DOI:
10.1039/D3MD00044C
(Research Article)
RSC Med. Chem., 2023,
14, 1698-1703
Enhancing allosteric inhibition of dihydrodipicolinate synthase through the design and synthesis of novel dimeric compounds†
Received
28th January 2023
, Accepted 1st July 2023
First published on 25th July 2023
Abstract
The synthesis of the first dimeric inhibitor of E. coli dihydrodipicolinate synthase (DHDPS) is reported herein. Inspired by 2,4-thiazolidinedione based ligands previously shown to inhibit DHDPS, a series of dimeric inhibitors were designed and synthesised, incorporating various alkyl chain bridges between two 2,4-thiazolidinedione moieties. Aiming to exploit the multimeric nature of this enzyme and enhance potency, a dimeric compound with a single methylene bridge achieved the desired outcome with low micromolar inhibition of E. coli DHDPS observed. This work highlights the continued importance of investigation into DHDPS as an antibacterial target. Furthermore, we demonstrate the design of dimeric ligands can provide a promising strategy to improve potency in the search for novel bioactive compounds.
Introduction
With the majority of oligomeric proteins existing as homodimers,1 the design of dimeric inhibitors that can interact with more than one monomer of a multivalent protein is a compelling approach to the development of new therapeutics. By binding to two sites simultaneously, a dimeric ligand can increase both potency and selectivity toward the target for a single ligand.2 This strategy has been explored in the development of treatments for influenza,3 the synthesis of anti-cancer agents4,5 and also for central nervous system therapeutics.6
Dihydrodipicolinate synthase (DHDPS) is a key enzyme of the diaminopimelate pathway. It predominately exists as a tetramer comprising of four identical monomeric units and is responsible for the production of lysine and its immediate precursor meso-DAP.7,8 As an essential enzyme in both bacteria and plants, DHDPS has attracted much interest as an enzyme target for the development of new antibiotics and herbicides.9–13 Despite many efforts in mimicking natural substrates of the pathway including pyruvate, (S)-aspartate semialdehyde (ASA), 2,3-dihydrodipicolinate (DHDP), 4-hydroxy-2,3,4,5-tetrahydro-(2S)-dipicolinic acid (HTPA), 2,3,4,5-tetrahydrodipicolinate (THDP) and (S)-lysine14–25 it wasn't until the discovery of R,R-bislysine (Fig. 1) that potent sub-micromolar inhibition of DHDPS was observed.26 Not only did R,R-bislysine demonstrate improved binding affinity in comparison to a single (S)-lysine ligand but also confirmed that DHDPS is susceptible to inhibition across a dimeric interface.
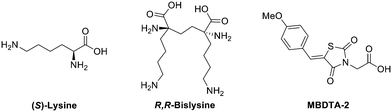 |
| Fig. 1 Natural allosteric inhibitor (S)-lysine, C. jejuni DHDPS inhibitor R,R-bislysine and previously synthesised E. coli and A. thaliana DHDPS, inhibitor MBDTA-2.26–30 | |
Recently we reported the discovery and synthesis of a series of 2,4-thiazolidinediones and analogous heterocycles as potent inhibitors of both bacterial and plant DHDPS. 2,4-Thiazolidinedione MBDTA-2 (Fig. 1) was found to have an IC50 value of 47.0 ± 2.3 μM against bacterial E. coli DHDPS and inhibited plant A. thaliana DHDPS1 and DHDPS2 with IC50 values of 66.2 ± 7.6 μM and 66.0 ± 11.0 μM respectively.27–29 We later demonstrated MBDTA-2 is the first inhibitor of the diaminopimelate pathway with herbicidal activity and validating DHDPS as a viable target for the development of agrichemicals.30 Herein, we expand upon our previous work to describe the synthesis of a series of novel dimeric 2,4-thiazolidinedione compounds based on MBDTA-2, with the intent to exploit the multimeric nature of DHDPS to generate an improved inhibitor of DHDPS.
