsic susceptibility of fresh MXenes to oxidation by oxygen imparts them with remarkable antioxidant capabilities. Notably, Ti3C2 and V2C nanosheets have demonstrated a broad-spectrum ability to scavenge reactive oxygen and nitrogen species;20–22 the activity of Ti3C2 even surpasses that of conventional natural antioxidants by several folds. Given their commendable biocompatibility, MXenes like V2C, Ti3C2, and Nb2C, known for their active oxygen species (ROS)-scavenging antioxidant properties, are emerging as promising therapeutics for oxidative stress-induced conditions, such as neuronal damage and acute kidney injury.22–25 However, auto-oxidation significantly alters MXenes' redox potentials and valence compositions, potentially steering their chemical and biological activities in different directions at various oxidation phases. For instance, during Ti3C2 oxidation, the Ti(II) fraction notably diminishes, while the Ti(IV) content escalates, leading to a marked reduction in its ability to neutralize ˙OH, H2O2, and DPPH radicals.20 MXenes' uncontrollable oxidation in aqueous solutions remains a pivotal constraint for their broader application. In studying the antioxidant behavior of MXenes, we observed a gradient oxidation process, culminating in an irreversible shift from antioxidant to pro-oxidant activities. This transition can instigate unforeseen biological consequences.
In our investigation, we primarily aim to decipher the impacts of oxidation on the structural and catalytic attributes of MXenes, with V4C3 MXene spotlighted as our model. This choice is motivated by vanadium's rich valence states (+2, +3, +4, and +5) and the inherent stability of the atomic configuration. Freshly prepared V4C3 MXene nanosheets demonstrate an impressive capability to scavenge free radicals, underscoring their antioxidant prowess. Through controlled oxidation of a few-layered V4C3, we synthesized VOxC nanosheets that adopt an intricately entangled morphology. The rise of nanozymes—nanostructures mimicking enzymatic properties—has garnered significant interest due to their exceptional merits and broad application spectrum.26–28 Intriguingly, the VOxC nanosheets we prepared manifest robust peroxidase (POD)-like activity. This is attributable to the mixed valence states within the VOxC, which bolster the Fenton reaction cycle involving Vn+ species and H2O2, perpetuating the generation of hydroxyl radicals. Leveraging the antagonistic influence of biomolecules on this POD-like behavior, we devised a proficient and sensitive colorimetric assay for the detection of dopamine and glutathione.
2. Experimental section
2.1 Chemical and materials
V4AlC3 MAX powder (400 mesh) was purchased from 11 Technology, hydrogen peroxide (H2O2), horseradish peroxidase (HRP), dopamine hydrochloride, dimethyl sulfoxide (DMSO), hydrofluoric acid (HF) and 3,3′,5,5′-tetramethylbenzidine (TMB) were purchased from Sinopharm Chemical Reagent Co., Ltd. (Beijing, China) and 2,2′-Azinobis-(3-ethylbenzthiazoline-6-sulphonate) (ABTS) were commercially available from Aladdin Industrial Co (CA, USA). L-Glutathione (GSH) were purchased from Beijing OKA Biotechnology Co., LTD. Potassium persulfate (K2S2O8) were purchased from Shanghai Maclin Biochemical Technology Co., LTD. Pure terephthalic acid (PTA) were purchased from Thermo Fisher Scientific. Tetramethyl ammonium hydroxide (TMAOH) were purchased from Sigma-Aldrich (Shanghai, China). Milli-Q water (18 MΩ cm) was used in the preparation of all solutions. All glassware and autoclave used in the following procedures were cleaned by aqua regia solution (HNO3/HCl = 1
:
3 v/v).
2.2 Characterization
UV-vis absorption spectra were obtained using a Cary 60 UV-Vis Spectrometer (Varian, USA) and a matched quartz cuvette with a path length of 1 cm. The crystal structures of the V4C3 and VOxC nanosheets were characterized by X-ray diffraction (XRD, D8 Advance diffractometer, Bruker, Germany) using monochromatized Cu Kα radiation (λ = 1.5418 Å). Transmission electron microscope (TEM) and high-resolution transmission electron microscope (HRTEM) were captured on a Tecnai G2 F20 U-Twin electron microscope with an accelerating voltage of 200 kV. SEM images were obtained by FEI Field Emission Scanning Electron Microscope (FE-SEM), USA. Fourier transform infrared spectroscopy (FT-IR) were acquired by Thermo Fisher Nicolet 6700 infrared spectrometer. X-ray photoelectron spectroscopy (XPS) was performed with a Thermo ESCALAB 250XI multifunctional imaging electron spectrometer (Thermo Fisher Scientific, USA) using 150 W Al Kα radiation and a base pressure of approximately 3 × 10−9 mbar. The binding energies were calibrated to the C 1s line at 284.8 eV. Fluorescence spectral signals were acquired by a Hitachi F-4600 fluorescence spectrometer, Japin. The measurements were carried out at room temperature under the following conditions: microwave power 2 mW, modulation amplitude 1.0 G, attenuation 20 dB, and scan range of 100 G.
2.3 Synthesis of VOxC nanosheets
Preparation of multilayer V4C3 nanosheets. 1 g of V4AlC3 was added to a 100 mL Teflon tank and then 20 mL of hydrofluoric acid (HF, 40%>) was carefully added in a fume hood. After sealing the reactor, it was stirred and reacted at room temperature for 14 days to obtain a multilayer V4C3 solution in which the Al layer was etched away. The product was washed by centrifugation several times with deionized water (3500 rpm) to pH > 6. The product was dried under vacuum at 60 °C for 12 h to obtain dried V4C3 powder.
Preparation of few layers V4C3 MXene.
Firstly, 0.1 g multilayer V4C3 powder was weighed into a 10 mL glass sample bottle, and 5 mL high concentration tetramethylammonium hydroxide solution (TMAOH, 25% aqueous solution) was added, and stirred at room temperature (25 °C) for 3 days. The TMAOH solution was then centrifuged three times at 8000 rpm. The black precipitates were re-dispersed into 20 mL deionized water, and the 2D V4C3 solution was obtained by ultrasound under argon atmosphere for 90 min. The precipitation was then removed by centrifugation at 8000 rpm for 20 min and a 2D V4C3 nanosheets colloidal solution was collected. The 2D V4C3 nanosheets is dried in a vacuum freeze dryer for at least 24 h and then collected for quantification.
