DOI:
10.1039/D3MD00290J
(Research Article)
RSC Med. Chem., 2023,
14, 1712-1721
Discovery of benzoxazole–thiazolidinone hybrids as promising antibacterial agents against Staphylococcus aureus and Enterococcus species†
Received
23rd June 2023
, Accepted 17th July 2023
First published on 27th July 2023
Abstract
Antibiotic resistance is rapidly exacerbating the unceasing rise in nosocomial infections caused by drug-resistant bacterial pathogens such as methicillin-resistant Staphylococcus aureus (MRSA), carbapenem-resistant Enterobacteriaceae (CRE) and vancomycin-resistant Enterococcus (VRE). Therefore, there is a dire need for new therapeutic agents that can mitigate the unbridled emergence of drug-resistant pathogens. In the present study, several benzoxazole–thiazolidinone hybrids (BT hybrids) were synthesized and evaluated for their antibacterial activity against the ESKAP pathogen panel. The preliminary screening revealed the selective and potent inhibitory activity of hydroxy BT hybrids against S. aureus with MIC ≤ 4 μg mL−1. Hydroxy compounds (BT25, BT26, BT18, BT12, and BT11) exhibited a good selectivity index (SI > 20), which were determined to be non-toxic to Vero cells. An engaging fact is that two compounds BT25 and BT26 showed potent activity against various clinically-relevant and highly drug resistant S. aureus (MRSA & VRSA) and Enterococcus (VRE) isolates. These hybrids showed concentration-dependent bactericidal activity that is comparable to vancomycin. These experimental results were corroborated with docking, molecular dynamics, and free energy studies to discern the antibacterial mechanisms of hydroxy BT hybrids with three bacterial enzymes DNA gyrase B, MurB, and penicillin binding protein 4 (PBP4). The reassuring outcome of the current investigation confirmed that the aforementioned BT hybrids could be used as very promisingly potent antibacterial agents for the treatment of Staphylococcus aureus and Enterococcus infections.
1. Introduction
The World Health Organization (WHO) has affirmed that that in the year preceding the COVID-19 pandemic, more than 1.27 million patients succumbed to a plethora of serious bacterial infections attributable to antimicrobial resistance (AMR) world-wide. The emerging AMR has become a matter of grave concern as a consequence of excessive and unregulated usage of antibiotics.1–3 Treating contagious infections with antibiotics is becoming a great challenge due to the escalation of AMR in human bacterial pathogens.4 Divergent surgeries and chemotherapies in hospitals worldwide are facing serious challenges without efficacious antibiotics arising due to AMR for the prevention and treatment of consequent infections in these patients.5 This has resulted in a global threat emerging out of AMR with concomitant upsurge in hospitalization, medical and recouping costs.6 AMR associated with pathogens including Escherichia coli, Staphylococcus aureus, Klebsiella pneumoniae, Streptococcus pneumoniae, Acinetobacter baumannii, and Pseudomonas aeruginosa was cumulatively associated with ∼3.57 million deaths in 2019.7 During the same year, methicillin-resistant S. aureus (MRSA) was one of the pathogen–drug combinations that was responsible for greater than 100
000 infections, entailing losses in the United States and Europe to the tune of a staggering total $57–70 billion and more than €1.5 billion annually.5 Based on the extensive studies and analysis, it has been prognosticated that if adequate preventative care and remedial measures are impaired, nearly 10 million people would die every year due to antimicrobial resistance by 2050.8 To circumvent these risks to global health, unprecedented research efforts are being made to combat the hazard of AMR.
A large class of naturally occurring and synthesized compounds with a wide range of biological activity share the benzoxazole ring as their fundamental structural component.9,10 A number of benzoxazole-based compounds have also demonstrated remarkable antibacterial activity. Examples of benzoxazole antibiotics include calcimycin (known for its antibiotic and ionophoric properties),11 boxazomycins (active against Gram-positive bacteria and anaerobes)12 and caboxamycin (an antibiotic produced by deep-sea Streptomyces sp. NTK 937).13 The thiazolidinone core, on the other hand, has piqued the curiosity of researchers due to its diverse degrees of pharmacological and therapeutic activities.14 The potential of the thiazolidinone moiety was demonstrated by successful introduction of etozoline as an antihypertensive, ralitoline as a powerful anti-convulsant, pioglitazone as a hypoglycemic agent and thiazolidomycin as an antibacterial agent against Streptomyces sp. As a consequence of all the aforementioned aspects including the earlier results.15,16 We describe herewith synthesis and biological evaluation of new hybrid derivatives by integrating both benzoxazole and 4-thiazolidinone moieties in one structural framework.
2. Experimental
2.1 General methods
All reagents and solvents were procured from SD Fine and Sigma-Aldrich chemicals. The 1HNMR and 13CNMR spectra were obtained on the Bruker Ascend 400 MHz spectrophotometer using tetramethyl silane (TMS) as the internal standard and dimethyl sulfoxide (DMSO-d6) as the solvent. Mass spectra were recorded on Agilent Technologies 6550 iFunnel LC-MS Q-TOF with APCI and ESI methods. IR spectra were obtained on a PerkinElmer FT-IR C114728 spectrophotometer with an ATR accessory. UV-vis spectra for all compounds were obtained on a Cary 60 UV-vis spectrophotometer. The melting points were recorded in the Stuart Melting Point Apparatus SMP30.
Synthesis of BT1 (parent BT hybrid).
Anhydrous NaOAc (0.631 g, 0.007 mol) was added to a magnetically stirred mixture of 2-thioureidobenzoxazole (1.158 g, 0.006 mol) and chloroacetic acid (0.728 g, 0.007 mol) in 15 mL of ethanol, and the mixture was heated at reflux for 24 hours. Following the reaction, the precipitate (1.291 g, 90%) was collected and re-crystallized from ethyl acetate as slender off-white needles; M.P. 255–257 °C; λmax(THF): 325 nm; νmax (cm−1): 3149 (N–H, thiazolidinone), 3090 (C–H aromatic), 1703 (C
O, thiazolidinone), 1570 (C
N); 1HNMR (400 MHz, DMSO-d6): δ 12.54 (s, 1H, amide), 7.62 (m, 2H, benzoxazole), 7.30 (m, 2H, benzoxazole), 4.11 (s, 2H, S–![[C with combining low line]](https://www.rsc.org/images/entities/char_0043_0332.gif)
2–CO, thiazolidinone); 13C NMR (100 MHz, DMSO-d6): δ 173.79, 170.41, 162.04, 147.43, 140.70, 123.70, 123.30, 117.75, 109.53, 34.84; mass (m/z): 234.0334 [M + H+].