Results and discussion
Design and synthesis of dimeric compounds
Based on X-ray data of the previously synthesised MBDTA-2 co-crystallised with A. thaliana DHDPS, we observed binding of two ligands in close proximity at the tight dimer interface (Fig. 2).29 It was anticipated that the binding mode for an inhibitor to the A. thaliana protein would be similar to the E. coli protein as both have a tetrameric quaternary structure consisting of a “dimer of dimers”, with the only difference being the configuration of the dimers for the plant structure (“back to back”) versus the bacterial structure (“head to head”). We hypothesised a short linker between two inhibiting molecules of MBDTA-2 would potentially allow allosteric binding across the dimeric interface and enhance DHPDS inhibition in comparison to the binding of a single ligand. It was therefore decided to produce dimers of 2,4-thiazolidinedione MBDTA-2 with methylene, ethylene and propylene bridges between the carboxylic acid moieties.
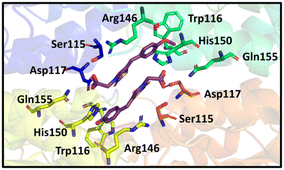 |
| Fig. 2 Binding of two MBTA-2 ligands with DHDPS.29 | |
To begin the synthesis of the proposed MBDTA-2 dimer with a methylene bridge, diethyl 2,4-dibromopentanedioate 1 (Scheme 1) was produced from glutaric acid as previously reported by Guanti and Riva.31 Subsequent alkylation of this diester with 2.1 equivalents of 2,4-thiazolidinedione in the presence of potassium carbonate under reflux conditions afforded a mixture of diastereoisomers 2. It was observed that there was no need for separation of diastereomers at this point as Knoevenagel condensation using a single diastereoisomer with 4-methoxybenzaldehyde would result in racemic product mixture regardless. It should also be noted that the presence of a small amount of diethyl 2-bromopentanedioate in the starting material of this reaction resulted in the formation of an unwanted product which was isolated by column chromatography and confirmed as the monosubstituted compound 3 (Scheme 2).
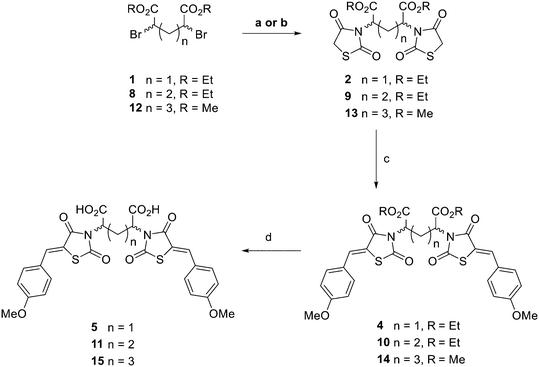 |
| Scheme 1 Synthetic route to afford one, two and three carbon bridge linked compounds. Reagents and conditions: (a) 2,4-thiazolidinedione, K2CO3, ACN, reflux 2–4 h, 36–39%; (b) KHCO3, DMF, rt 3 days, 51%; (c) 4-methoxybenzaldehyde, piperidine, AcOH, PhCH3, reflux 18 h, 24–70%; (d) conc. HCl : AcOH (1 : 2), reflux 2 h, 26–85%. | |
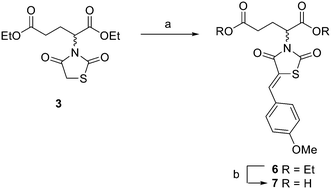 |
| Scheme 2 Synthesis of extended analogue of MBDTA-2. Reagents and conditions: (a) 4-methoxybenzaldehyde, piperidine, AcOH, PhCH3, reflux 18 h, 68%; (b) conc. HCl : AcOH (1 : 2), reflux 2 h, 78%. | |
Knoevenagel condensation of compound 2 with 4-methoxybenzaldehyde was conducted in the presence of piperidine and acetic acid in toluene. Column chromatography resulted in the elution of two compounds with the same molecular mass, presumably the rac and meso compounds. Proton NMR spectroscopy of both compounds gave, unsurprisingly, similar spectra except for the doublet of doublets (dd) signals rising from the methylene bridge. The spectra of the 4a had this signal at 4.93 ppm while the same signal was downfield at 5.08 ppm for 4b (ESI† data).