Preparation of VOxC nanosheets.
V4C3 nanosheets is prepared into a solution of 1 mg mL−1 and placed in a stripped sample bottle with the solution filling not exceeding 50%. The sample bottle was placed at 40 °C for 72 h until the solution was a light dark green state.
Preparation of V2O5.
The V4C3 nanosheets was freeze-dried to obtain a black light powder, which was placed in a porcelain pot and calcined in a Muffle furnace at a heating rate of 5 °C min−1 and held for 3 h. After cooling, the powder was yellow.
2.4 Evaluation of total antioxidant activity
The antioxidant capacity of nanosheets was assessed by ABTS assay.
Firstly, the stable free radical ABTS+˙ was freshly made by dissolving 0.0374 g ABTS and 0.0066 g K2S2O8 in 30 mL deionized water for 12 h in dark, and the solution was diluted by 20 times before use. The antioxidant capacity of V4C3 nanosheets at different oxidation stages was tested by UV-vis absorption spectroscopy. In typical, 10 μL V4C3 or VOxC nanosheets (0.5 mg mL−1) was added to 3 mL ABTS+˙ solution, and the absorption spectrum was recorded with two minutes interval. The scavenging rate was calculated by plotting the absorbance at 730 nm in function of reaction time.
2.5 POD-like enzyme activity and enzymatic kinetics test
The POD-like activity of VOxC nanosheets has been tested by using TMB assay.
In typical, 20 μL 20 mM TMB and 20 μL 0.1 M H2O2 were mixed in 3 mL H2O or 10 mM HAc-NaAc buffer with different pH. Then, 10 μL of V4C3 or VOxC (0.2 mg mL−1) was added to accelerate the oxidation of TMB. The UV-vis absorption spectra evolution was recorded using the scanning kinetics model with 1 min interval. The absorbance at 650 nm vs. reaction time was plotted to calculate the catalytic reaction kinetic parameters.
The apparent steady-state kinetic measurements were carried out. For TMB and H2O2 as substrate, the enzymatic kinetic parameters, Michaelis constant (Km) and maximal reaction velocity (Vmax), were calculated according to the Michaelis–Menten equation:
1/ν = Km/Vmax × (1/[S]) + 1/Vmax |
where
υ is the reaction initial velocity and [
S] is the concentration of the substrate.
2.6 Detection of dopamine and glutathione
Detection of dopamine.
The colorimetric detection of dopamine based on the concentration-dependent inhibition of VOxC POD-like activity was performed. Typically, dopamine is configured in a solution of 2 mM concentration. To ensure the accuracy of the experiment, the total solution of the sample to be tested was strictly controlled to be 3 mL. Therefore, during the test, x μL of dopamine solution was added to 2940−x μL (x = 10, 20, 30, 40, 50, 60) deionized water. Next, 20 μL of 20 mM TMB solution and 20 μL of 0.1 M H2O2 solution were added, followed by 20 μL of 0.2 mg mL−1 VOxC solution, immediately mixed for timing, and UV-vis absorption spectrum data began to be recorded when the reaction lasted for two minutes, with a monitoring range of 800–400 nm. With this method, at least three repeated tests were performed to measure different amounts of dopamine.
Detection of glutathione.
Similarly, colorimetric detection of glutathione was performed based on the concentration-dependent inhibition of POD-like activity by glutathione. Normally, glutathione is configured in a solution of 0.5 mM concentration. To ensure the accuracy of the experiment, the total solution of the sample to be tested was strictly controlled to be 3 mL. Therefore, during the test, x μL glutathione solution was added to 2940−x μL (x = 10, 20, 30, 40, 50, 60, 70, 80, 140) deionized water. Next, 20 μL of 20 mM TMB solution and 20 μL of 0.1 M H2O2 solution were added, followed by 20 μL of 0.2 mg mL−1 VOxC solution, immediately mixed for timing, and ultraviolet-visible absorption spectrum data began to be recorded when the reaction lasted for two minutes, with a monitoring range of 800–400 nm. The method was used to perform at least three repeatable tests for different levels of glutathione.
3. Results and discussion
3.1 Formation of VOxC via oxidation engineering of V4C3
As delineated in Fig. 1, we introduce a meticulously designed protocol to fabricate VOxC nanosheets. The genesis of these nanosheets stems from a systematic oxidation engineering of few-layered (FL) V4C3 MXene nanosheets under controlled conditions at 40 °C. For this endeavor, V4AlC3 MAX phase, characterized by its dense 3D layered architecture, was judiciously selected as the foundational precursor. To realize the FL-V4C3 nanosheets, we employed the well-established HF etching technique, followed by a 25% TMAOH intercalation process (Fig. S1a–c†).29 As inferred from the TEM imaging (Fig. 2a), the newly synthesized V4C3 nanosheets display dimensions ranging from 300 to 500 nm. These nascent nanosheets bear a multitude of hydrophilic moieties (–OH, –F, –O) on their surface, thereby facilitating an excellent dispersion in aqueous solutions and manifesting the quintessential Tyndall phenomena, as depicted in Fig. S2.† Recognizing the inherent propensity of the FL-V4C3 nanosheets, especially in their fresh state, towards oxidation in aqueous environments—predominantly due to the extensive presence of low-valence vanadium—we embarked on a systematic study of their oxidation dynamics.12 Consequently, we deliberately undertook the oxidation process of V4C3 under a carefully controlled condition at 40 °C. Upon exposure to a heat treatment at 40 °C for a span of 72 h, the V4C3 MXene nanosheets undergo a transformation to form their oxidation derivatives, VOxC nanosheets, as vividly depicted in Fig. 2b and S1d.† Intriguingly, these VOxC nanosheets exhibit a characteristic belt-like morphology, reminiscent of thin, delicately curled sheets, and boast an impressive span, extending beyond the 2 μm mark.