Synthesis of aryl BT hybrids.
In a 25 mL RBF, a mixture of parent BT hybrid (BT1) (0.002 mol), corresponding aldehyde (0.003 mol), and the base catalyst, anhydrous NaOAc (0.004 mol) in glacial acetic acid (15 mL) was refluxed overnight. After the completion of the reaction, the precipitated solid was collected through filtration, dried and recrystallized from THF or EtOH–DMF mix depending on the solubility of the synthesized compound.
BT2: yellow solid; yield: 70%; M.P. 319–321 °C; λmax(THF): 365 nm; νmax (cm−1): 3119 (N–H lactam), 3056 (C–H aromatic), 1693 (C
O thiazolidinone), 1567 (C
N); 1HNMR (400 MHz, DMSO-d6): δ 13.18 (s, 1H, amide), 7.83 (s, 1H, ethenyl), 7.73 (m, 1H, benzoxazole), 7.72 (d, J = 7.2 Hz, 2H, aryl), 7.65 (m, 1H, benzoxazole), 7.61 (t, J = 7.2 Hz, 1H, aryl), 7.53 (t, J = 7.2 Hz, 1H, aryl), 7.34 (m, 2H, benzoxazole); 13C NMR (101 MHz, DMSO-d6) δ 167.13, 163.11, 161.93, 148.28, 141.23, 133.24, 133.14, 130.67, 130.28 (2C), 129.48 (2C), 124.59, 124.32, 123.77, 118.70, 110.36; Mass (m/z): 321.9832 [M + H+].
The characterization details of the remaining compounds (BT3–BT26) are described in the ESI.† The nomenclature of the synthesized compounds is provided in Table 1.
Table 1 Nomenclature of synthesized BT hybrids
BT hybrid |
Aryl group |
BT hybrid |
Aryl group |
BT hybrid |
Aryl group |
BT1
|
— |
BT10
|
|
BT19
|
|
BT2
|
|
BT11
|
|
BT20
|
|
BT3
|
|
BT12
|
|
BT21
|
|
BT4
|
|
BT13
|
|
BT22
|
|
BT5
|
|
BT14
|
|
BT23
|
|
BT6
|
|
BT15
|
|
BT24
|
|
BT7
|
|
BT16
|
|
BT25
|
|
BT8
|
|
BT17
|
|
BT26
|
|
BT9
|
|
BT18
|
|
|
|
2.2
In vitro antibacterial studies
2.2.1 Growth media and reagents.
All bacterial media and supplements including Mueller–Hinton cation supplemented broth II (MHBII), Mueller–Hinton agar (MHA) and Tryptic soy broth (TSB) were purchased from Becton-Dickinson (Franklin Lakes, NJ, USA). All other chemicals and antibiotics were procured from Sigma-Aldrich (St. Louis, MO, USA). Roswell Park Memorial Institute medium (RPMI) and fetal bovine serum (FBS) were purchased from Lonza (Lonza, USA). All methods were performed in accordance with the relevant guidelines and regulations.
2.2.2 Bacterial strains.
The molecules were screened against a bacterial panel consisting of ESKAPE pathogens, namely Escherichia coli (ATCC 25922), Staphylococcus aureus (ATCC 29213), Klebsiella pneumoniae (BAA-1705), Acinetobacter baumannii (BAA-1605), Pseudomonas aeruginosa (ATCC 27853) and Enterococcus sp. The panel was further expanded to include drug-resistant clinical S. aureus and Enterococcus strains including those resistant to vancomycin and other clinically utilized antibiotics. These strains were procured from Biodefense and Emerging Infections Research Resources Repository/Network on Antimicrobial Resistance in Staphylococcus aureus/American Type Culture Collection (BEI/NARSA/ATCC, USA) and routinely cultivated on MHA and MHBII. Before starting the experiment, a single colony was picked from MHA plate, inoculated in MHBII and incubated overnight at 37 °C with shaking for 18–24 h to get the starter culture.
2.2.3 Antibiotic susceptibility testing.
Antibiotic susceptibility testing of hits was conducted according to the CLSI guidelines using the broth microdilution assay. 10 mg mL−1 stock solutions of test compounds were prepared in DMSO. Bacterial cultures were inoculated in MHBII and optical density (OD) was measured at 600 nm, followed by dilution to achieve ∼106 CFU mL−1. The compounds were tested from 64–0.5 mg L−1 in two-fold serial diluted fashion with 2.5 μL of each concentration added to well of a 96-well round bottom microtiter plate. Later, 97.5 μL of bacterial suspension was added to each well containing either test compound or appropriate controls. The plates were incubated at 37 °C for 18–24 h following which the MIC was determined. The MIC is defined as the lowest concentration of the compound at which there is absence of visible growth. For each test compound, MIC determinations were carried out independently three times using duplicate samples.
2.2.4 Cell cytotoxicity against Vero cells (ATCC CCL-81).
Cell toxicity was performed against Vero cells using the MTT assay. ∼103 cells per well were seeded in 96 well plate and incubated at 37 °C in an 5% CO2 atmosphere. After 24 h, compound was added ranging from 100–12.5 μg mL−1 concentration and incubated for 72 h. After the incubation was over, MTT was added in each well, incubated at 37 °C for further 4 h, residual medium was discarded, 0.1 mL of DMSO was added to solubilise the formazan crystals and OD was taken at 540 nm for the calculation of CC50. CC50 is defined as the lowest concentration of compound which leads to a 50% reduction in cell viability. Doxorubicin was used as positive control and each experiment was repeated in triplicate.