Acid hydrolysis of diester 4a by reflux in concentrated hydrochloric acid and glacial acetic acid afforded the desired final diacid product 5a in 68% yield. Singles crystals of 5a were grown from chloroform/diethyl ether, which were high quality and allowed for a satisfactory structural solution and refinement by X-ray crystallography. Compound 5a was confirmed as the rac mixture, revealing an “open” structure with the two thiazolidine-2,4-dione groups extended in anti configuration relative to the pentanedioic acid moiety and as shown by the R,R enantiomer depicted in Fig. 3. Compound 4b was also subjected to the same hydrolysis conditions to afford the diacid 5b in 74% yield. X-ray crystallography indicated 4b to be the expected meso compound with a “closed” structure with the two thiazolidine-2,4-dione groups extended in syn configuration relative to the pentanedioic acid moiety (ESI† Fig. S1). Notable differences in the methylene protons were also observed in the proton NMR spectra for the diastereomers. Racemic isomers of the diacid product 5a saw splitting of the methylene protons into two separate environments meanwhile the methylene protons were isochronous for the meso isomer 5b. A similar phenomenon was reported by Krasnov et al. in the synthesis of adipic and glutaric acid stereoisomers.32
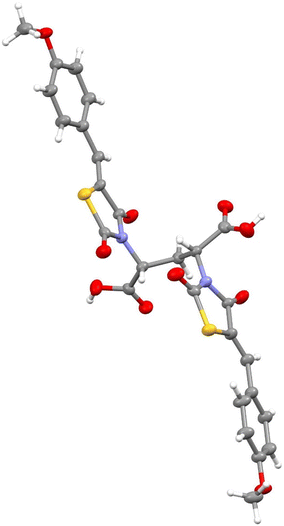 |
| Fig. 3 Crystal structure of compound 5a indicating an “open” configuration and rac stereochemistry with the R,R enantiomer shown. | |
In addition to the planned synthesis of the methylene bridged dimeric compound, the monosubstituted compound 3 was also reacted by Knoevenagel condensation and acid hydrolysis to afford compound 7 (Scheme 2) as an extended analogue of MBDTA-2 for biological testing.
To synthesise an ethylene bridge between dimeric ligands, the commercially available diethyl meso-2,5-dibromoadipate 8 was coupled with 2,4-thiazolidinedione to afford 9 as a mixture of stereoisomers. Knoevenagel condensation of the mixture with 4-methoxybenzaldehyde resulted in the synthesis of compound 10. Trituration of this product with small amounts of methanol afforded a solid as compound 10a which was found by proton NMR spectroscopy to have a doublet of doublets methylene signal at 4.89 ppm and was indicated to be the meso isomer via X-ray crystallography (ESI† Fig. S2). It was noted that the <5 ppm proton NMR methylene signal was indicative of the formation of an “open” crystal structure, consistent with the observations made for compound 4a. As expected, hydrolysis of meso diester 10a resulted in the corresponding diacid product 11a.
The methanolic solution, from which the meso compound 10a had been removed by trituration, was found to contain mostly the racemate 10b as a mixture of R,R and S,S enantiomers but also a small amount of compound 10a. Compound 10b was found to have a doublet of doublets methylene signal at 5.02 ppm by proton NMR spectroscopy. This material was not purified any further but hydrolysed to give the desired diacid compound 11, with the major product isolated by semi-preparative RP-HPLC as compound 11b and indicated to be the rac mixture with a “closed” structure which was assigned as the S,S enantiomer (ESI† Fig. S3). As observed for compounds 5a and 5b respectively, splitting of the methylene protons into two separate environments occurred for racemic compound 11b while the methylene protons were isochronous for the meso compound 11a. In summary, both isomers of the ethylene bridged 2,4-thiazolidinedione dimers were thus synthesised and characterised alongside both of the methylene bridged, 4-thiazolidinedione dimers in preparation for biological testing (Fig. 4).