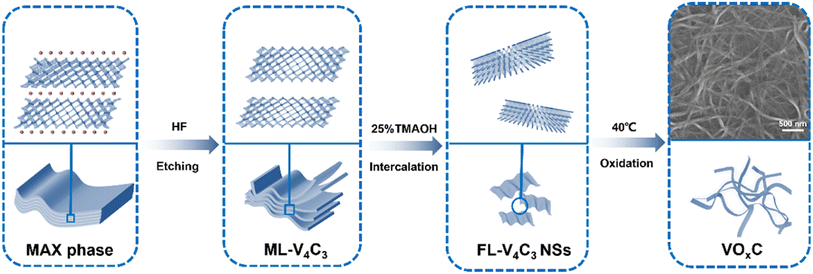 |
| Fig. 1 Schematic illustration for the synthesis and structure diagram of VOxC nanosheets. | |
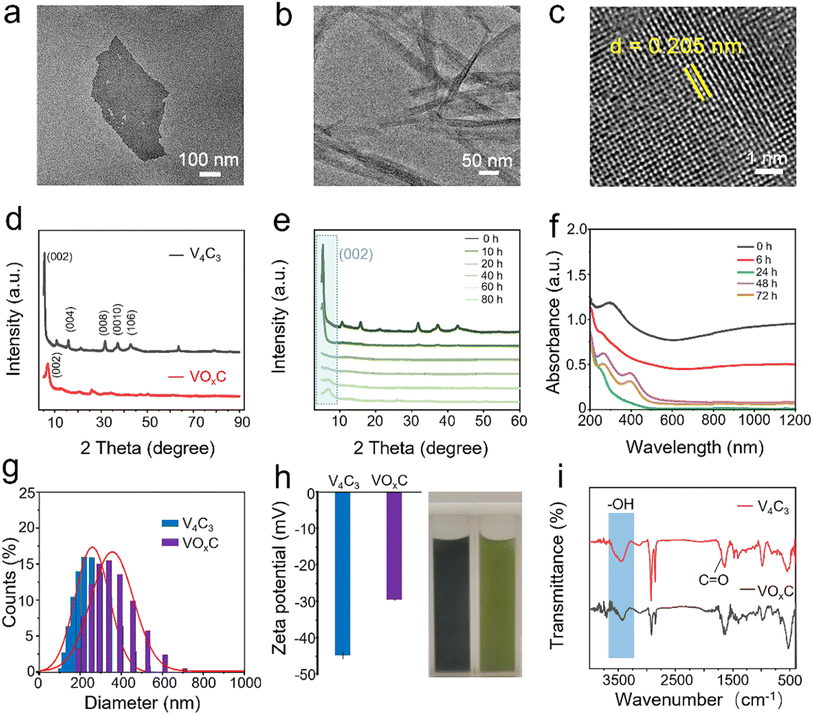 |
| Fig. 2 Characterization of V4C3 and VOxC nanosheets. (a) TEM image of fresh V4C3 nanosheets; (b) TEM and (c) HRTEM images of VOxC nanosheets after auto-oxidation treatment for 72 h at 40 °C; (d) XRD patterns of fresh V4C3 and VOxC nanosheets; (e) XRD patterns of V4C3 at different oxidation times; (f) UV-vis absorption spectra of V4C3 at different oxidation times; (g) kinetic dimension comparison of fresh V4C3 and VOxC nanosheets; (h) zeta potential comparison and optical image of fresh V4C3 and VOxC nanosheets; (i) FT-IR comparison before and after oxidation of fresh V4C3 and VOxC nanosheets. | |
The HRTEM revealed the intricate lattice fringe of VOxC. An adjacent planar separation was meticulously calculated to be 0.205 nm, corresponding to the (002) plane of VOxC, as showcased in Fig. 2c. XRD served as an instrumental technique in deciphering the crystalline structures of both V4C3 and VOxC. A noteworthy observation is the vanishing of the (004), (006), and (008) diffraction peaks of V4C3. Concurrently, the (002) diffraction peaks of V4C3 migrate to an elevated angle with a pronounced diminution in intensity as oxidation time escalates, as highlighted in Fig. 2d and e. Most strikingly, the diffraction peak of V4C3 nanosheets exhibits a steady decline and vanishes post 20 h, underscoring the hypothesis that oxidation precipitates the degradation and ensuing amorphization of V4C3 nanosheets. As oxidation ensues, the (002) diffraction peak makes a re-emergence post the 60 h mark, signifying the orchestrated re-organization and re-crystallization of VOxC. This underpins a transformative pathway for VOxC, reminiscent of a “breakage-followed-by-reconstruction” paradigm, while ensuring the resultant VOxC retains its quintessential layered MXene crystalline structure.
The dynamism of the UV-vis spectra of the V4C3 suspension, mapped across the span of oxidation at 40 °C, delineates the shifting optical properties inherent to the metamorphosis from V4C3 to VOxC. Of particular note are the emergent peaks at wavelengths 280 and 400 nm, serving as hallmarks of the VO2+ absorption band. This further corroborates the reconstruction narrative of VOxC, mirroring findings from the XRD analysis (Fig. 2f).
Furthermore, the oxidative journey begets a palpable expansion in the DLS diameter, evolving from ∼250 nm in V4C3 to ∼350 nm in VOxC (Fig. 2g). Concurrently, we witness a declination in zeta potential values, transitioning from −44.8 mV to a more subdued −29.6 mV (Fig. 2h). FT-IR was instrumental in corroborating shifts in the surface functional group composition and bond structuring pre- and post-oxidation (Fig. 2i). The spectra for VOxC are indicative of a discernible attenuation in the –OH signal juxtaposed against an escalation in the C
O signal intensity, a trend that resonates with the increased tethering of oxygen during oxidation.
Cumulatively, these revelations shed light on profound transformations in morphology, dimensions, crystallinity, surface characteristics, and optical nuances associated with V4C3 and its oxidized counterpart, VOxC. This underscores the pivotal role auto-oxidation plays in sculpting the MXene framework. Providentially, this lends an intuitive avenue to fathom the structure–activity nexus, thereby facilitating the nuanced tailoring of MXene functionalities.
