DOI:
10.1039/D3MA00668A
(Paper)
Mater. Adv., 2023,
4, 5252-5262
A novel magnetic HS−-adsorptive nanocomposite photocatalyst (rGO/CoMn2O4–MgFe2O4) for hydrogen fuel production using H2S feed†
Received
5th September 2023
, Accepted 18th September 2023
First published on 19th September 2023
Abstract
Synthesis of low-cost, eco-friendly, semiconducting solar-energy materials with excellent photocatalytic activity [high surface area, good reactant adsorption, photon harnessing in the visible region, and low charge recombination] for application in pollutant conversion to hydrogen is of great importance from environmental remediation as well as green energy and fuel production perspectives. In the present work, a magnetic heterojunction of CoMn2O4/MgFe2O4 and reduced graphene oxide (rGO) was synthesized through a combined Hummers’/hydrothermal method. The obtained nanocomposite (rGO/CoMn2O4–MgFe2O4) was employed for photocatalytic conversion of H2S feed into hydrogen fuel. Adsorption studies in the feed solution proved a good capability for the photocatalyst to adsorb HS− reactant from the reaction medium. This effect was ascribed to the presence of the CoMn2O4 component, serving as a strong bisulfide adsorbent. VSM (vibrating sample magnetometry) analysis revealed that the magnetic property of the photocatalyst was due to the MgFe2O4 component. Photocatalytic investigations showed that the addition of rGO to the CoMn2O4/MgFe2O4 nanocomposite not only improves its reactant adsorption capacity, but also increases the photocatalyst surface area, enhances photon absorption, and suppresses the charge (e/h) recombination, which eventually boosts the photocatalyst activity to produce more hydrogen fuel (∼1.5 times).
1. Introduction
Pollutant elimination and conversion into hydrogen fuel using effective, affordable semiconducting energy materials is a green and economic strategy towards environmental remediation and sustainable supplying of the fuel/energy demand.1–4 One of the most dangerous and toxic pollutants is H2S gas, generated on a large scale during various natural and industrial processes.5–7 Facing the H2S issue, the existing routes to solve the problem are the use of adsorbents, thermal decomposition, electrochemical and photo-splitting, and burning in an oxygen atmosphere (Claus process) with the aim of H2S elimination and production of hydrogen, sulfur, or other value-added materials.8–11 Among these methods, the utilization of photons (sunlight) and semiconducting photocatalyst materials is an easy and economical way to convert this harmful pollutant into hydrogen fuel, and has attracted the attention of many researchers in recent years.12–14 In this regard, magnetic adsorbing nanocomposite materials look ideal because, at the end of the photoconversion process, the photocatalyst particles can be easily collected and separated from the reaction medium by using an appropriate magnet.15–18 Furthermore, considering the factors such as: (1) the use of earth-abundant elements and affordable eco-friendly chemicals, (2) the attempt to reach a large surface area, strong absorption of photons, low charge recombination, and appropriate energy levels, and (3) the ability of the photocatalyst to adsorb reactant species, are crucial in the design and synthesis of effective photocatalyst materials for large-scale applications.19,20 To achieve these goals, the rational synthesis of composite photocatalysts with tailored physicochemical properties is an effective strategy.13,21,22
Regarding the semiconducting components of the nanocomposite photocatalyst synthesized in this work, it is worth mentioning that CoMn2O4 is a narrow-bandgap p-type semiconductor, which has been widely used for the photocatalytic degradation of organic pollutants.23,24 Moreover, due to the presence of Co and Mn, strong hydrogen sulfide adsorption is anticipated for this material.13,25–27 The disadvantage of this semiconductor is its high charge recombination, which can be solved by making a composite with an n-type semiconductor.28,29 In the present study, MgFe2O4 was used as a narrow-bandgap n-type magnetic semiconductor. The photocatalytic application of this material has also been reported in the literature for degradation of organic pollutants and water splitting as well.30,31
Since H2S photoconversion is a heterogeneous process and the conversion does not occur until the reactant species adsorb on the photocatalyst surface, the surface area plays a crucial role in the photocatalytic activity.21,32–34 To increase the photocatalyst surface area and improve its sorption capability [photon absorption/harnessing and reactant adsorption], reduced graphene oxide (rGO) was employed and the ternary magnetic rGO/CoMn2O4–MgFe2O4 nanocomposite was synthesized for the first time and applied as an efficient photocatalyst for the production of hydrogen fuel using H2S feed.
2. Experimental section
2.1 Synthesis of GO, rGO, MgFe2O4, CoMn2O4 and their nanocomposites
Graphene oxide (GO) was synthesized through a Hummers’ method with some modifications.35–37 For this purpose, 2 g graphite powder (particle size: 0.4–1.2 μm, purity: 99.9%; US Research Nanomaterials, Inc.) and 4 g NaNO3 (99%; Fluka) were added to 100 mL of concentrated H2SO4 (98%; Merck). The obtained mixture was placed in an ice water bath inside a laboratory hood and stirred for 30 min. Without removing the reaction vessel from the bath, 4 g KMnO4 (99%; Fluka) was gradually (bit by bit) added to the reaction solution and stirred for 4 h. The acidic viscous mixture obtained from the previous step was slowly added to a solution containing 100 mL of deionized water (DW) and 10 mL of H2O2 (30%; Merck) and stirred for 15 min. Then the precipitate obtained from this step was filtered and washed with dilute HCl (0.1 M) and thereafter rewashed several times with DW. At the end, the precipitate was dried at 70 °C for 12 h.
To prepare reduced graphene oxide (rGO), a chemical reduction method was applied.38–41 Accordingly, 100 μL of hydrazine (a reducing agent; H6N2O, 80%, Merck) was added to 100 mL DW containing 0.3 g GO (synthesized in the previous stage). The mixture was then refluxed with stirring for 8 h at 80 °C. Finally, the resulting precipitate was filtered and dried after washing several times with DW.
For the synthesis of CoMn2O4 and MgFe2O4 semiconductors, a hydrothermal method was used.42–46 For CoMn2O4, 50 mL of an aqueous solution containing 5 mmol of Co2+ (Co(NO3)2·6H2O; 98%; Fluka) and 10 mmol of Mn2+ (Mn(NO3)2·4H2O; 98%; Fluka) was first prepared. Then, 1 M NaOH was added dropwise with stirring of the solution until pH 11 was reached. The obtained mixture was subsequently transferred into a Teflon-lined stainless steel autoclave and heated at 180 °C for 8 h. At the end, after washing several times with DW, the precipitate obtained was dried at 80 °C for 8 h. For the synthesis of MgFe2O4, using a 50 mL solution containing 5 mmol of Mg2+ (Mn(NO3)2·6H2O; 98%; Fluka) and 6.6 mmol of Fe3+ (Fe(NO3)3·9H2O; 98%; Fluka), the same procedure was repeated.