2.2.5 Bacterial time kill kinetics.
The presence or absence of bactericidal activity was assessed by the time-kill method as described earlier. Briefly, S. aureus ATCC 29213 were diluted ∼106 CFU mL−1 in MHBII and treated with 1× and 10× MIC of compounds and vancomycin and incubated at 37 °C with shaking for 24 h. 100 μL samples were collected at 0, 1, 6 and 24 h, serially diluted in PBS and plated on MHA followed by incubation at 37 °C for 18–20 h. The kill curves were constructed by counting colonies from plates and plotting the CFU mL−1 of surviving bacteria at each time point in the presence and absence of compound. Each experiment was repeated three times in duplicate and the mean data were plotted.
3. Results and discussion
3.1 Chemistry
A series of benzoxazole–thiazolidinone hybrids bearing different aryl groups at the fifth position on the thiazolidinone ring was synthesized using a simple method as outlined in Scheme 1. Initially, isoperthiocyanic acid (IPA) was prepared from the reaction between ammonium thiocyanate and conc. HCl by following the Klason method.17 As previously reported, good yields of 2-thioureidobenzoxazole (BOT) were obtained by the reaction of IPA with 2-aminophenol in absolute ethanol.18 In the presence of anhydrous sodium acetate, chloroacetic acid reacts with BOT as a cyclizing agent to generate 4-thiazolidinone ring linked to benzoxazole moiety with an imine linkage (BT1). Subsequent Knoevenagel type condensation of BT1 with various aromatic aldehydes in acetic acid afforded the BT hybrids (BT2–BT26). The synthesized compounds were characterized by IR, 1H/13C-NMR and mass spectral studies. From the IR spectra, the presence of CO–NH (thiazolidinone) group is inferred by the occurrence of absorption bands at 1725–1680 cm−1 whereas the absorption bands at 3110–3162 cm−1, 1496–1452 cm−1, 3150–2860 cm−1 and 1602–1570 cm−1 corresponds to the presence of N–H, C
C, C–H and C
N group, respectively. In the instance of 1H-NMR, the signals of protons associated with amide groups (thiazolidinone, CO–NH) of aryl BT hybrids were detected at a lower magnetic field (>13 ppm) than their counterpart in parent BT hybrid (BT1). However, in the 13C-NMR spectra, the signals of the carbonyl carbon (174.4 ppm) of aryl hybrids were more shielded than that of the parent hybrid (∼166 ppm). Depending on the aryl group or heterocycle substitutions, the ethenyl protons of the aryl BT hybrids appeared at 7.7 to 8.6 ppm.
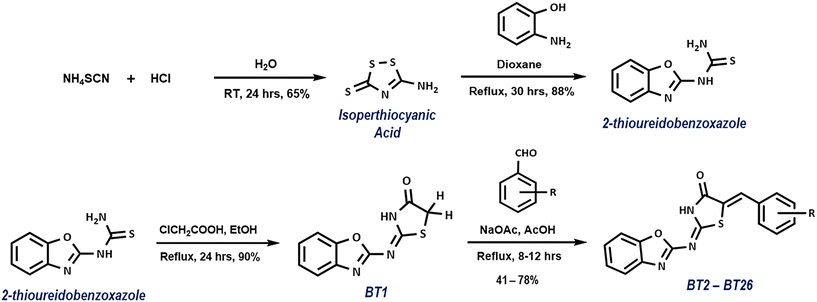 |
| Scheme 1 General synthetic strategy for the synthesis of BT hybrids | |
3.2
In vitro antibacterial studies
The synthesized BT hybrids (1–26) were initially screened against a panel of bacterial strains including E. coli ATCC 25922, S. aureus ATCC 29213, K. pneumoniae BAA 1705, A. baumannii BAA 1605 and P. aeruginosa ATCC 27853. Levofloxacin was utilized as positive control and the results are displayed in Table 2.
Table 2 Minimum inhibitory concentration (MIC) (μg mL−1) of BT hybrids against bacterial pathogen panel
Compound |
MIC (μg mL−1) |
E. coli ATCC 25922 |
S. aureus ATCC 29213 |
K. pneumoniae BAA 1705 |
A. baumannii BAA 1605 |
P. aeruginosa ATCC 27853 |
BT1
|
>64 |
8
|
>64 |
>64 |
>64 |
BT2
|
>64 |
4
|
>64 |
>64 |
>64 |
BT3
|
>64 |
16
|
>64 |
>64 |
>64 |
BT4
|
>64 |
8
|
>64 |
>64 |
>64 |
BT5
|
>64 |
8
|
>64 |
>64 |
>64 |
BT6
|
>64 |
16
|
>64 |
>64 |
>64 |
BT7
|
>64 |
8
|
>64 |
>64 |
>64 |
BT8
|
>64 |
8
|
>64 |
>64 |
>64 |
BT9
|
>64 |
32
|
>64 |
>64 |
>64 |
BT10
|
>64 |
4
|
>64 |
>64 |
>64 |
BT11
|
>64 |
2
|
>64 |
>64 |
>64 |
BT12
|
>64 |
2
|
>64 |
>64 |
>64 |
BT13
|
>64 |
8
|
>64 |
>64 |
>64 |
BT14
|
>64 |
>64 |
>64 |
>64 |
>64 |
BT15
|
>64 |
>64 |
>64 |
>64 |
>64 |
BT16
|
>64 |
>64 |
>64 |
>64 |
>64 |
BT17
|
>64 |
>64 |
>64 |
>64 |
>64 |
BT18
|
>64 |
2
|
>64 |
>64 |
>64 |
BT19
|
>64 |
4
|
>64 |
>64 |
>64 |
BT20
|
>64 |
>64 |
>64 |
>64 |
>64 |
BT21
|
>64 |
32
|
>64 |
>64 |
>64 |
BT22
|
>64 |
16
|
>64 |
>64 |
>64 |
BT23
|
>64 |
8
|
>64 |
>64 |
>64 |
BT24
|
>64 |
8
|
>64 |
>64 |
>64 |
BT25
|
>64 |
1
|
>64 |
>64 |
>64 |
BT26
|
>64 |
1
|
>64 |
>64 |
>64 |
Levofloxacin
|
0.0156
|
0.125
|
64
|
8
|
1
|
The results of antibacterial activity indicated that BT hybrids were inactive against all tested bacterial strains (MIC > 64 μg mL−1) with exception of S. aureus ATCC 29213. With MIC ranging from 1–32 μg mL−1, 21 of the 26 synthesized derivatives demonstrated moderate to strong activity against S. aureus ATCC 29213. The dimethylamino-, methoxy-, and nitro-groups containing compounds made the remaining 6 compounds that were likewise resistant to S. aureus ATCC 29213. The parent BT hybrid (BT1) had a moderate level of activity (8 μg mL−1) and its benzaldehyde derivative (BT2, with no substitutions on aryl group) had a relatively high level of activity (4 μg mL−1), demonstrating that 5-arylidene derivatives had an antibacterial efficacy significantly greater than that of the parent thiazolidinone.