Beyond the notable metamorphosis in atomic structure, V4C3's oxidation journey substantially reconfigures both its elemental composition and bond disposition. The XPS survey spectra vouch for the concurrent presence of V, C, and O across both the V4C3 and VOxC spectra, as illuminated in Fig. 3a. Intriguingly, while elemental consistency persists, a remarkable oxygen upsurge is discerned. Spectrum analysis reveals an escalation in the oxygen fraction, notably surging from 25.23% in V4C3 to 43.10% in VOxC. A deeper dive into the bonds suggests that this newly incorporated oxygen predominantly pairs with V in a V–O arrangement, as detailed in Table S1.†
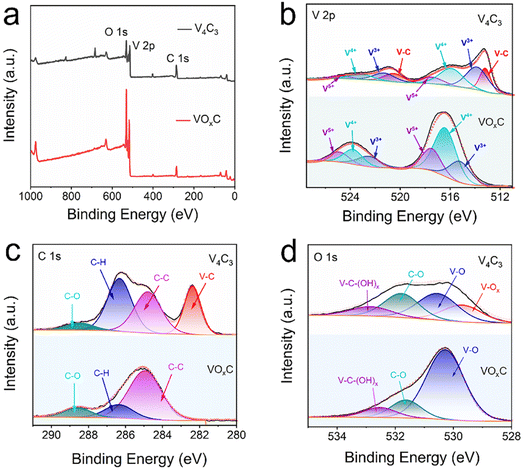 |
| Fig. 3 XPS spectra of VOxC nanosheets. (a) Survey spectra; (b–d) high-resolution fitted spectra of V 2p, O 1s, and C 1s. | |
In the meticulous V 2p high-resolution XPS spectra of V4C3 nanosheets, four distinct deconvolutional peaks emerge. These correspond to the diverse states: V–C (V2+), V3+, V4+, and V5+. The manifestation of V4+ and V5+ signals might owe their presence to incidental oxidation of V4C3 during storage and subsequent measurements. On the other hand, the discerned doublet peaks in VOxC echo the signatures of V3+, V4+, and V5+.30,31 Astoundingly, post-oxidative treatment, the V–C bond in VOxC ceases to exist, highlighting the formation of VOxC as a nuanced tapestry of mixed valence vanadium oxide, as exemplified in Fig. 3b. Diving deeper into the valence intricacies, a coexistence of multiple vanadium valences is evident in both V4C3 and VOxC. V4C3 is predominantly colored by lower valences, with V2+ and V3+ jointly accounting for approximately 60% and cumulatively reaching near 90% with V4+. In stark contrast, VOxC showcases V4+ as the dominant player at about 50%, flanked by roughly equal contributions from V3+ and V5+.
Pivoting to the C 1s spectrum of V4C3 nanosheets, the analysis discerns four Gaussian peaks, symbolizing V–C, C–C, C–H, and C–O. Herein, the V–C peak is conspicuously absent post-oxidation, accompanied by a diminution in the C–H peaks, while the C–C and C–O peaks see a significant surge in intensity, as captured in Fig. 3c. The orchestrated O 1s peaks, signifying varied oxygen bonds in V–C–(OH)x, C
O, V–O, and V–Ox, buttress the assertion of a marked augmentation in bound V oxygen, a narrative articulated in Fig. 3d.
Collectively, through mentioned above analytical techniques (TEM, XRD, XPS, etc.), the oxidation proceeding mechanism have been uncovered. With the oxidation proceeding, the morphology, size distribution, crystal structure, chemical element, and valence compositions have changed dramatically, underscoring the V4C3 nanosheets go through destruction, disintegration, and reconstruction to form VOxC nanosheets. These insights paint a compelling picture of the oxidation's transformative potential, underscoring its finesse in masterfully orchestrating the chemical and valence tapestry of MXenes.
3.2 Oxidation engineering triggered the POD-like activity of VOxC nanosheets
The auto-oxidative process introduces profound shifts in the redox potential of V4C3 nanosheets, catalyzing unanticipated biological activities. Predominantly, the antioxidant prowess emerges as a central narrative. The evolution of the antioxidant disposition of V4C3 nanosheets through the oxidation arc holds paramount significance in biological contexts. Our meticulous exploration unveiled that nascent V4C3 nanosheets exhibit a flair for ROS scavenging, underscoring their remarkable antioxidant fortitude. To distill the core of this study, we gauged antioxidant efficacy through the lens of the total antioxidant capacity (TAC), facilitated by the reduction of ABTS+·. This entity, borne from the oxidation of ABTS via K2S2O8, is a staple in TAC assays. Parallelly, the pro-oxidant mettle was mapped via its prowess in orchestrating TMB oxidation, a chromogenic substrate emblematic of POD-like activity assays. This oxidation typically manifests as a distinctive blue hue, characterized by its unique absorption spectrum. In their pristine state, V4C3 nanosheets can deftly quench ABTS+·, resonating with their antioxidant acumen, yet remain inert to catalyzing TMB oxidation in an H2O2 milieu.
However, as oxidation progresses, a captivating narrative unfolds: the once dominant antioxidant efficacy, as gauged by ABTS+· reduction, wanes progressively until it vanishes (Fig. 4a). Intriguingly, after an incubation of 40 h, the once dormant POD-like dynamism to drive TMB oxidation stirs to life, amplifying subsequently (Fig. 4a). These crescendo peaks post a 60 h oxidation interval, where the POD zeal reaches its zenith and the ABTS+· quenching capability plunges to its nadir. Fig. 4b and c vouch for the capability of VOxC in spearheading TMB oxidation in an H2O2 backdrop and a time-centric paradigm, devoid of any oxidase (OXD)-like undertones. Fig. 4a encapsulates this transformational journey, portraying the poignant intersection between antioxidant and pro-oxidant trajectories around the 40 h mark during the V4C3 nanosheet oxidation. This empirical revelation underscores the crux of our findings: V4C3 oxidation is the linchpin that pivots the sheets from their inherent antioxidant stature to a newfound pro-oxidant persona, replete with intrinsic POD-like activity.