The above hydrothermal route was also employed in the synthesis of xCoMn2O4–yMgFe2O4 nanocomposites with different molar (x/y) ratios. For example, to synthesize the nanocomposite material with a 1
:
1 molar ratio (x/y = 1), 1.2 g CoMn2O4 (synthesized in the previous section) was ultrasonically dispersed in 50 mL DW for 15 min. Next, 5 mmol Mg2+ and 10 mmol Fe3+ were added to the mixture, and the pH of the medium was adjusted to 11 by adding NaOH solution (1 M) drop by drop while stirring the mixture. The mixture was then transferred into the autoclave and heated at 180 °C for 8 h. At the end, the obtained composite precipitate was washed several times with DW and dried at 80 °C for 8 h. To synthesize the other composites with the molar ratios of x/y = 2/1, 1/2 and 1/3, the same procedure was employed and the initial amounts of CoMn2O4 were 2.4, 0.6, and 0.4 g, respectively.
For the preparation of the ternary rGO/CoMn2O4–MgFe2O4 nanocomposite (termed as rGO/Compos), the synthesis process was conducted in the presence of 2 wt% rGO47 for the heterojunction composite with the molar ratio of 0.5, which exhibited the maximum performance (Fig. S1, ESI†). Therefore, for the synthesis of the rGO/Compos, a 50 mL aqueous solution containing rGO was first prepared and ultrasonically dispersed for half an hour. Then, by adding 0.6 g CoMn2O4, 5 mmol Mg2+ and 10 mmol Fe3+ to the mixture, the composite photocatalyst was synthesized through the same hydrothermal method described above. The photocatalyst preparation steps are depicted in Fig. 1.
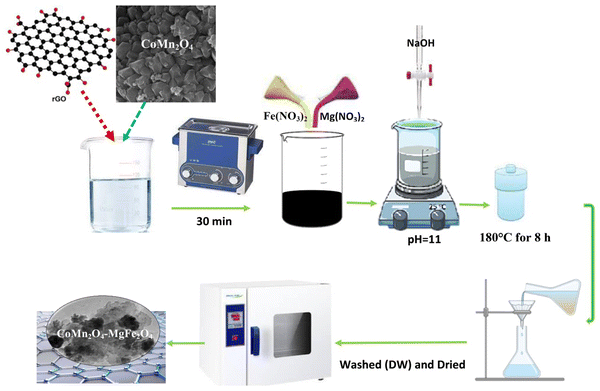 |
| Fig. 1 Schematic representation of the photocatalyst preparation route. | |
2.2 Material characterization
For characterization of the synthesized materials, we employed various techniques including X-ray diffraction (XRD), X-ray photoelectron spectroscopy (XPS), Fourier transform infrared (FTIR), photoluminescence (PL) and diffuse reflectance (DR) UV-Visible spectroscopies, vibrating sample magnetometry (VSM), field emission scanning electron and high-resolution transmission electron microscopies (FESEM and HRTEM), and the Mott–Schottky method. XRD patterns, XPS, and VSM data were obtained using a Philips X’Pert Pro X-ray diffractometer (λ = 1.54 D; Cu Kα beam), a SPECS Phoibos 100 (1D-DLD) analyzer (excitation: 1486.61 eV) and a vibrating sample magnetometer (Magnetic Daghigh Daneshpajouh Co.), respectively. To record FTIR, PL, and DR UV-Vis spectra of the photocatalyst materials, BRUKER Vector 22, Varian Cary Eclipse Fluorescence (λex = 350 nm) and 5E UV-Vis-NIR spectrophotometers were utilized, respectively. The FESEM and HRTEM images of the synthesized materials were taken using Tescan Mira3 and JEM-2100 microscopes, respectively. Mott–Schottky diagrams were recorded at 1 kHz using an Ivium-Vertex Potentio-Galvanostat in a three-electrode setup [electrolyte: 0.5 M Na2S (pH = 11); the working, counter, and reference electrodes were photocatalyst film, Pt-foil (2.5 cm2), and Ag/AgCl (3 M), respectively; for details of the approach, see ref. 6.
2.3 N2 and bisulfide adsorption tests
Nitrogen adsorption–desorption isotherm and porosimetry data were obtained at 77 K using a BELSORB-max (BEL, Japan) instrument. In order to determine the capacity of the photocatalyst to sorb the reactant species (HS−; see Section 2.4), a 0.5 M Na2S solution was prepared, and its pH decreased to 11 by gradually adding a concentrated HCl to the medium [this solution is equivalent to the H2S feed used for the conversion process, ref. 19. The bisulfide adsorption tests were conducted for the photocatalyst materials in the mentioned medium (solid to liquid ratio: 4 g L−1) at 25 °C in a sealed beaker under magnetically stirring conditions. After a fixed solid–liquid contact time (3 h), the photocatalyst powder was separated through a vacuum filter using a Büchner funnel, followed by drying at 70 °C overnight. The amount of S adsorbed by the photocatalyst (mass percent) was measured using a SC-144DR sulfur and carbon analyzer. Each test was repeated at least twice and the mean value was reported as final data.