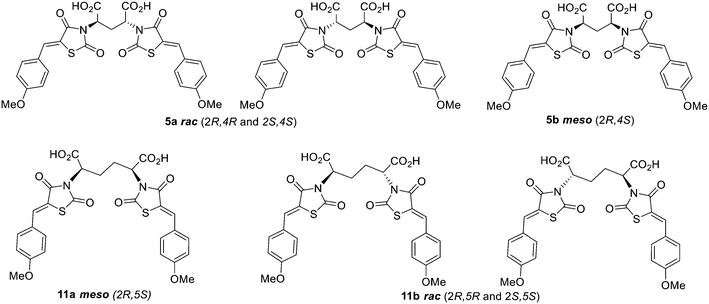 |
| Fig. 4 Dimeric 2,4-thiazolidinediones synthesised with methylene or ethylene bridges. | |
Lastly, a compound possessing a propylene bridge was synthesised via the N-alkylation of 2,4-thiazolidinedione with dimethyl 2,6-dibromoheptandioate 12 in the presence of potassium hydrogen carbonate in N,N-dimethylformamide to afford compound 13. Knoevenagel condensation resulted in diester 14 which was followed by acid hydrolysis to afford the desired diacid product 15 as a mixture of rac and meso diastereomers in an approximate 1
:
1 ratio according to proton NMR spectroscopy. Recrystallisation, column chromatography and semi-preparative RP-HPLC were attempted to separate the meso and rac compounds, initially as the desired acid products but also at the stage of the precursor esters. Unfortunately, these efforts went unrewarded. It was decided to test compound 15 as a mixture of diastereomers and then undertake alternative strategies for purification if it was warranted by the initial assay results.
Biological assay results
The synthesised MBDTA-2 analogues were subjected to biological testing against E. coli DHDPS in vitro via the DHDPS–DHDPR coupled enzyme assay (Table 1).33 All dimeric compounds saw improved inhibitory in vitro activity towards E. coli DHDPS, with the exception of the extended diacid analogue 8 which was not expected to have comparable inhibition given the absence of the second 2,4-thiazolidinedione moiety. The most potent compound was found to be meso compound 5b with the methylene bridge which had an improved IC50 value of 9.95 ± 0.6 μM against E. coli DHDPS followed by rac compound 11b with an ethylene bridge with an IC50 value of 19.7 ± 1.5 μM. The mixture of stereoisomers of compound 15 with a propylene bridge did not see a significant improvement in potency over the methylene or ethylene bridged analogues with an IC50 of 40.6 ± 3.0 μM, thus separation of the stereoisomers of this compound was not pursued any further.
Table 1 IC50 values of inhibitors against E. coli DHDPS
Compound |
Bridge length |
Stereochemistry |
E. coli IC50 (μM) |
MBDTA-2
|
— |
achiral
|
47.0 ± 2.3 |
5a
|
n = 1 |
rac (R,R and S,S) |
30.4 ± 1.8 |
5b
|
n = 1 |
meso
|
9.95 ± 0.6 |
8
|
— |
rac (R and S) |
77.4 ± 5.8 |
11a
|
n = 2 |
meso
|
42.0 ± 0.8 |
11b
|
n = 2 |
rac (R,R and S,S) |
19.7 ± 1.5 |
15
|
n = 3 |
meso, rac (R,R and S,S) |
40.6 ± 3.0 |
Compound 5b was then selected for testing against the two orthologues of Arabidopsis thaliana to evaluate potential herbicidal activity of this series. 5b was found to inhibit with an IC50 35.6 ± 7.4 μM and 33.9 ± 8.0 μM against DHDPS1 and DHDPS2 respectively. Inhibition efficacy was found to be slightly weaker than that observed against bacterial E. coli DHDPS, though such variation is to be expected as the allosteric site is only semi-conserved across species.
Conclusion
With DHDPS as a target of significant interest in the development of new antibiotics and herbicides, a series of dimeric chiral 2,4-thiazolidinedione compounds consisting of a methylene, ethylene and propylene bridge were designed and synthesised. Separation of the meso isomer for this series was achieved by trituration and/or recrystallisation while the remaining R,R and S,S enantiomers required separation by RP-HPLC in order to remove traces of the meso compound. By taking advantage of the symmetrical nature of the target, allosteric inhibition of DHDPS was improved by approximately five-fold over the single ligand. The most potent inhibitor was found to be compound 5b as a meso dimer containing a methylene bridge which was found to possess an IC50 value of 9.95 ± 0.6 μM against E. coli DHDPS. This work describes the first low micromolar dimeric inhibitor of E. coli DHDPS and further supports the continued exploration of this enzyme as biological target of significant interest.