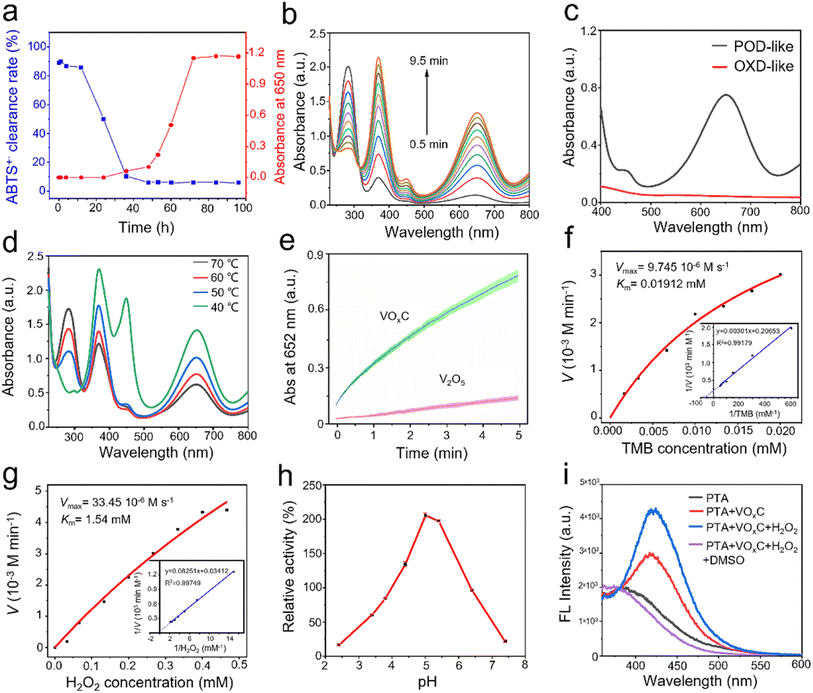 |
| Fig. 4 POD-like activity of VOxC. (a) The transformation of antioxidant and POD-like activity during the oxidation process; (b) kinetic absorption spectra of H2O2 oxidation of TMB catalyzed by VOxC; (c) comparison of TMB absorption spectra of VOxC catalytic oxidation with and without H2O2; (d) the comparison of VOxC prooxidation activities at different oxidation temperatures; (e) comparison the POD-like activity of VOxC and V2O5; (f–g) effect of TMB and H2O2 concentration on the rate of VOxC catalytic oxidation of TMB; the illustration shows the corresponding double reciprocal plots; (h) relative POD activity at different pH values; (i) fluorescence spectra of hydroxyl captured by PTA. | |
Temperature is pivotal in governing the oxidation kinetics of V4C3 and subsequently sculpting the emergence of VOxC. Indeed, it's conceivable that such variations could inflect the nuances of POD activity. To unravel this thermodynamic interplay, we embarked on a systematic investigation spanning four distinct oxidation temperatures: 40 °C, 50 °C, 60 °C, and 70 °C. Our objective was to chart the interplay between POD-like activity and the temperature trajectory.
As illustrated in Fig. 4d, VOxC, when nurtured at 40 °C, reaches the zenith of POD-like activity. However, a captivating trend emerges with escalating temperatures. Each incremental rise attenuates the POD prowess of the resulting VOxC nanosheets. To present a stark contrast, V4C3 nanosheets underwent rigorous oxidation through calcination at 500 °C in an air milieu, culminating in the formation of V2O5 nanocrystals (Fig. S3†). When juxtaposed, the POD-like dynamism of VOxC (birthed at 40 °C) conspicuously outshines, being an astounding 5.5-fold more potent than its V2O5 counterpart (Fig. 4e).
Further exploration into the catalytic nuances of VOxC led to a systematic assessment of its responses to variations in pH, as well as H2O2 and TMB concentrations, as depicted in Fig. 4f–h. Intriguingly, VOxC's optimal catalytic activity manifested at a pH of 5.0. This pH mark stands marginally elevated compared to canonical nano-peroxidases, suggesting a unique response profile for VOxC in the nano-peroxidase family.32 The concentration of H2O2 shares the same trends on affecting the catalytic rate, which is positively proportional to H2O2 and TMB concentration in the detection range. By fitting the typical double reciprocal plots (1/ν vs. 1/[S]) to the Michaelis–Menten equation, we calculated the enzyme kinetic parameters of VOxC as peroxidase using H2O2 and TMB as substrate, respectively (Fig. 4e and g). When TMB is the substrate, the Michaelis constant (Km) and maximum reaction velocity (Vmax) was calculated to be 0.01912 mM and 9.745 × 10−6 M s−1, respectively. When H2O2 is substrate, the Km and Vmax of VOxC were calculated to be 1.54 mM and 33.45 × 10−6 M s−1, respectively. This test results suggest that VOxC exhibited superior POD-like activity. To reveal the POD-like catalytic mechanism, the production of hydroxyl radicals in the presence of VOxC and hydrogen peroxide was determined by fluorescence spectroscopy. TA is selected as the fluorescent probe to ˙OH. The results showed a stronger fluorescence signal after the mixture of TA, VOxC and H2O2, and the signal could be removed by typical ˙OH scavenger DMSO, demonstrating the generation of ˙OH.
3.3 Possible mechanism for POD-like activity
Drawing from our comprehensive analysis, a conceptual trajectory detailing the metamorphosis from V4C3 to VOxC emerges (Fig. 5a). Initiated by oxidative interactions, the resilient V–C bonds are compromised, leading to a substantial alteration in the initial structural framework. As the oxidation journey continues, there's a subsequent structural renaissance marked by the reorganization and re-crystallization of the VOxC nanocrystals. This transformative sequence culminates in pronounced shifts in both morphology and chemical composition (Fig. 5b).