2.4 Photoreactor setup and H2S conversion to hydrogen fuel
The photoreactor employed in this work was a handmade vertical double-walled T-controlling cylindrical glass vessel illuminated by xenon light with the intensity adjusted to 1 sun (100 mW cm−2).48,49 The capacity of the reactor was 50 mL and the reaction medium was an alkaline H2S-saturated feed at pH = 11 (H2S + OH− ⇌ HS− + H2O8,19), containing 0.2 g photocatalyst powder–suspended magnetically during the process.19,50 The pH of the medium was set to 11, because at this pH, bisulfide (HS−) is the dominant species and the maximum performance (H2 production) is achieved.8,19 The amount of hydrogen gas released during the photoconversion process was measured every 10 min through a volumetric method described in detail elsewhere.51
3. Results and Discussion
3.1 Photocatalyst synthesis and characterization
The X-ray diffraction (XRD) patterns of graphite (G), graphene oxide (GO), and reduced graphene oxide (rGO) are shown in Fig. 2(a). The examination of this figure clearly indicates that the peak around 27° is the characteristic (002) peak of graphite,52,53 whose intensity significantly decreases by exfoliation/oxidation of graphene layers and production of GO. Also, the appearance of a peak at ca. 13° is known as the characteristic XRD peak of GO, which is not observed in the case of G.54,55 With the reduction of GO and synthesis of rGO, the intensity of this peak decreases, and by contrast, the intensity of the graphitic ones (peaks at 27 and 42°) is augmented. These facts and the appearance of a new peak at ∼77°, all indicate the reduction of GO and successful synthesis of rGO.54,56
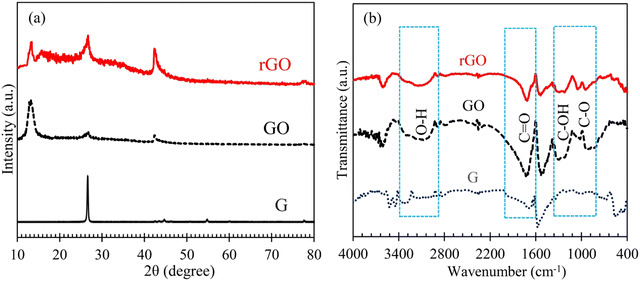 |
| Fig. 2 XRD patterns (a) and FTIR spectra (b) of the graphite (G), graphene oxide (GO; exfoliated graphite via Hummers’ method) and reduced graphene oxide (rGO) synthesized/employed in this work. | |
In addition to XRD, the FTIR technique was used to further corroborate the synthesis of GO and rGO; see Fig. 2(b). This figure clearly shows that during the Hummers’ process and G conversion (exfoliation/oxidation) to GO, the IR bands related to O–H, C
O, C–OH and C–O functional groups emerged/are strengthened.57,58 With the reduction of GO, the intensity of the mentioned peaks is reduced and the conversion of GO to rGO is confirmed.57,58 N2 adsorption–desorption studies of GO and rGO (Fig. S2, ESI†) showed a mesoporous structure (pore diameter between 2 and 50 nm) for these materials. Furthermore, by the reduction of GO, its surface area of 8.98 m2 g−1 increased by about 14%.59,60
The XRD diagram of the composite photocatalysts is presented in Fig. 3. Examining this diagram shows that the XRD of MgFe2O4 and CoMn2O4 are consistent with JCPDS 01-073-1960 and JCPDS 00-001-1126 respectively, indicating a spinel structure for the mentioned compounds.28,61,62 The presence of these peaks in the composite photocatalyst proved their synthesis.
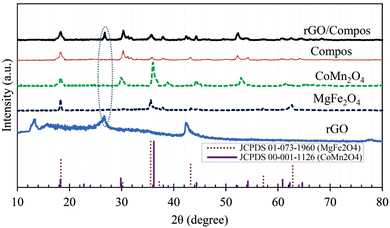 |
| Fig. 3 X-ray diffraction (XRD) patterns of the magnetic nanocomposite solar-energy materials (Compos: CoMn2O4–MgFe2O4, rGO/Compos: rGO/CoMn2O4–MgFe2O4) along with their constituents (i.e. CoMn2O4, MgFe2O4, and rGO: reduced graphene oxide). | |
In the XRD pattern of the rGO/Compos, in addition to the peaks of the Compos, the presence of the characteristic peak of rGO confirmed the successful synthesis of this composite photocatalyst/solar-energy material. Furthermore, the careful examination of the XRD diagram of rGO/Compos indicates that during the hydrothermal synthesis, the remaining GO characteristic peak (∼13°; see Fig. 1(a) and 2) completely disappears. Such a phenomenon has also been seen in other works, which indicates the completion of the GO reduction–occurring during the hydrothermal process.35,63 Regarding this fact of why the reduction of GO becomes completed during the synthesis of rGO/Compos, it can be briefly noted that under hydrothermal conditions, the superheated water (SW) in the autoclave reactor (T = 180 °C) serves as a supercritical reducing medium. Hence, further de-oxygenation of GO occurs via the H+/OH− catalyzed dehydration process,64–67 in which SW acts as an improved electrolyte (H+/OH−) [notice: since water autoionization, i.e. H2O ⇌ H+ + OH− is an endothermic process, KW increases with temperature and more protons/hydroxides are generated under supercritical/hydrothermal conditions]. In addition to XRD, the presence of components and their constituent elements in the photocatalyst materials was confirmed by EDX data (Table 1 and Fig. S3, ESI†). XPS investigations of the composite photocatalyst verified the oxidation states of +2 for Co and Mg, and +3 for Fe and Mn elements [Table 2 and Fig. S4, ESI†].
Table 1 Energy dispersive X-ray spectroscopic (EDX/EDS) data (wt%) of the photocatalyst materials synthesized in this study
Photocatalyst |
Co |
Mn |
Mg |
Fe |
O |
C |
CoMn2O4 |
20.57 |
41.37 |
— |
— |
38.06 |
— |
MgFe2O4 |
— |
— |
12.02 |
50.16 |
37.82 |
— |
Compos |
8.70 |
17.03 |
7.18 |
29.86 |
37.23 |
— |
rGO/Compos |
8.14 |
15.62 |
6.50 |
29.09 |
38.83 |
1.82 |
Table 2 XPS results for the composite photocatalyst in the presence of rGO
Atom |
Spectral line |
Binding energya (eV) |
Oxidation state |
Ref. |
Binding energies were corrected with respect to 284.8 eV (the adventitious C 1S peak).6
|
Co |
2p3/2 |
780.6 |
+2 |
68,69
|
2p1/2 |
796.4 |
Mn |
2p3/2 |
641.6 |
+3 |
28
|
2p1/2 |
653.3 |
Mg |
2p3/2 |
49.5 |
+2 |
31
|
2p1/2 |
61.3 |
Fe |
2p3/2 |
712.5 |
+3 |
70,71
|
2p1/2 |
725.2 |
C |
1s |
284.5, 286.4, 287.8 |
+2, +1, 0 |
72,73
|
O |
1s |
529.4, 530.8, 531.6 |
−2 |
42,74–76
|
In the XPS spectra of carbon (Fig. 4), the deconvoluted peaks around 284.5, 286.4, and 287.8 eV are linked to C
C (dotted line refers to the resonance between single and double bonds), C–O, and C
O bonds of rGO, respectively.72 With the synthesis of rGO/Compos, the intensity of the C
O peak (∼287.8 eV) decreases significantly and gets almost halved [see Fig. 4(a) and (b) and compare the intensities]. This observation can be attributed to the completion of rGO reduction occurring during the hydrothermal synthesis of the rGO/Compos, which has already been recognized by the disappearance of the XRD peak at 2θ = 13° (Fig. 3).