The activity, however, diminished or became comparable to the parent BT hybrid when the aryl group was replaced with alternative heterocycles (thiophene – 16 μg mL−1, furan – 8 μg mL−1 & pyridine – 8 μg mL−1). The compounds with chloro and flouro-substitutions on aryl group (B3 & BT6, MIC – 16 μg mL−1; BT4 & BT7, MIC – 8 μg mL−1; BT5 & BT8, MIC – 8 μg mL−1) demonstrated the same activity regardless of their chemical behavior. Hydroxy substitution at 2′, 3′ or 4′ positions on aryl group exhibited remarkable potency. The strongest and the best activity was shown by hydroxyl derivatives of BT hybrids (BT10, BT11, BT12, BT18, BT19, BT25 and BT26; MIC ≤ 4 μg mL−1).
More interestingly, eight-fold increased activity was observed in BT25 and BT26, when the aryl group was replaced by the halo-salicyl group on BT hybrids. Both these compounds have the highest activity of all the compounds tested, with a MIC 1 μg mL−1. The notable antibacterial activity of these compounds against the tested microbes spurred further investigation on them. The active hydroxy BT hybrids with MIC ≤ 2 μg mL−1 against S. aureus ATCC 29213, are displayed in Fig. 1.
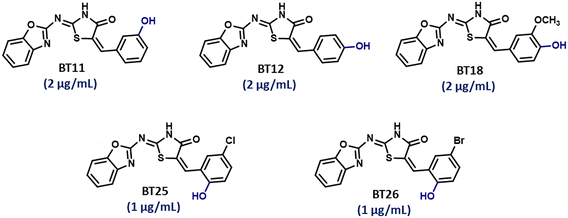 |
| Fig. 1 The hydroxy BT hybrids which exhibited potent activity against S. aureus ATCC 29213 in the preliminary screening. | |
The in vitro cytotoxicity of active compounds (MIC ≤ 2 μg mL−1) against Vero cells was then evaluated using the MTT assay in order to determine the selectivity index (SI) of the active compounds. For a compound to move forward for pre-clinical development, an SI of greater than 10 is regarded as necessary. As shown in Table 3, all of the selected compounds had an impressive SI > 20, thus affirming their specificity towards bacterial rather than host cells.
Table 3 Cytotoxicity profile and SI of selected BT hybrids against Vero cells
Compound |
MIC (μg mL−1) against S. aureus ATCC 29213 |
Cell cytotoxicity CC50 (μg mL−1) |
Selectivity index (SI) |
CC50: 50% cytotoxic concentration (μg mL−1). |
BT26
|
1 |
>20 |
>20 |
BT25
|
1 |
>20 |
>20 |
BT18
|
2 |
>40 |
>20 |
BT12
|
2 |
>40 |
>20 |
BT11
|
2 |
>40 |
>20 |
Doxorubicin
|
— |
10 |
ND |
Levofloxacin
|
0.125 |
>100 |
>800 |
As the selected BT hybrids possess a high SI, they were further tested for their antibacterial activity potential against a variety of clinically-relevant S. aureus and Enterococcus isolates along with levofloxacin, minocycline, meropenem and vancomycin as comparators. These clinical strains have a wide range of resistance patterns, including those that are resistant to vancomycin and other commonly used antibiotics (Table 1, ESI†). The MIC values of active BT hybrids against the clinical drug-resistant isolates are tabulated in Tables 4 and 5.
Table 4 Minimum inhibitory concentration (MIC) (μg mL−1) of selected BT hybrids against clinically relevant multidrug-resistant MRSA and VRSA isolates
Strain |
MIC (μg mL−1) |
BT26
|
BT25
|
BT18
|
BT12
|
BT11
|
LEVO
|
MERO
|
VANC
|
LEVO (levofloxacin), MERO (meropenem), VANC (vancomycin); bold font is used to denote the MIC of most active hybrids. |
S. aureus ATCC 29213 |
1 |
1 |
4 |
2 |
2 |
0.25 |
0.125 |
1 |
MRSA
|
NRS 100 |
0.5
|
0.5
|
2 |
1 |
1 |
0.25 |
32 |
2 |
NRS 119 |
1 |
1 |
4 |
2 |
2 |
16 |
64 |
2 |
NRS 129 |
0.5
|
0.5
|
4 |
2 |
2 |
0.25 |
4 |
1 |
NRS 186 |
0.5
|
0.5
|
2 |
1 |
1 |
8 |
8 |
1 |
NRS 191 |
0.5
|
0.5
|
2 |
2 |
2 |
16 |
64 |
1 |
NRS 192 |
1 |
1 |
4 |
2 |
2 |
8 |
8 |
1 |
NRS 193 |
1 |
1 |
4 |
2 |
2 |
32 |
64 |
2 |
NRS 194 |
1 |
1 |
4 |
2 |
2 |
0.125 |
1 |
1 |
NRS 198 |
1 |
1 |
4 |
2 |
2 |
32 |
64 |
2 |
VRSA
|
VRS 1 |
1 |
1 |
4 |
2 |
2 |
64 |
64 |
>64 |
VRS 4 |
1 |
1 |
4 |
2 |
2 |
>64 |
64 |
>64 |
VRS 12 |
0.5
|
0.5
|
2 |
2 |
2 |
32 |
8 |
>64 |
Table 5 Minimum inhibitory concentration (MIC) (μg mL−1) of selected BT hybrids against VSE and VRE strains
Strain |
MIC (μg mL−1) |
BT26
|
BT25
|
BT18
|
BT12
|
BT11
|
LEVO
|
MINO
|
VANC
|
LEVO (levofloxacin), MINO (minocycline), VANC (vancomycin); bold font is used to denote the MIC of most active hybrids. |
VSE
|
E. faecalis NR 31884 |
4 |
4 |
16 |
16 |
16 |
0.25 |
0.5 |
2 |
E. faecalis NR 31885 |
4 |
4 |
16 |
16 |
16 |
0.25 |
0.5 |
2 |
E. faecalis NR 31886 |
4 |
4 |
16 |
16 |
16 |
0.25 |
0.5 |
2 |
E. faecalis NR 31887 |
2 |
2 |
16 |
8 |
8 |
0.25 |
8 |
2 |
E. faecalis NR 31888 |
4 |
4 |
16 |
16 |
16 |
0.25 |
0.5 |
2 |
VRE
|
E. faecium NR 31903 |
1 |
1 |
4 |
4 |
4 |
>64 |
8 |
>64 |
E. faecium NR 31909 |
0.125
|
0.125
|
0.25
|
0.25
|
0.25
|
64 |
8 |
>64 |
E. faecium NR 31912 |
0.125
|
0.125
|
0.25
|
0.25
|
0.25
|
64 |
8 |
>64 |