Conflicts of interest
There is no conflict of interest to declare.
Acknowledgements
We are very grateful for the support and many helpful chemical discussions provided by Dr. Les Deady. R. M. C. would like to acknowledge the Australian Government as a recipient of an Australian Government Research Training Program Scholarship and the assistance provided by a LIMS Write-Up Award. T. P. S. C. acknowledges support from an Australian National Health and Medical Research Council Early Career Fellowship (APP1091976) and Australian Research Council Discovery Early Career Researcher Award (DE190100806).
References
- G. Mei, A. Di Venere, N. Rosato and A. Finazzi-Agro, The importance of being dimeric, FEBS J., 2005, 272(1), 16–27 CrossRef CAS PubMed.
- C. N. Chi, A. Bach, M. Gottschalk, A. S. Kristensen, K. Strømgaard and P. Jemth, Deciphering the Kinetic Binding Mechanism of Dimeric Ligands Using a Potent Plasma-stable Dimeric Inhibitor of Postsynaptic Density Protein-95 as an Example, J. Biol. Chem., 2010, 285(36), 28252–28260 CrossRef CAS PubMed.
- S. J. F. Macdonald, K. G. Watson, R. Cameron, D. K. Chalmers, D. A. Demaine, R. J. Fenton, D. Gower, J. N. Hamblin, S. Hamilton, G. J. Hart, G. G. A. Inglis, B. Jin, H. T. Jones, D. B. McConnell, A. M. Mason, V. Nguyen, I. J. Owens, N. Parry, P. A. Reece, S. E. Shanahan, D. Smith, W.-Y. Wu and S. P. Tucker, Potent and Long-Acting Dimeric Inhibitors of Influenza Virus Neuraminidase Are Effective at a Once-Weekly Dosing Regimen, Antimicrob. Agents Chemother., 2004, 48(12), 4542–4549 CrossRef CAS PubMed.
- M. K. Hadden and B. S. J. Blagg, Dimeric approaches to anti-cancer chemotherapeutics, Anti-Cancer Agents Med. Chem., 2008, 8(7), 807–816 CrossRef CAS PubMed.
- J. M. Ramanjulu, G. S. Pesiridis, J. Yang, N. Concha, R. Singhaus, S.-Y. Zhang, J.-L. Tran, P. Moore, S. Lehmann, H. C. Eberl, M. Muelbaier, J. L. Schneck, J. Clemens, M. Adam, J. Mehlmann, J. Romano, A. Morales, J. Kang, L. Leister, T. L. Graybill, A. K. Charnley, G. Ye, N. Nevins, K. Behnia, A. I. Wolf, V. Kasparcova, K. Nurse, L. Wang, Y. Li, M. Klein, C. B. Hopson, J. Guss, M. Bantscheff, G. Bergamini, M. A. Reilly, Y. Lian, K. J. Duffy, J. Adams, K. P. Foley, P. J. Gough, R. W. Marquis, J. Smothers, A. Hoos and J. Bertin, Design of amidobenzimidazole STING receptor agonists with systemic activity, Nature, 2018, 564, 439–443 CrossRef CAS PubMed.
- T. Drapier, P. Geubelle, C. Bouckaert, L. Nielsen, S. Laulumaa, E. Goffin, S. Dilly, P. Francotte, J. Hanson, L. Pochet, J. S. Kastrup and B. Pirotte, Enhancing Action of Positive Allosteric Modulators through the Design of Dimeric Compounds, J. Med. Chem., 2018, 61(12), 5279–5291 CrossRef CAS PubMed.
- B. R. Burgess, R. C. Dobson, M. F. Bailey, S. C. Atkinson, M. D. Griffin, G. B. Jameson, M. W. Parker, J. A. Gerrard and M. A. Perugini, Structure and evolution of a novel dimeric enzyme from a clinically important bacterial pathogen, J. Biol. Chem., 2008, 283(41), 27598–27603 CrossRef CAS PubMed.