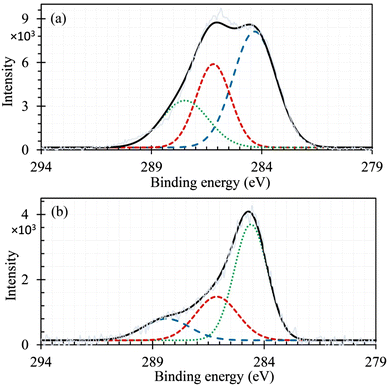 |
| Fig. 4 XPS spectra of C (1s) recorded for (a) pristine rGO and (b) rGO/Compos [the noisy and corresponding smooth curves represent raw and enveloped data; for the survey spectra, see Fig. S4a and S5, ESI†]. | |
The magnetic behavior of the heterojunction nanocomposite photocatalyst synthesized in the absence and presence of rGO is depicted in Fig. 5. Examining this figure indicates that both photocatalysts are soft ferromagnets with a saturation/spontaneous magnetization of 3.2 and 10 emu g−1 for Compos and rGO/Compos materials, respectively.77,78 It should be noted that the magnetic property of the Compos material is due to the MgFe2O4 component [magnetization: ∼22 emu g−1; see Fig. S6, ESI†]. Furthermore, the incorporation of rGO into the composite photocatalyst significantly enhances its magnetic properties. To the best of our knowledge, although no specific reason was reported for the mentioned effect, it is generally believed that rGO could facilitate electron hopping/transfer79,80 in the composite network, improving the magnetic property of the rGO/Compos in comparison to that of Compos alone.
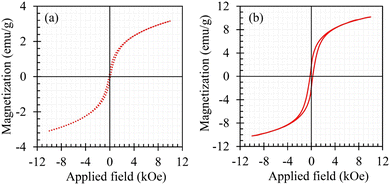 |
| Fig. 5 Magnetic hysteresis loop of the heterojunction composite photocatalyst (Compos) in the absence (a) and presence of rGO (b). | |
Scanning electron micrographs of the composite photocatalyst in the absence and presence of rGO are shown in Fig. 6. It is evident that in the presence of rGO (Fig. 6(c)), the morphology of the photocatalyst (Fig. 6(a)) is changed and a hybrid/combined structure (Fig. 6(b)) appears for the resulting rGO/photocatalyst composite [for additional images and BET data of the components, refer to the ESI,† Fig. S7, S8, and S2].
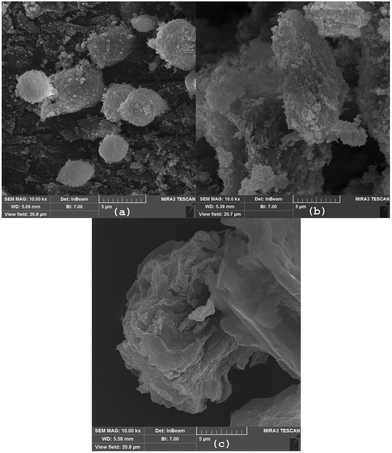 |
| Fig. 6 FESEM images of the magnetic nanocomposite photocatalyst taken in the absence (a) and presence (b) of rGO (c). | |
The layered morphology of rGO and the placement/deposition of the composite (Compos) nanoparticles on it were further confirmed through HRTEM observations; see Fig. 7 [for the images of CoMn2O4 and MgFe2O4, refer to Fig. S9, ESI†].
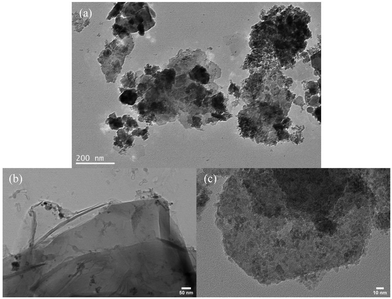 |
| Fig. 7 HRTEM images of the nanocomposite photocatalyst (Compos; a), rGO (b), and rGO/Compos (c). | |
The porous morphology recognized through microscopic images for the composite photocatalyst was also verified via N2 adsorption/desorption analyses conducted in the absence and presence of rGO (Fig. 8). A pore diameter of 39.8 nm was obtained for Compos, which was reduced to 10.1 nm by compositing the Compos photocatalyst with rGO. Furthermore, the presence of rGO caused a three-fold increase in the surface area of the resulting composite photocatalyst (Fig. 8, compare the s values).
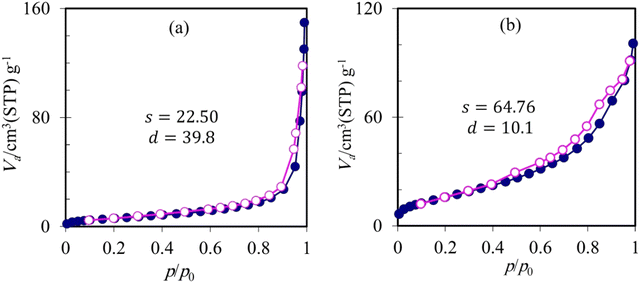 |
| Fig. 8 Nitrogen adsorption–desorption (BET) isotherms of the composite photocatalyst (Compos) in the absence (a) and presence (b) of rGO (in these diagrams, s and d stand for surface area (m2 g−1) and mean pore diameter (nm), respectively). | |
3.2 Determining factors on photocatalyst activity for effective hydrogen fuel production using H2S feed
In addition to having a high surface area, an efficient photocatalyst material should be able to harness photons in the visible region and exhibit low recombination between photogenerated e/h pairs.2,6Fig. 9 shows that the composite photocatalyst synthesized in this work can absorb photons in a wide UV-Vis region and by compositing with rGO, not only the ability of the photocatalyst to absorb incident light increases (Fig. 9(a)) but the extent of charge recombination (PL emission) is also diminished (Fig. 9(b)). The good ability of the Compos to harness photons can be attributed to the presence of MgFe2O4 and CoMn2O4 semiconducting components, which serve as a superior light absorber (
) and preventer of charge recombination (
),6 respectively. Concerning rGO/Compos, it should also be noted that since rGO is a good electronic (e/h) conductor,81 the charge transfer can be facilitated within the composite material, thereby improving the charge separation and hence boosting the photocatalyst activity.47 By utilizing the absorption data and Kubelka–Munk approach,48 the band gap of the Compos photocatalyst was determined to be around 1.5 eV (refer to Fig. S12, ESI†). Moreover, the incorporation of rGO and the synthesis of rGO/Compos resulted in a reduced bandgap of ∼1.2 eV, indicating the enhanced ability of the photocatalyst/solar-energy material to harness more photons.