It was found that BT25 and BT26 showed the same potency (MIC 0.5–1 μg mL−1) against various MRSA and VRSA strains, as shown in Table 4. BT25 and BT26 typically had a fourfold increase in activity compared to BT18 but they displayed a twofold increase in activity when compared with BT11 and BT12. Comparing MIC for various strains of MRSA and VRSA to those for BT25 and BT26, vancomycin, levofloxacin, and meropenem demonstrated a broad range of MIC values. Thus, it may be concluded that drug-resistance mechanisms have no effect on the antibacterial efficacy of BT25 and BT26.
Vancomycin-resistant Enterococci (VRE) are typically associated with serious multidrug-resistant (MDR) persistent infections.19,20 In this scenario, the development of prospective therapeutic candidates is crucial and urgent for the treatment of MDR Enterococci infections. As anticipated, it was discovered that BT25 and BT26, with MIC of 0.125 μg mL−1, were the most potent molecules at inhibiting two VRE strains, E. faecium NR 31909 and E. faecium NR 31912 as displayed in Table 5. Moreover, they exhibited considerable potency against VSE strains (MIC ≤ 4 μg mL−1). On the other hand, B11, B12, and BT18 also showed potency against the chosen bacterial strains, albeit with reduced activity than BT25 and BT26 in a comparable manner. Overall, the selected BT hybrids showed medium effectiveness against VSE but were more potent against VRE, which is encouraging for their potential to be active against strains that are MDR.
To ascertain whether the reported antibacterial activity was bacteriostatic or bactericidal in nature, the time killing kinetics activity of BT25 and BT26 with S. aureus ATCC 29213 was evaluated and compared with vancomycin (Fig. 2). Although in certain instances, it is advantageous to have drugs with bacteriostatic activity to manage microbes, bactericidal antibiotics are often preferred since they have a faster rate of infection clearance and a reduced chance of developing drug resistance.21 The current investigation demonstrates the bactericidal activity of both BT25 and BT26 at 10× MIC is on par with vancomycin. Overall, the selected BT hybrids showed concentration-dependent bactericidal activity that is comparable to vancomycin.
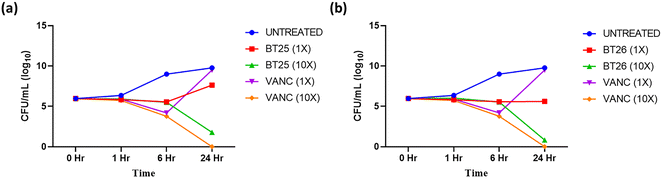 |
| Fig. 2 Time-kill kinetics plots of BT25 (a) and BT26 (b) against S. aureus ATCC 29213 compared with vancomycin (VANC). The experiment was repeated twice in duplicate and the curves are representing the mean. | |
3.3
In silico studies
The hydroxy BT hybrids exhibited excellent efficacy against some of the bacterial strains in in vitro experiments. To gain more insights into the antibacterial mechanisms of these compounds, each of them and the parent BT-hybrid were taken into consideration for the in silico investigations. Due to their broad spectrum biological activities, benzoxazoles, benzthiazoles, and benzimidazoles serve as essential fragments in medicinal chemistry.22 Benzthiazoles and benzimidazoles were described as DNA gyrase B inhibitors in the literature.23–26 Due to its structural resemblance to other benzheterazoles, benzoxazole may be categorized as a DNA gyrase B inhibitor. On the other hand, the function of substituted 4-thiazolidinones and their derivatives as MurB inhibitors has garnered a great deal of attention.27–29E. coli MurB was inhibited by trisubstituted 4-thiazolidinones at low micromolar concentrations, likely functioning as diphosphate substitutes for the native substrate.30 Furthermore, the related imidazolidinone displayed potent antibacterial activity and inhibited the MurB enzyme.31 In addition, thiazolidine ring is the structural basis of penicillin nucleus of the penicillin antibiotics. Penicillin analogues bind to bacterial proteins called penicillin-binding proteins (PBPs) which play crucial roles in the synthesis of bacterial cell walls because they are engaged in the biosynthesis of peptidoglycan.32
To discern insights into the antibacterial mechanisms of hydroxy BT hybrids, molecular docking and molecular dynamics simulations were performed on the crystal structures of the bacterial enzymes DNA gyrase B, MurB, and Penicillin binding protein 4 (PBP4). Through molecular docking, the promising interactions of hydroxy BT hybrids with bacterial enzymes were discovered. The molecular dynamics simulations showed that the bacterial complexes exhibited moderate to high stability. The key interactions of BT25, BT26 and reference drugs at the binding sites of the three enzymes are shown in Fig. 3. The crucial finding from these simulations was that the hydroxyl group of the hydroxy BT hybrids was perpetually involved in the hydrogen bonding interactions with the key residues of the bacterial enzymes.