- C. Mirwaldt, I. Korndörfer and R. Huber, The crystal structure of dihydrodipicolinate synthase from Escherichia coli at 2.5 A resolution, J. Mol. Biol., 1995, 227–239 CrossRef CAS PubMed.
- R. J. Cox, The DAP pathway to lysine as a target for antimicrobial agents, Nat. Prod. Rep., 1996, 13(1), 29–43 RSC.
- R. J. Cox, A. Sutherland and J. C. Vederas, Bacterial diaminopimelate metabolism as a target for antibiotic design, Bioorg. Med. Chem., 2000, 8(5), 843–871 CrossRef CAS PubMed.
- C. A. Hutton, T. J. Southwood and J. J. Turner, Inhibitors of lysine
biosynthesis as antibacterial agents, Mini-Rev. Med. Chem., 2003, 3(2), 115–127 CrossRef CAS PubMed.
- C. A. Hutton, M. A. Perugini and J. A. Gerrard, Inhibition of lysine biosynthesis: an evolving antibiotic strategy, Mol. BioSyst., 2007, 3(7), 458–465 RSC.
- C. V. Coulter, J. A. Gerrard, J. A. E. Kraunsoe and A. J. Pratt, Escherichia coli dihydrodipicolinate synthase and dihydrodipicolinate reductase: kinetic and inhibition studies of two putative herbicide targets, Pestic. Sci., 1999, 55(9), 887–895 CrossRef CAS.
- W. E. Karsten, Dihydrodipicolinate synthase from Escherichia coli: pH dependent changes in the kinetic mechanism and kinetic mechanism of allosteric inhibition by L-lysine, Biochemistry, 1997, 36(7), 1730–1739 CrossRef CAS PubMed.
- S. Blickling, C. Renner, B. Laber, H. D. Pohlenz, T. A. Holak and R. Huber, Reaction mechanism of Escherichia coli dihydrodipicolinate synthase investigated by X-ray crystallography and NMR spectroscopy, Biochemistry, 1997, 36(1), 24–33 CrossRef CAS PubMed.
- P. Shrivastava, V. Navratna, Y. Silla, R. P. Dewangan, A. Pramanik, S. Chaudhary, G. Rayasam, A. Kumar, B. Gopal and S. Ramachandran, Inhibition of Mycobacterium tuberculosis dihydrodipicolinate synthase by alpha-ketopimelic acid and its other structural analogues, Sci. Rep., 2016, 6, 30827 CrossRef CAS PubMed.
- B. Laber, F. X. Gomis-Rüth, M. J. Romão and R. Huber, Escherichia coli dihydrodipicolinate synthase. Identification of the active site and crystallization, Biochem. J., 1992, 288(2), 691–695 CrossRef CAS PubMed.
- F. I. Y. Yamakura, K. Kimura and T. Sasakawa, Partial purification and some properties of pyruvate-aspartic semialdehyde condensing enzyme from sporulating Bacillus subtilis, J. Biochem., 1974, 76(3), 611–621 CrossRef CAS PubMed.
- Y. V. Skovpen and D. R. J. Palmer, Dihydrodipicolinate synthase from Campylobacter jejuni: Kinetic mechanism of cooperative allosteric inhibition and inhibitor-induced substrate cooperativity, Biochemistry, 2013, 52(32), 5454–5462 CrossRef CAS PubMed.
- J. J. Turner, J. A. Gerrard and C. A. Hutton, Heterocyclic inhibitors of dihydrodipicolinate synthase are not competitive, Bioorg. Med. Chem., 2005, 13(6), 2133–2140 CrossRef CAS PubMed.
- L. Couper, J. E. McKendrick, D. J. Robins and E. J. T. Chrystal, Pyridine and piperidine derivatives as inhibitors of dihydrodipicolinic acid synthase, a key enzyme in the diaminopimelate pathway to L-lysine, Bioorg. Med. Chem. Lett., 1994, 4(19), 2267–2272 CrossRef.
- B. A. Boughton, L. Hor, J. A. Gerrard and C. A. Hutton, 1,3-Phenylene bis(ketoacid) derivatives as inhibitors of Escherichia coli dihydrodipicolinate synthase, Bioorg. Med. Chem., 2012, 20(7), 2419–2426 CrossRef CAS PubMed.