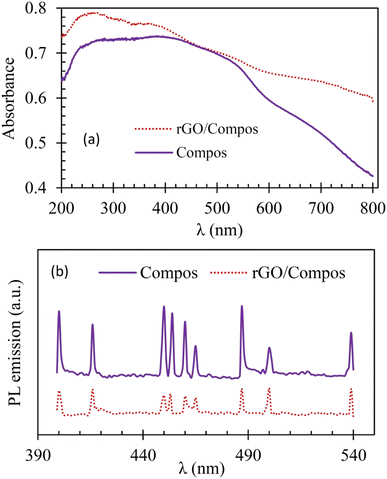 |
| Fig. 9 Diffuse reflectance (DR) UV-visible (a) and photoluminescence (PL; b) spectra of the Compos and rGO/Compos photocatalyst/solar-energy materials under consideration [for spectra of CoMn2O4 and MgFe2O4, refer to the ESI† (Fig. S10)]. | |
The photocatalytic production of hydrogen gas using H2S feed can be well rationalized taking into account that bisulfide–the dominant species in the reaction medium–plays a crucial role during the photo-transformation process. The reactant species is initially adsorbed on the photocatalyst surface and subsequently converted to hydrogen fuel through the following reduction reaction:8,13
| 2HS− + 2eCB− → H2↑ + 2S2− | (1) |
Therefore, it is expected that a photocatalyst with higher bisulfide adsorption capacity (BAC) and superior light absorption ability should produce more hydrogen gas. The mentioned adsorption capacity was measured for the composite photocatalysts under consideration and the results are presented in Table 3.
Table 3 Ability of photocatalysts to adsorb bisulfide anions from the reaction medium [initial pH: 11; data reported as mass percentage of sulfur sorbed by the photocatalyst material]
Photocatalyst |
S (wt%) |
pHa |
Measured at the end of the adsorption process.
|
Compos |
4.02 |
11.57 |
rGO/Compos |
4.57 |
11.59 |
The data listed in Table 3 indicate that both composite photocatalysts have a good ability to adsorb bisulfide anions. By adding rGO and making rGO/Compos, the adsorption capacity is improved and the production of more hydrogen gas is anticipated. Table 3 also reveals that by adsorption of bisulfide on the photocatalyst surface, the pH of the medium increases. This fact can be rationalized in terms of the equilibrium existing between sulfide and bisulfide species in the alkaline reaction medium:19
| 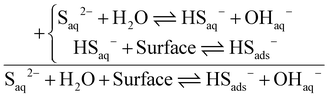 | (2) |
Eqn (2) shows that by the adsorption of bisulfide (= its removal from the aqueous solution), the equilibrium is shifted to the right, i.e. the production of more hydroxide anions [Le Châtelier's principle]. Therefore, the pH increase is justified. Regarding bisulfide adsorption by the composite photocatalysts, it should also be noted that between MgFe2O4 and CoMn2O4 components, the latter is mainly responsible for the adsorption of bisulfide anions on the photocatalyst material (Table S1, ESI†). To rationalize the good capacity of CoMn2O4 in bisulfide adsorption, it should be noted that both metallic cations, particularly Co2+ can strongly interact with sulfide species (KSP(CoS) ≤ 4.0 × 10−21, KSP(MnS) ≤ 2.5 × 10−10;82 HS− ⇌ H+ + S2−), providing a reason why Co/Mn-containing materials could exhibit good adsorption.83–85
Among the photocatalyst materials synthesized in this work, owing to the high surface area, strong absorption of photons across a broad range of the UV-Vis. spectrum, low charge recombination, and good potency to adsorb the reactant species, the composite rGO/Compos photocatalyst should exhibit the highest H2 release. Fig. 10 confirms this prediction, showing that the maximum performance (hydrogen evolution) is attained upon the mentioned photocatalyst material. Specifically, the rGO/Compos yields approximately 120 mL of H2 gas after 3 hours of illumination, surpassing the Compos photocatalyst by around 50%.
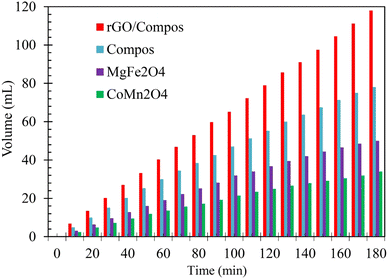 |
| Fig. 10 Volume of hydrogen gas evolved photocatalytically as a function of irradiation time (data recorded every 10 min under atmospheric pressure at 298 K; the reaction chamber contained 0.2 g of photocatalyst powder dispersed in a 50 mL H2S alkaline solution). | |
Fig. 10 also demonstrates that the lowest photocatalytic activity is due to the CoMn2O4 component. Concerning this observation, we should clarify that despite its highest BAC, CoMn2O4 cannot serve as a good photocatalyst, because of its poor photon absorption (harnessing incident light) and lowest surface area (Fig. S10 and S8, ESI†). Regarding rGO/Compos, which showed the highest gas release, it should finally be noted that this recyclable composite photocatalyst/solar-energy material has adequate photostability and the decline in its activity is insignificant in long-term use (Fig. 11).