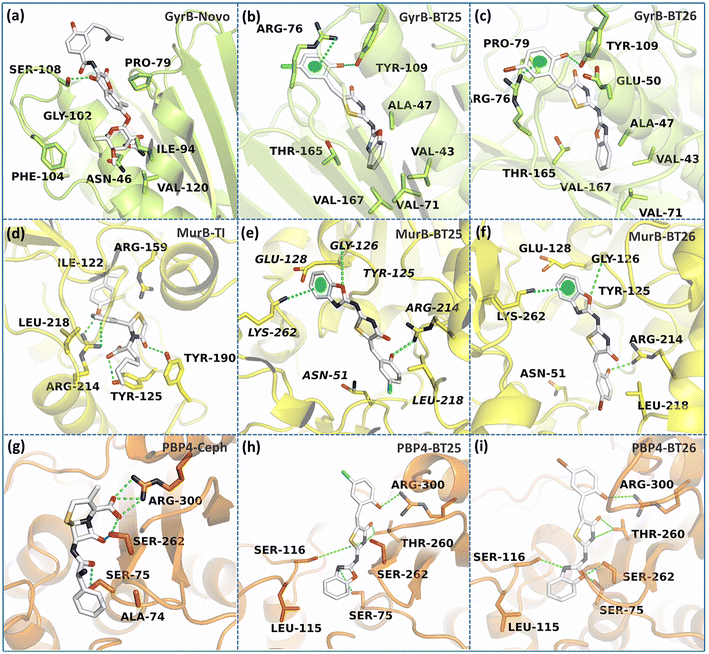 |
| Fig. 3 3D interaction plots of BT25, BT26 and reference drugs with DNA gyrase B (green), MurB (yellow) and PBP4 (light brown). Two main interactions between the BT hybrids and the selected proteins are: hydrogen bonding interactions (cyan dotted lines) and π-cation interactions (green dotted lines). The molecular interactions of BT25, BT26, and reference drugs are shown with DNA gyrase B in (a), (b) and (c), with MurB in (d), (e) and (f), and with PBP4 in (g), (h) and (i) respectively. | |
For instance, the hydroxyl group actively interacted with Asp73, Tyr109, Ser10 and Thr165 in the case of DNA gyrase B; Glu128, Arg159, Arg214, and Gln288 in the case of MurB; Glu297 and Arg300 in the case of PBP4. This could delineate the rationale for high inhibitory activities exhibited by hydroxy BT hybrids. The results and discussions pertaining to molecular docking and molecular dynamics simulations are provided in the supplementary information.
From the MD simulations, it is very evident that the PBP4 systems exhibited relatively high stability. It's noteworthy to note that both BT25 and BT26 exhibited a maximum of 9 and 7 hydrogen bonds during the simulation comparable to cephalexin (7), respectively. Furthermore, the binding free energy calculations revealed that BT26 displayed high binding free energy (−25.15 kcal mol−1) than the reference drug cephalexin (−10.15 kcal mol−1) towards PBP4. Since the hydroxy BT hybrids demonstrated more affinity for PBP4 than the other two enzymes, it could be the possible target for the BT hybrids for their antibacterial activity.
4. Conclusion
Designing and discovering new drug candidates are indispensable and sine quo non in the drug development process. To address the various challenges that the present drug development process encounters, the concept of hybrid molecules was developed.33 Two or more pharmacologically active compounds are amalgamated into hybrid molecules with an appropriate linker to integrate the properties or functions of each moiety into a single chemical entity.34 In the current study, two such pharmacologically active scaffolds were combined to create benzoxazole–thiazolidinone hybrids. A relatively simple four-step procedure was employed to synthesize the proposed BT hybrids and they were characterized using various spectroscopic methods. The BT hybrids were then screened for their antibacterial activity against a panel of pathogens consisting of S. aureus, E. coli, A. baumannii, K. pneuminiae and P. aeruginosa. Among all the synthesized compounds, hydroxy BT hybrids demonstrated selective and potent inhibitory activity against S. aureus, with MIC ranging from 1–4 μg mL−1. Different substituents at various positions on the aryl group on the 5th position of the thiazolidinone ring exhibited varying antibacterial activity and it has been proposed that functional groups and their positions are also crucial determinants of antibacterial activity. The in vitro cytotoxicity studies of active compounds (MIC ≤ 2 μg mL−1) against Vero cells revealed that these hybrids were non-toxic to Vero cells with a selectivity index > 20. Further, the potency of BT25 and BT26 against different MRSA and VRSA strains was found to be the same (MIC 0.5–1 μg mL−1). Furthermore, the compounds BT25 and BT26 were found to selectively inhibit VRE strains (E. faecium NR 31909 and NR 31912) with a MIC of 0.125 μg mL−1, when screened against clinical VSE and VRE isolates. These experimental results were substantiated with docking, molecular dynamics, and free energy studies to discern the antibacterial mechanisms of hydroxy BT hybrids with three bacterial enzymes DNA gyrase B, MurB, and penicillin binding protein 4 (PBP4). The outcome of our investigations has affirmed that hydroxy BT hybrids can be very promising antibacterial agents for treating Staphylococcus and Enterococcus infections.
Author contributions
VSK: methodology, investigation, visualization, software, data curation, formal analysis, writing – original draft. AA, DS and RM: investigation. SC: methodology, supervision, writing – reviewing & editing. SCN: conceptualization, supervision, writing – reviewing and editing.
Conflicts of interest
There are no conflicts of interest to declare.
Acknowledgements
CNS and VSK are grateful to Bhagwan Sri Sathya Sai Baba, Founder Chancellor of Sri Sathya Sai Institute of Higher Learning, for His constant support and inspiration. VSK acknowledge the characterization facilities at CRIF, PSN Campus, SSSIHL. VSK appreciate Sri Prashant Rai, Sri Nitesh Tamang, and Sri Manohar Bhujel, Department of Chemistry, PSN Campus, SSSIHL, for their assistance in obtaining the spectral data. VSK expresses gratitude to Sri M G Nandagopal, Librarian (Hon.), BRN Campus, SSSIHL for his help in providing the computational resources for MD simulations. VSK thanks Dr. K Aravind Kumar, Senior Engineer at Materials & Structural Analysis Division of Thermo Fisher Scientific, USA, for his valuable inputs. VSK thanks Dr. Swayamsiddha Kar, Dept. of Chemistry, BRN campus, SSSIHL for facilitating the anti-bacterial studies. VSK thanks SSSIHL for the financial support. AA and DS thank UGC whereas RM thanks DST INSPIRE for their fellowships.