- B. A. Boughton, R. C. J. Dobson, J. A. Gerrard and C. A. Hutton, Conformationally constrained diketopimelic acid analogues as inhibitors of dihydrodipicolinate synthase, Bioorg. Med. Chem. Lett., 2008, 18(2), 460–463 CrossRef CAS PubMed.
- J. J. Turner, J. P. Healy, R. C. J. Dobson, J. A. Gerrard and C. A. Hutton, Two new irreversible inhibitors of dihydrodipicolinate synthase: diethyl (E,E)-4-oxo-2,5-heptadienedioate and diethyl (E)-4-oxo-2-heptenedioate, Bioorg. Med. Chem. Lett., 2005, 15(4), 995–998 CrossRef CAS PubMed.
- R. M. Christoff, C. K. Gardhi, T. P. Soares da Costa, M. A. Perugini and B. M. Abbott, Pursuing DHDPS: an enzyme of unrealised potential as a novel antibacterial target, MedChemComm, 2019, 10(9), 1581–1588 RSC.
- Y. V. Skovpen, C. J. T. Conly, D. A. R. Sanders and D. R. J. Palmer, Biomimetic design results in a potent allosteric inhibitor of dihydrodipicolinate synthase from Campylobacter jejuni, J. Am. Chem. Soc., 2016, 138(6), 2014–2020 CrossRef CAS PubMed.
- R. M. Christoff, T. P. Soares da Costa, S. Bayat, J. K. Holien, M. A. Perugini and B. M. Abbott, Synthesis and structure-activity relationship studies of 2,4-thiazolidinediones and analogous heterocycles as inhibitors of dihydrodipicolinate synthase, Bioorg. Med. Chem., 2021, 52, 1–9 CrossRef PubMed.
-
M. A. Perugini, B. M. Abbott and T. P. Soares da Costa, Heterocylic inhibitors of lysine biosynthesis via the diaminopimelate pathway, WO2018187845, 2018.
- T. P. Soares da Costa, C. J. Hall, S. Panjikar, J. A. Wylie, R. M. Christoff, S. Bayat, M. D. Hulett, B. M. Abbott, A. R. Gendall and M. A. Perugini, Towards novel herbicide modes of action by inhibiting lysine biosynthesis in plants, eLife, 2021, 10, e69444 CrossRef PubMed.
- E. R. R. Mackie, A. S. Barrow, R. M. Christoff, B. M. Abbott, A. R. Gendall and T. P. Soares da Costa, A dual-target herbicidal inhibitor of lysine biosynthesis, eLife, 2022, 11, e78235 CrossRef CAS PubMed.
- G. Guanti and R. Riva, Synthesis of chiral non-racemic azetidines by lipase-catalysed acetylations and their transformation into amino alcohols: precursors of chiral catalysts, Tetrahedron: Asymmetry, 2001, 12(4), 605–618 CrossRef CAS.
- V. P. Krasnov, E. A. Zhdanova, M. A. Korolyova, I. M. Bukrina, M. I. Kodess, V. K. Kravtsov and V. N. Biyushkin, Synthesis of stereoisomers of 2,4-diaminoglutaric and 2,5-diaminoadipic acids, Russ. Chem. Bull., 1997, 46(2), 319–323 CrossRef CAS.
- R. C. J. Dobson, J. A. Gerrard and F. G. Pearce, Dihydrodipicolinate synthase is not inhibited by its substrate, (S)-aspartate beta-semialdehyde, Biochem. J., 2004, 377(Pt 3), 757–762 CrossRef CAS PubMed.
Footnotes |
† Electronic supplementary information (ESI) available. CCDC 2235014–2235016. For ESI and crystallographic data in CIF or other electronic format see DOI: https://doi.org/10.1039/d3md00044c |
‡ Current address: School of Agriculture, Food and Wine, Waite Research Institute, The University of Adelaide, Waite Campus, Australia. |
|
This journal is © The Royal Society of Chemistry 2023 |
Click here to see how this site uses Cookies. View our privacy policy here.