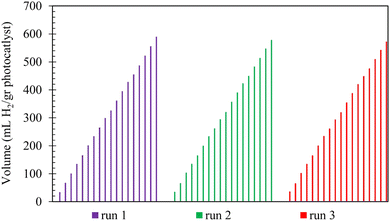 |
| Fig. 11 Volume of hydrogen gas evolved over the rGO/Compos photocatalyst for 3 successive runs/cycles. After each run, the photocatalyst was collected and used in the subsequent test. Each run/cycle lasted 3 h and the volume of gas was recorded every 10 minutes. | |
Concerning the mechanism of photocatalytic conversion of bisulfide to hydrogen fuel and the role of semiconducting components and rGO, we can briefly state that with the formation of the Compos photocatalyst (CoMn2O4–MgFe2O4), photoexcited electrons in the conduction band (CB) of the p-type component (CoMn2O4) are transferred to the CB of the n-type semiconductor (MgFe2O4), and consumed in the hydrogen production process (eqn (1)); see Fig. 12.
| 2HS− + 2hVB+ → S22− + 2H+ | (3) |
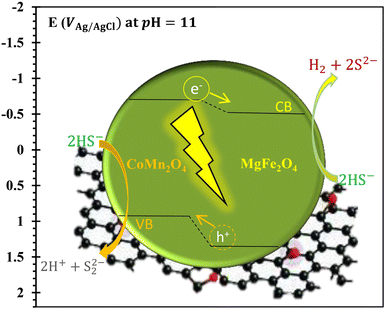 |
| Fig. 12 Conversion mechanism and production of hydrogen gas using a bisulfide anion upon the rGO-based nanocomposite photocatalyst in the H2S saturated alkaline medium (pH = 11) [see ref. 2, 13, 69 and 87, and Fig. S11 and S12, ESI†]. | |
Therefore, with the formation of the pn composite (Compos), the charge (e/h) separation is improved and the photogenerated charges can hence be effectively consumed in the bisulfide redox processes, resulting in a higher performance if compared to that of components alone. The presence of rGO also significantly enhanced the activity of the rGO/Compos photocatalyst (Fig. 10), in terms of improving the absorption of incident light and boosting charge transfer/separation86 (Fig. 9), increasing the photocatalyst surface area (Fig. 8), and enhancing its adsorption capacity for the reactant species (Table 3).
4. Conclusion
In the present work, a magnetic bisulfide adsorbing nanocomposite of p-CoMn2O4/n-MgFe2O4 (Compos) and rGO (reduced graphene oxide) was synthesized using a combined Hummers’/hydrothermal method and eco-friendly affordable materials. The synthesized nanocomposite was then applied for the photocatalytic conversion of H2S hazmat to hydrogen fuel. Based on this study, the following conclusions can be drawn:
• The photocatalytic activity of Compos is significantly boosted by adding rGO, in terms of increasing the photocatalyst surface area, enhancing its ability to absorb more photons, reducing charge recombination, and improving the adsorption of the reactant (HS−) species.
• Bisulfide adsorption capacity investigations showed that the Compos photocatalyst has a good potency to adsorb the reactant species. Moreover, the presence of rGO further enhances the adsorption ability of the photocatalyst. The good adsorption capacity of the Compos photocatalyst is linked to the presence of the CoMn2O4 component.
• Both nanocomposite photocatalysts synthesized in this work were magnetic; by adding rGO to the Compos and synthesis of the rGO/Compos, the magnetic property of the resulting nanocomposite material was improved.
• Among different xCoMn2O4–yMgFe2O4 [r (= x/y): 0, 0.33, 0.5, 1, 2 and ∞] composites, the maximum photocatalytic activity (hydrogen production) was obtained for the composite material with r = 0.5. In the presence of rGO, the activity of Compos was promoted about 1.5 times.
Conflicts of interest
There are no conflicts of interest to declare.
Acknowledgements
The authors would like to acknowledge the anonymous Referees as well as the Editor of this journal for their useful comments to enhance the quality of the work.
References
- A. Kumar, P. Choudhary, A. Kumar, P. H. C. Camargo and V. Krishnan, Recent advances in plasmonic photocatalysis based on TiO2 and noble metal nanoparticles for energy conversion, environmental remediation, and organic synthesis, Small, 2022, 18, 2101638 CrossRef CAS PubMed.
- M. Lashgari and M. Ghanimati, Pollutant photo-conversion strategy to produce hydrogen green fuel and valuable sulfur element using H2S feed and nanostructured alloy photocatalysts: Ni-dopant effect, energy diagram and photo-electrochemical characterization, Chem. Eng. Res. Des., 2020, 162, 85–93 CrossRef CAS.
- A. G. De Crisci, A. Moniri and Y. Xu, Hydrogen from hydrogen sulfide: towards a more sustainable hydrogen economy, Int. J. Hydrogen Energy, 2019, 44, 1299–1327 CrossRef CAS.
- M. A. Raja and V. Preethi, Performance of square and trapezoidal photoreactors for solar hydrogen recovery from various industrial sulphide wastewater using CNT/Ce+3 doped TiO2, Int. J. Hydrogen Energy, 2019, 45, 7616–7626 CrossRef.
- Y. Uesugi, H. Nagakawa and M. Nagata, Highly efficient photocatalytic degradation of hydrogen sulfide in the gas phase using anatase/TiO2(B) nanotube, ACS Omega, 2022, 7, 11946–11955 CrossRef CAS PubMed.
- M. Lashgari and M. Ghanimati, A new efficient eco-friendly quaternary solid-solution nanoenergy material for photocatalytic hydrogen fuel production from H2S aqueous feed, Chem. Eng. J., 2019, 358, 153–159 CrossRef CAS.
- M. Ferree, J. Kosco, F. Laquai, A. C. Sepulveda and D. P. S. Roman, Alerigi, Solar fuel production from hydrogen sulfide: An upstream energy perspective, Adv. Energy Sustainability Res., 2023, 2200201 CrossRef CAS.
- M. Lashgari, M. Sabeti-Khabbazmoayed and M. Konsolakis, A cost-effective H2S pollutant electro-transformation to hydrogen clean fuel and value-added semiconducting materials: A green alternative to Claus process, J. Ind. Eng. Chem., 2023, 122, 326–333 CrossRef CAS.
- H. Oladipo, A. Yusuf, S. Al Jitan and G. Palmisano, Overview and challenges of the photolytic and photocatalytic splitting of H2S, Catal. Today, 2021, 380, 125–137 CrossRef CAS.
- K. Vikrant, K. H. Kim and A. Deep, Photocatalytic mineralization of hydrogen sulfide as a dual-phase technique for hydrogen production and environmental remediation, Appl. Catal., B, 2019, 259, 118025 CrossRef CAS.
- M. Balsamo, S. Cimino, G. de Falco, A. Erto and L. Lisi, ZnO–CuO supported on activated carbon for H2S removal at room temperature, Chem. Eng. J., 2016, 304, 399–407 CrossRef CAS.