References
- A. G. Tóth, M. F. Judge, S. Á. Nagy, M. Papp and N. Solymosi, A survey on antimicrobial resistance genes of frequently used probiotic bacteria, 1901 to 2022, Eurosurveillance, 2023, 28 DOI:10.2807/1560-7917.ES.2023.28.14.2200272.
- I. Elibe Mba and E. Innocent Nweze, Antimicrobial Peptides Therapy: An Emerging Alternative for Treating Drug-Resistant Bacteria, Yale J. Biol. Med., 2022, 95, 445–463 Search PubMed.
- J. A. Ayukekbong, M. Ntemgwa and A. N. Atabe, The threat of antimicrobial resistance in developing countries: Causes and control strategies, Antimicrob. Resist. Infect. Control, 2017, 6, 1–8, DOI:10.1186/s13756-017-0208-x.
- B. Aslam, W. Wang, M. I. Arshad, M. Khurshid, S. Muzammil, M. H. Rasool, M. A. Nisar, R. F. Alvi, M. A. Aslam, M. U. Qamar, M. K. F. Salamat and Z. Baloch, Antibiotic resistance: a rundown of a global crisis, Infect. Drug Resist., 2018, 11, 1645–1658, DOI:10.2147/IDR.S173867.
- J. M. A. Blair, M. A. Webber, A. J. Baylay, D. O. Ogbolu and L. J. V. Piddock, Molecular mechanisms of antibiotic resistance, Nat. Rev. Microbiol., 2015, 13, 42–51, DOI:10.1038/nrmicro3380.
- X. Wittebole, S. De Roock and S. M. Opal, A historical overview of bacteriophage therapy as an alternative to antibiotics for the treatment of bacterial pathogens, Virulence, 2014, 5, 226–235, DOI:10.4161/viru.25991.
- T. Ueda, Y. Takesue, K. Nakajima, K. Ichiki, K. Ishikawa, K. Yamada, T. Tsuchida, N. Otani, Y. Takahashi, M. Ishihara, S. Takubo, K. Iijima, H. Ikeuchi, M. Uchino and T. Kimura, Correlation between Antimicrobial Resistance and the Hospital-Wide Diverse Use of Broad-Spectrum Antibiotics by the Antimicrobial Stewardship Program in Japan, Pharmaceutics, 2023, 15 DOI:10.3390/pharmaceutics15020518.
- M. E. A. de Kraker, A. J. Stewardson and S. Harbarth, Will 10 Million People Die a Year due to Antimicrobial Resistance by 2050?, PLoS Med., 2016, 13, 1–6, DOI:10.1371/journal.pmed.1002184.
-
S. Pal, B. Manjunath, S. Ghorai and S. Sasmal, Benzoxazole Alkaloids: Occurrence, Chemistry, and Biology, Elsevier Inc., 1st edn, 2018, DOI:10.1016/bs.alkal.2017.12.002.
- S. Singh, G. Veeraswamy, D. Bhattarai, J. Il Goo, K. Lee and Y. Choi, Recent Advances in the Development of Pharmacologically Active Compounds that Contain a Benzoxazole Scaffold, Asian, J. Org. Chem., 2015, 4, 1338–1361, DOI:10.1002/ajoc.201500235.
- D. A. Evans, C. E. Sacks, W. A. Kleschick and T. R. Taber, Polyether Antibiotics Synthesis. Total Synthesis and Absolute Configuration of the Ionophore A-23187, J. Am. Chem. Soc., 1979, 101, 6789–6791, DOI:10.1021/ja00516a069.
- T. Kusumi, T. Ooi, M. R. Wülchli and H. Kakisawa, Structure of the novel antibiotics boxazomycins A, B, and C, J. Am. Chem. Soc., 1988, 110, 2954–2958, DOI:10.1021/ja00217a043.
- C. Hohmann, K. Schneider, C. Bruntner, E. Irran, G. Nicholson, A. T. Bull, A. L. Jones, R. Brown, J. E. M. Stach, M. Goodfellow, W. Beil, M. Krämer, J. F. Imhoff, R. D. Süssmuth and H. P. Fiedler, Caboxamycin, a new antibiotic of the benzoxazole family produced by the deep-sea strain Streptomyces sp. NTK 937, J. Antibiot., 2009, 62, 99–104, DOI:10.1038/ja.2008.24.
- A. C. Tripathi, S. J. Gupta, G. N. Fatima, P. K. Sonar, A. Verma and S. K. Saraf, 4-Thiazolidinones: The advances continue, Eur. J. Med. Chem., 2014, 72, 52–77, DOI:10.1016/j.ejmech.2013.11.017.
- P. Vicini, A. Geronikaki, M. Incerti, F. Zani, J. Dearden and M. Hewitt, 2-Heteroarylimino-5-benzylidene-4-thiazolidinones analogues of 2-thiazolylimino-5-benzylidene-4-thiazolidinones with antimicrobial activity: Synthesis and structure-activity relationship, Bioorg. Med. Chem., 2008, 16, 3714–3724, DOI:10.1016/j.bmc.2008.02.001.
- V. S. Krishna Cheerala, P. Ghanta and S. C. Neelakantan, Design, synthesis and in silico screening of benzoxazole– thiazolidinone hybrids as potential inhibitors of SARS-CoV-2 proteases, RSC Adv., 2021, 11, 39328–39342, 10.1039/d1ra07504g.
- R. Seltzer and W. J. Considine, Basicity of isoperthiocyanic acid, J. Org. Chem., 1970, 35, 1665–1666, DOI:10.1021/jo00830a087.