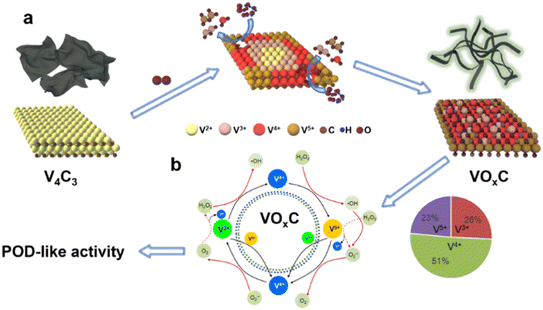 |
| Fig. 5 The schematic diagram for the (a) formation mechanism of VOxC and (b) the valence engineering driven catalytic mechanism for POD-like activity of VOxC. | |
Pivoting to the underlying mechanics of POD-like activity, we venture into the domain of valence engineering. The canonical Fenton or Fenton-like processes, underpinned by the interaction between H2O2 and specific transition metal ions (notably Fe2+ and Cu2+), have been acclaimed for their prowess in spawning hydroxyl radicals through reaction (1). Many studies are reporting the POD-like activity of nanomaterials is attributed to hydroxyl radicals' production based on Fenton reactions.33
| H2O2 + Mn+ = Mn+1 + ˙OH + HO− | (1) |
| H2O2 + 2H+ + 2e− = 2H2O (φ = +1.44 V) | (2) |
| O2+ + 2H+ + e− = VO2+ + H2O (φ = +1.00 V) | (3) |
| VO2+ + 2H+ + e− = V3+ + H2O (φ = +0.34 V) | (4) |
Vanadium complexs represent an important kind of catalysts in chemical and biological applications because of their unique electronic property.34 In our VOxC, the chemistry of vanadium is accessible with 3 adjacently mixed valence states +3 to +5 in an aqueous solution. As we calculated above, oxidation can balance the V valence compositions. The proportional composition of V3+, V4+ and V5+ in VOxC is 26%, 51% and 23%, respectively. Such mixed valence state with V4+ dominating possesses abundant electron transport behaviors. The standard redox half-reactions for H2O2/H2O, VO2+/VO2+ and VO2+/V3+ are shown above.35 Theoretically, either V3+ and V4+ can react with hydrogen peroxide to generate hydroxyl radicals based on Fenton mechanisms (eqn (5) and 6).36
| V3+ + H2O2 = VO2+ + ˙OH + H+ | (5) |
| VO2+ + H2O2 = VO2+ + ˙OH + H+ | (6) |
| VO2+ + H2O2 = VO2+ + O2˙− + H2O | (7) |
| VO2+ + HO2˙ + H+ = VO2+ + O2 + H2O | (9) |
Strikingly, the VO2+ species can be reduced back to VO2+ through three pathways, as depicted in eqn (7)–(9). These routes allow for the completion of the cycle. Owing to the presence of mixed valences, it is hypothesized that there are multiple pathways to produce hydroxyl radicals, as well as four pathways for the regeneration of VO2+ and V3+. This maintains the cycle (Fig. 5b). As a result, VOxC exhibits a highly efficient and persistent ability to generate hydroxyl radicals, demonstrating robust and sustained POD-like activity.
Notably, it is important to understand why the freshly prepared V4C3 does not exhibit POD-like activity or generate hydroxyl radicals. The freshly prepared V4C3, which has undergone minimal oxidation, mainly comprises vanadium in lower valence states. The V2+, V3+ and V4+ not only have the potential to produce hydroxyl radicals based on the Fenton mechanism, but they also act as potent reducing agents capable of reducing ˙OH and H2O. This results in complex reaction dynamics.
The rich presence of low-valence states with strong electron-donating capabilities can effectively and quickly reduce highly active ˙OH, explaining the observed phenomena. These findings highlight that the valence composition of mixed-valence vanadium is critical in regulating biological activities, such as antioxidant and pro-oxidant behaviors. In this study, we demonstrated that controlling auto-oxidation provides a potential strategy for regulating valence composition.
3.4 Detection of dopamine and glutathione
The inherent POD-like functionality of VOxC has remarkable implications not just in the realm of catalysis, but also in the domain of biological detection. Dopamine, a pivotal neurotransmitter and hormone, assumes significant interest due to its potential dysregulation, leading to a multitude of health disorders. An overexpression can trigger conditions like mania, obesity, and addictive behaviors, whereas a deficiency is often linked to Parkinson's disease and attention deficit hyperactivity disorder. Thus, the importance of accurate detection of dopamine for managing health conditions can't be overstated.
In our investigations, we discovered that the POD-like activity of VOxC could be suppressed by dopamine, consequently slowing down the oxidation of TMB. Interestingly, this inhibition was found to be dependent on the concentration of dopamine, which sets the foundation for the colorimetric detection of this neurotransmitter. We designed experiments in which the concentrations of VOxC, H2O2, and TMB were kept constant while varying the dopamine concentrations. As dopamine was introduced, we observed a decrease in absorbance at 650 nm, indicating a reduction in TMB oxidation (Fig. 6b).
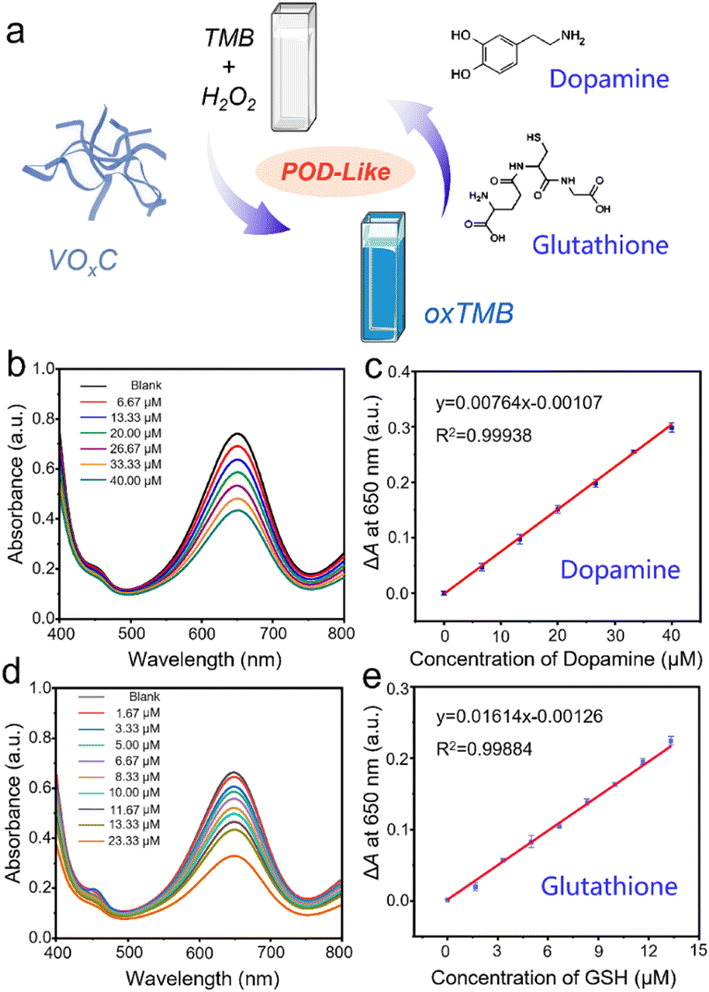 |
| Fig. 6 Detection of dopamine and glutathione by using the POD-like activity of VOxC. (a) Scheme of the inhibitory mechanism for biomolecular detection. (b) The UV-vis absorption spectrum evolution in the absence and presence of dopamine with different concentrations. (c) The dependence of ΔA at 650 nm on the concentration of dopamine. (d) The UV-vis absorption spectrum evolution in the absence and presence of glutathione with different concentrations. (e) The dependence of ΔA at 650 nm on the concentration of glutathione. | |
Following this, we plotted a response curve of A0–A (ΔA), where A0 denotes the absorbance of the control group and A represents the absorbance in the presence of varied dopamine concentrations. The plot displayed a robust linear relationship (R2 = 0.999) within the dopamine concentration range of 6.67–40 μM, with the limit of detection (LOD) for dopamine determined to be 2.5 μM (S/N = 3) (Fig. 6c). Interestingly, dopamine molecules, with their amino and phenolic hydroxyl groups, have the potential to act as electron donors, reducing H2O2 and leading to the formation of dopamine-o-quinone. This involvement of dopamine competes with TMB for H2O2 consumption, thereby reducing the amount of H2O2 involved in TMB oxidation and subsequently leading to a decrease in absorbance at 650 nm. This intriguing discovery underscores the potential utility of VOxC in dopamine detection.