- S. B. Khan, M. S. J. Khan, K. Akhtar, E. M. Bakhsh, T. Kamal, A. M. Asiri and Y. Shen, Design of efficient solar photocatalytic system for hydrogen production and degradation of environmental pollutant, J. Mater. Res. Technol., 2021, 14, 2497–2512 CrossRef CAS.
- M. Lashgari and M. Ghanimati, An excellent heterojunction nanocomposite solar-energy material for photocatalytic transformation of hydrogen sulfide pollutant to hydrogen fuel and elemental sulfur: a mechanistic insight, J. Colloid Interface Sci., 2019, 555, 187–194 CrossRef CAS PubMed.
- H. Yi, D. Huang, L. Qin, G. Zeng, C. Lai, M. Cheng, S. Ye, B. Song, X. Ren and X. Guo, Selective prepared carbon nanomaterials for advanced photocatalytic application in environmental pollutant treatment and hydrogen production, Appl. Catal., B, 2018, 239, 408–424 CrossRef CAS.
- N. Subha, M. Mahalakshmi, S. Monika, P. S. Kumar, V. Preethi, G. Vaishnavi and A. Rajabhuvaneswari, Heterostructured γ-Fe2O3/FeTiO3 magnetic nanocomposite: An efficient visible-light-driven photocatalyst for the degradation of organic dye, Chemosphere, 2022, 306, 135631 CrossRef CAS PubMed.
- D. Qiao, Z. Li, J. Duan and X. He, Adsorption and photocatalytic degradation mechanism of magnetic graphene oxide/ZnO nanocomposites for tetracycline contaminants, Chem. Eng. J., 2020, 400, 125952 CrossRef CAS.
- A. M. Ismael, A. N. El-Shazly, S. E. Gaber, M. M. Rashad, A. H. Kamel and S. S. M. Hassan, Novel TiO2/GO/CuFe2O4 nanocomposite:
A magnetic, reusable and visible-light-driven photocatalyst for efficient photocatalytic removal of chlorinated pesticides from wastewater, RSC Adv., 2020, 10, 34806–34814 RSC.
- M. Ma, Y. Yang, Y. Chen, Y. Ma, P. Lyu, A. Cui, W. Huang, Z. Zhang, Y. Li and F. Si, Photocatalytic degradation of MB dye by the magnetically separable 3D flower-like Fe3O4/SiO2/MnO2/BiOBr-Bi photocatalyst, J. Alloys Compd., 2021, 861, 158256 CrossRef CAS.
- M. Lashgari and M. Ghanimati, Photocatalytic degradation of H2S aqueous media using sulfide nanostructured solid-solution solar-energy-materials to produce hydrogen fuel, J. Hazard. Mater., 2018, 345, 10–17 CrossRef CAS PubMed.
- D. Benz, K. M. Felter, J. Köser, J. Thöming, G. Mul, F. C. Grozema, H. T. Hintzen, M. T. Kreutzer and J. R. Van Ommen, Assessing the role of Pt clusters on TiO2 (P25) on the photocatalytic degradation of acid blue 9 and rhodamine B, J. Phys. Chem. C, 2020, 124, 8269–8278 CrossRef CAS.
- J. Ge, Y. Zhang, Y. J. Heo and S. J. Park, Advanced design and synthesis of composite photocatalysts for the remediation of wastewater: A review, Catalysts, 2019, 9, 122 CrossRef.
- B. O. Orimolade, B. N. Zwane, B. A. Koiki, L. Tshwenya, G. M. Peleyeju, N. Mabuba, M. Zhou and O. A. Arotiba, Solar photoelectrocatalytic degradation of ciprofloxacin at a FTO/BiVO4/MnO2 anode: kinetics, intermediate products and degradation pathway studies, J. Environ. Chem. Eng., 2020, 8, 103607 CrossRef CAS.
- M. J. Abel, V. Archana, A. Pramothkumar, N. Senthilkumar, K. Jothivenkatachalam and J. Joseph, Prince, Investigation on structural, optical and photocatalytic activity of CoMn2O4 nanoparticles prepared via simple co-precipitation method, Phys. B, 2020, 601, 412349 Search PubMed.
- M. Zhu, X. Dong, M. Li, L. Jia, Y. Ma, M. Zhao and H. Cui, Using photo-induced p–n junction interface effect of CoMn2O4/MnO2 oxidase mimetic for colorimetric determination of hydroquinone in seawater, Anal. Chim. Acta, 2021, 1172, 338695 CrossRef CAS PubMed.
- S. Lee, M. Govindan and D. Kim, CoFe-based layered double hydroxide for high removal capacity of hydrogen sulfide under high humid gas stream, Chem. Eng. J., 2021, 416, 127918 CrossRef CAS.
- M. Maroño, I. Ortiz, J. M. Sánchez, L. Alcaraz, F. J. Alguacil and F. A. López, Effective removal of hydrogen sulfide using Mn-based recovered oxides from recycled batteries, Chem. Eng. J., 2021, 419, 129669 CrossRef.
- H. L. Tran, M. S. Kuo, W. D. Yang and Y. C. Huang, Hydrogen sulfide adsorption by thermally treated cobalt(II)-exchanged NaX zeolite, Adsorpt. Sci. Technol., 2016, 34, 275–286 CrossRef CAS.
- J. Zheng, Y. Hu and L. Zhang, Design and construction of a bifunctional magnetically recyclable 3D CoMn2O4/CF hybrid as an adsorptive photocatalyst for the effective removal of contaminants, Phys. Chem. Chem. Phys., 2017, 19, 25044–25051 RSC.
- M. Misra, Sh. R. Chowdhury and N. Singh, TiO2@Au@CoMn2O4 core-shell nanorods for photo-electrochemical and photocatalytic activity for decomposition of toxic organic compounds and photoreduction of Cr6+ ion, J. Alloys Compd., 2020, 824, 153861 CrossRef CAS.
- G. M. Kumar, H. D. Cho, D. J. Lee, J. Ram Kumar, C. Siva, P. Ilanchezhiyan, D. Y. Kim and T. W. Kang, Elevating the charge separation of MgFe2O4 nanostructures by Zn ions for enhanced photocatalytic and photoelectrochemical water splitting, Chemosphere, 2021, 283, 131134 CrossRef CAS PubMed.