- N. R. Krishnaswamy, C. N. Sundaresan, P. N. K. Nambisan and K. Sandya, 5-Amino-3H-1, 2, 4-Dithiazole-3-Thione as a Synthon: New Synthesis of 2-thioureidobenzheteroazoles, Heteroat. Chem., 1994, 5, 567–569 CrossRef CAS.
- F. Lebreton, W. van Schaik, A. M. McGuire, P. Godfrey, A. Griggs, V. Mazumdar, J. Corander, L. Cheng, S. Saif, S. Young, Q. Zeng, J. Wortman, B. Birren, R. J. L. Willems, A. M. Earl and M. S. Gilmore, Emergence of epidemic multidrug-resistant Enterococcus faecium from animal and commensal strains, MBio, 2013, 4 DOI:10.1128/mBio.00534-13.
- Y. Cetinkaya, P. Falk and C. G. Mayhall, Vancomycin-Resistant Enterococci, Clin. Microbiol. Rev., 2000, 13, 686–707, DOI:10.1128/CMR.13.4.686.
- K. Y. Rhee and D. F. Gardiner, Clinical relevance of bacteriostatic versus bactericidal activity in the treatment of gram-positive bacterial infections [2], Clin. Infect. Dis., 2004, 39, 755–756, DOI:10.1086/422881.
- D. Seenaiah, P. R. Reddy, G. M. Reddy, A. Padmaja, V. Padmavathi and N. Siva Krishna, Synthesis, antimicrobial and cytotoxic activities of pyrimidinyl benzoxazole, benzothiazole and benzimidazole, Eur. J. Med. Chem., 2014, 77, 1–7, DOI:10.1016/j.ejmech.2014.02.050.
- Ž. Skok, M. Barančoková, O. Benek, C. D. Cruz, P. Tammela, T. Tomašič, N. Zidar, L. P. Mašič, A. Zega, C. E. M. Stevenson, J. E. A. Mundy, D. M. Lawson, A. Maxwell, D. Kikelj and J. Ilaš, Exploring the Chemical Space of Benzothiazole-Based DNA Gyrase B Inhibitors, ACS Med. Chem. Lett., 2020, 11, 2433–2440, DOI:10.1021/acsmedchemlett.0c00416.
- M. Gjorgjieva, T. Tomašič, M. Barančokova, S. Katsamakas, J. Ilaš, P. Tammela, L. P. Mašič and D. Kikelj, Discovery of Benzothiazole Scaffold-Based DNA Gyrase B Inhibitors, J. Med. Chem., 2016, 59, 8941–8954, DOI:10.1021/acs.jmedchem.6b00864.
- M. Barančoková, D. Kikelj and J. Ilaš, Recent progress in the discovery and development of DNA gyrase B inhibitors, Future, Med. Chem., 2018, 10, 1207–1227, DOI:10.4155/fmc-2017-0257.
- S. N. Dighe and T. A. Collet, Recent advances in DNA gyrase-targeted antimicrobial agents, Eur. J. Med. Chem., 2020, 199, 112326, DOI:10.1016/j.ejmech.2020.112326.
- M. Fesatidou, P. Zagaliotis, C. Camoutsis, A. Petrou, P. Eleftheriou, C. Tratrat, M. Haroun, A. Geronikaki, A. Ciric and M. Sokovic, 5-Adamantan thiadiazole-based thiazolidinones as antimicrobial agents. Design, synthesis, molecular docking and evaluation, Bioorg. Med. Chem., 2018, 26, 4664–4676, DOI:10.1016/j.bmc.2018.08.004.
- S. Cascioferro, B. Parrino, D. Carbone, D. Schillaci, E. Giovannetti, G. Cirrincione and P. Diana, Thiazoles, Their Benzofused
Systems, and Thiazolidinone Derivatives: Versatile and Promising Tools to Combat Antibiotic Resistance, J. Med. Chem., 2020, 63, 7923–7956, DOI:10.1021/acs.jmedchem.9b01245.
- C. Tratrat, A. Petrou, A. Geronikaki, M. Ivanov, M. Kostić, M. Soković, I. S. Vizirianakis, N. F. Theodoroula and M. Haroun, Thiazolidin-4-Ones as Potential Antimicrobial Agents: Experimental and In Silico Evaluation, Molecules, 2022, 27, 1930, DOI:10.3390/molecules27061930.
- C. J. Andres, J. J. Bronson, S. V. D'Andrea, M. S. Deshpande, P. J. Falk, K. A. Grant-Young, W. E. Harte, H. T. Ho, P. F. Misco, J. G. Robertson, D. Stock, Y. Sun and A. W. Walsh, 4-Thiazolidinones: Novel inhibitors of the bacterial enzyme MurB, Bioorganic Med, Chem. Lett., 2000, 10, 715–717, DOI:10.1016/S0960-894X(00)00073-1.
- J. J. Bronson, K. L. DenBleyker, P. J. Falk, R. A. Mate, H. T. Ho, M. J. Pucci and L. B. Snyder, Discovery of the first antibacterial small molecule inhibitors of MurB, Bioorg. Med. Chem. Lett., 2003, 13, 873–875, DOI:10.1016/S0960-894X(02)01076-4.
- M. A. Welsh, A. Taguchi, K. Schaefer, D. Van Tyne, F. Lebreton, M. S. Gilmore, D. Kahne and S. Walker, Identification of a Functionally Unique Family of Penicillin-Binding Proteins, J. Am. Chem. Soc., 2017, 139, 17727–17730, DOI:10.1021/jacs.7b10170.
-
M. Decker, Design of Hybrid Molecules for Drug Development, Elsevier, Würzburg, 2017 Search PubMed.
- S. Choudhary, P. K. Singh, H. Verma, H. Singh and O. Silakari, Success stories of natural product-based hybrid molecules for multi-factorial diseases, Eur. J. Med. Chem., 2018, 151, 62–97, DOI:10.1016/j.ejmech.2018.03.057.
Footnote |
† Electronic supplementary information (ESI) available: The ESI contains: characterization details; 1H and 13C NMR spectra of BT hybrids; mass spectra of BT hybrids; in silico studies; molecular details of the bacterial strains. See DOI: https://doi.org/10.1039/d3md00290j |
|
This journal is © The Royal Society of Chemistry 2023 |