Often hailed as “The Mother of All Antioxidants,” glutathione occupies a pivotal position in biological systems, instrumental in governing immune responses and determining longevity. A scarcity of this antioxidant in the body frequently correlates with numerous chronic ailments and diseases. In a manner mirroring dopamine, glutathione's potent antioxidant properties can also stifle the POD-like activity of VOxC. Seizing upon this inhibitory paradigm, we engineered a colorimetric assay for glutathione detection. Maintaining consistent concentrations of VOxC, H2O2, and TMB, we varied glutathione levels within controlled bounds. Our observations conclusively revealed that the oxidation rate of TMB is markedly suppressed by glutathione, and this suppression is strikingly concentration-dependent (Fig. 6d).
Further analysis provided a lucid view: plotting the response ΔA at 650 nm against glutathione concentrations spanning from 1.67 to 23.33 μM unveiled a tight linear correlation, as evident from an R2 value of 0.9988. This assay demonstrated an impressive LOD for glutathione, pegging it at 0.36 μM. Therefore, leveraging the unique POD-like properties of VOxC nanosheets presents an elegant and efficient route to detect crucial bio-analytes like dopamine and glutathione, further enriching the palette of analytical tools in biomedicine.
4. Conclusion
In conclusion, the POD-like activity of VOxC nanosheets triggered by oxidatively engineering V4C3 MXene was presented in this work. With the oxidation proceeding, the V4C3 nanosheets go through destruction, disintegration, and reconstruction to form VOxC nanosheets and the morphology, size distribution, crystal structure, chemical element, and valence compositions have changed dramatically. Especially, V4C3 is a non-enzymatic antioxidant that can scavenge free radicals, while the VOxC shows superior POD-like activity to generate hydroxyl radicals. The valence engineering-driven mechanism underlying the POD-like activity was proposed, the oxidation balanced the valence composition of V in VOxC that can facilitate the Fenton reaction through multiple pathways to persistently produce hydroxyl radicals. Based on the inhibitory effect on the POD-like activity of VOxC, a facile method has been developed for the detection of dopamine and glutathione. Although oxidation instability has been considered as the bottleneck challenge in MXenes development, it, fortunately, provides a facile way to delicately treat MXenes and produce various derivates with versatility. Our study may suggest a reference for exploring MXenes' oxidation chemistry and catalytic function and a full understanding of their chemical and biological activities.
Author contributions
He WW developed the concept and conceived the experiments. Jia HM and Liu Quan carried out the main experiments and wrote the manuscript. Chen YY and Zhou G performed partial experiments. Si JJ, Lan HH and He WW revised the manuscript. All the authors contributed to the data analysis and scientific discussion.
Conflicts of interest
The authors declare no conflict of interest.
Acknowledgements
This work is supported financially by National Natural Science Foundation of China (Grants 62274141, 22301258) and the National College Students' innovation and entrepreneurship training program (202210480001).
References
- Á. Morales-García, F. Calle-Vallejo and F. Illas, ACS Catal., 2020, 10, 13487–13503 CrossRef.
- L. Chen, X. Y. Dai, W. Feng and Y. Chen, Acc. Chem. Res., 2022, 3, 785–798 CAS.
- Z. M. Qiu, Y. Bai, Y. D. Gao, C. L. Liu, Y. Ru, Y. C. Pi, Y. Z. Zhang, Y. S. Luo and H. Pang, Rare Met., 2022, 41, 1101–1128 CrossRef CAS.
- Y. Cheng, L. Li, Z. Liu, S. Yan, F. Cheng, Y. Yue, S. Jia, J. Wang, Y. Gao and L. Li, Research, 2022, 2022, 9843268 CAS.
- Y. Cheng, Y. Xie, H. Cao, L. Li, Z. Liu, S. Yan, Y. Ma, W. Liu, Y. Yue, J. Wang, Y. Gao and L. Li, Chem. Eng. J., 2023, 453, 139823 CrossRef CAS.
- M. Naguib, V. N. Mochalin, M. W. Barsoum and Y. J. A. m. Gogotsi, Adv. Mater., 2014, 26, 992–1005 CrossRef CAS PubMed.
- R. M. S. Yoo and A. Djire, ACS Catal., 2023, 13, 6823–6836 CrossRef CAS.
- X. S. Lv, L. Z. Kou and T. Frauenheim, ACS Appl. Mater. Interfaces, 2021, 13, 14283–14290 CrossRef CAS PubMed.
- X. S. Sun, X. J. He, Y. Zhu, E. Obeng, B. R. Zeng, H. Deng, J. l. Shen and R. D. Hu, Chem. Eng. J., 2023, 451, 138985 CrossRef CAS.
- Z. L. Tan, J. X. Wei, Y. Liu, F. u. Zaman, W. Rehman, L. R. Hou and C. Z. Yuan, Rare Met., 2022, 41, 775–797 CrossRef CAS.
- Y. Cheng, Y. Xie, Z. Liu, S. Yan, Y. Ma, Y. Yue, J. Wang, Y. Gao and L. Li, ACS Nano, 2023, 17, 1393–1402 CrossRef CAS PubMed.
- Y. Cheng, Y. Xie, Y. Ma, M. Wang, Y. Zhang, Z. Liu, S. Yan, N. Ma, M. Liu, Y. Yue, J. Wang and L. Li, Nano Energy, 2023, 107, 108131 CrossRef CAS.
- H. Huang, C. h. Dong, W. Feng, Y. Wang, B. c. Huang and Y. Chen, Adv. Drug Delivery Rev., 2022, 184, 114178 CrossRef CAS PubMed.
- J. L. Wu, Y. Y. Yu and G. X. Su, Nanomaterials, 2022, 12, 828 CrossRef CAS PubMed.
- Y. Cheng, Y. Xie, S. Yan, Z. Liu, Y. Ma, Y. Yue, J. Wang, Y. Gao and L. Li, Sci. Bull., 2022, 67, 2216–2224 CrossRef CAS PubMed.
- L. Li, Y. Cheng, H. Cao, Z. Liang, Z. Liu, S. Yan, L. Li, S. Jia, J. Wang and Y. Gao, Nano Energy, 2022, 95, 106986 CrossRef CAS.
- R. A. Soomro, P. Zhang, B. M. Fan, Y. Wei and B. Xu, Nano-Micro Lett, 2023, 15, 108 CrossRef CAS PubMed.
- F. C. Cao, Y. Zhang, H.
Q. Wang, K. Khan, A. K. Tareen, W. Qian, H. Zhang and H. Ågren, Adv. Mater., 2022, 34, 2107554 CrossRef CAS PubMed.
- X. F. Zhao, A. Vashisth, E. Prehn, W. M. Sun, S. A. Shah, T. Habib, Y. Chen, Z. Tan, J. L. Lutkenhaus and M. Radovic, Green Matter., 2019, 1, 513–526 CrossRef.
- H. Q. Geng, Y. P. Ren, G. Qin, T. Wen, Q. Liu, H. Y. Xu and W. W. He, RSC Adv., 2022, 12, 11128–11138 RSC.
- Y. Li, R. Z. Fu, Z. G. Duan, C. H. Zhu and D. D. Fan, ACS Nano, 2022, 16, 7486–7502 CrossRef CAS PubMed.
- W. Feng, X. G. Han, H. Hu, M. Q. Chang, L. Ding, H. J. Xiang, Y. Chen and Y. H. Li, Nat. Commun., 2021, 12, 2203 CrossRef CAS PubMed.
- X. Zhao, L. Y. Wang, J. M. Li, L. M. Peng, C. Y. Tang, X. J. Zha, K. Ke, M. B. Yang, B. H. Su and W. Yang, Adv. Sci., 2021, 8, 2101498 CrossRef CAS PubMed.
- C. J. Du, W. Feng, X. Y. Dai, J. H. Wang, D. Y. Geng, X. D. Li, Y. Chen and J. Zhang, Small, 2022, 18, 2203031 CrossRef CAS PubMed.
- X. Y. Ren, M. F. Huo, M. M. Wang, H. Lin, X. X. Zhang, J. Yin, Y. Chen and H. H. Chen, ACS Nano, 2019, 13, 6438–6454 CrossRef CAS PubMed.
- R. F. Zhang, X. Y. Yan and K. L. Fan, Acc. Chem. Res., 2021, 2, 534–547 CAS.
- H. Wei, L. Z. Gao, K. L. Fan, J. W. Liu, J. Y. He, X. G. Qu, S. J. Dong, E. K. Wang and X. Y. Yan, Nano Today, 2021, 40, 101269 CrossRef CAS.
- Q. Yang, Y. Y. Mao, Q. Liu and W. W. He, Rare Met., 2023, 42, 2928–2948 CrossRef CAS.
- Y. Wei, P. Zhang, R. A. Soomro, Q. Z. Zhu and B. Xu, Adv. Mater., 2021, 33, 2103148 CrossRef CAS PubMed.
- X. Wang, S. Lin, H. Y. Tong, Y. N. Huang, P. Tong, B. C. Zhao, J. M. Dai, C. H. Liang, H. Wang, X. B. Zhu, Y. P. Sun and S. X. Dou, Electrochim. Acta, 2019, 307, 414–421 CrossRef CAS.
- Y. Liu, Y. Jiang, Z. Hu, J. Peng, W. H. Lai, D. L. Wu, S. W. Zuo, J. Zhang, B. Chen, Z. W. Dai, Y. G. Yang, Y. Huang, W. Zhang, W. Zhao, W. Zhang, L. Wang and S. L. Chou, Adv. Funct. Mater., 2020, 31, 2008033 CrossRef.
- L. Z. Gao, J. Zhuang, L. Nie, J. B. Zhang, Y. Zhang, N. Gu, T. H. Wang, J. Feng, D. L. Yang, S. Perrett and X. Yan, Nat. Nanotechnol., 2007, 2, 577–583 CrossRef CAS PubMed.
- H. J. Dong, W. Du, J. Dong, R. C. Che, F. Kong, W. L. Cheng, M. Ma, N. Gu and Y. Zhang, Nat. Commun., 2022, 13, 5365 CrossRef CAS PubMed.
- R. R. Langeslay, D. M. Kaphan, C. L. Marshall, P. C. Stair, A. P. Sattelberger and M. Delferro, Chem. Rev., 2019, 119, 2128–2191 CrossRef CAS PubMed.
-
F. A. Cotton, G. Wilkinson, C. A. Murillo and M. Bochmann, Adv. Inorg. Chem., John Wiley and Sons, Inc., 1999 Search PubMed.
- G. D. Du and J. H. Espenson, Inorg. Chem., 2005, 44, 5514–5522 CrossRef CAS PubMed.
Footnote |
† Electronic supplementary information (ESI) available: Elemental atomic percentages of V4C3 and VOxC calculated by XPS fitted spectra (Table S1); SEM image of MAX phase V4AlC3, multilayered V4C3, few layered V4C3 NSs and VOxC (Fig. S1); dindar optical effect of V4C3 nanosheets dilute solution under laser pointer irradiation (Fig. S2); XRD pattern of V2O5 after calcination of V4C3 nanosheets (Fig. S3). See DOI: https://doi.org/10.1039/d3na00642e |
|
This journal is © The Royal Society of Chemistry 2023 |
Click here to see how this site uses Cookies. View our privacy policy here.