- J. Chen, D. Zhao, Z. Diao, M. Wang, L. Guo and S. Shen, Bifunctional modification of graphitic carbon nitride with MgFe2O4 for enhanced photocatalytic hydrogen generation, ACS Appl. Mater. Interfaces, 2015, 7, 18843–18848 CrossRef CAS PubMed.
-
U. I. Gaya, Mechanistic principles of photocatalytic reaction in Heterogeneous photocatalysis using inorganic semiconductor solids, ed. U. I. Gaya, Springer Science & Business Media, Dordrecht, 2014, pp. 73–89 Search PubMed.
- M. Seredych and T. J. Bandosz, Reactive adsorption of hydrogen sulfide on graphite oxide/Zr(OH)4 composites, Chem. Eng. J., 2011, 166, 1032–1038 CrossRef CAS.
- C. Petit, B. Mendoza and T. J. Bandosz, Hydrogen sulfide adsorption on MOFs and MOF/graphite oxide composites, ChemPhysChem, 2010, 11, 3678–3684 CrossRef CAS PubMed.
- J. Jo, S. Lee, J. Gim, J. Song, S. Kim, V. Mathew, M. H. Alfaruqi, S. Kim, J. Lim and J. Kim, Facile synthesis of reduced graphene oxide by modified Hummers’ method as anode material for Li-, Na- and K-ion secondary batteries, R. Soc. Open Sci., 2019, 6, 181978 CrossRef CAS PubMed.
- M. Aghajani, E. Safaei and B. Karimi, Selective and green oxidation of sulfides in water using a new iron(III) bis (phenol) amine complex supported on functionalized graphene oxide, Synth. Met., 2017, 233, 63–73 CrossRef CAS.
- N. Cao and Y. Zhang, Study of reduced graphene oxide preparation by Hummers’ method and related characterization, J. Nanomater., 2015, 2015, 168125, DOI:10.1155/2015/168125.
- S. Park, J. An, J. R. Potts, A. Velamakanni, S. Murali and R. S. Ruoff, Hydrazine-reduction of graphite and graphene oxide, Carbon, 2011, 49, 3019–3023 CrossRef CAS.
- S. Hassanpoor and N. Rouhi, Electrochemical sensor for determination of trace amounts of cadmium(II) in environmental water samples based on MnO2/RGO nanocomposite, Int. J. Environ. Anal. Chem., 2021, 101, 513–532 CrossRef CAS.
- S. N. Tripathi, P. Saini, D. Gupta and V. Choudhary, Electrical and mechanical properties of PMMA/reduced graphene oxide nanocomposites prepared via in situ polymerization, J. Mater. Sci., 2013, 48, 6223–6232 CrossRef CAS.
- H. Chen, L. Ding, K. Zhang, Z. Chen, Y. Lei, Z. Zhou and R. Hou, Preparation of chemically reduced graphene using hydrazine hydrate as the reduction agent and its NO2 sensitivity at room temperature, Int. J. Electrochem. Sci., 2020, 15, 10231–10242 CrossRef.
- Z. Yan, J. Gao, Y. Li, M. Zhang and M. Guo, Hydrothermal synthesis and structure evolution of metal-doped magnesium ferrite from saprolite laterite, RSC Adv., 2015, 5, 92778–92787 RSC.
- D. Ghanbari and M. Salavati-Niasari, Hydrothermal synthesis of different morphologies of MgFe2O4 and magnetic cellulose acetate nanocomposite, Korean J. Chem. Eng., 2015, 32, 903–910 CrossRef CAS.
- S. Ilhan, S. G. Izotova and A. A. Komlev, Synthesis and characterization of MgFe2O4 nanoparticles prepared by hydrothermal decomposition of co-precipitated magnesium and iron hydroxides, Ceram. Int., 2014, 41, 577–585 CrossRef.
- N. Garg, M. Mishra and A. K. Ganguli, Electrochemical and magnetic properties of nanostructured CoMn2O4 and Co2MnO4, RSC Adv., 2015, 5, 84988–84998 RSC.
- A. Chatterjee and S. Wing, Metal–organic framework-derived MnO/CoMn2O4@N-C nanorods with nanoparticle interstitial decoration in core shell structure as improved bifunctional electrocatalytic cathodes for Li–O2 batteries, Electrochim. Acta, 2020, 338, 135809 CrossRef CAS.
- P. Zhang, H. Liu and X. Li, Plasmon-driven engineering in bimetallic CuCo combined with reduced graphene oxide for photocatalytic overall water splitting, Appl. Surf. Sci., 2021, 559, 149865 CrossRef CAS.
- M. Lashgari and M. Ghanimati, A highly efficient nanostructured quinary photocatalyst for hydrogen production, Int. J. Energy Res., 2015, 39, 516–524 CrossRef CAS.
- M. Lashgari and P. Zeinalkhani, Ammonia photosynthesis under ambient conditions using an efficient nanostructured FeS2/CNT solar-energy-material with water feedstock and nitrogen gas, Nano Energy, 2018, 48, 361–368 CrossRef CAS.
- H. Oladipo, C. Garlisi, J. Sa, E. Lewin, K. Al-Ali and G. Palmisano, Unveiling the role of bisulfide in the photocatalytic splitting of H2S in aqueous solutions, Appl. Catal., B, 2020, 270, 118886 CrossRef CAS.
- M. Lashgari and M. Ghanimati, Efficient mesoporous/nanostructured Ag-doped alloy semiconductor for solar hydrogen generation, J. Photonics Energy, 2014, 4, 044099 CrossRef CAS.
- X. Jiao, Y. Qiu, L. Zhang and X. Zhang, Comparison of the characteristic properties of reduced graphene oxides synthesized from natural graphites with different graphitization degrees, RSC Adv., 2017, 7, 52337–52344 RSC.
- P. R. Riley, P. Joshi, H. Penchev, J. Narayan and R. J. Narayan, One-step formation of reduced graphene oxide from insulating polymers induced by laser writing method, Crystals, 2021, 11, 1308 CrossRef CAS.
- D. R. Rout and H. M. Jena, Removal of malachite green dye from aqueous solution using reduced graphene oxide as an adsorbent, Mater. Today Proc., 2021, 47, 1173–1182 CrossRef CAS.
- S. Gurunathan, J. W. Han, A. A. Dayem, V. Eppakayala and J. H. Kim, Oxidative stress-mediated antibacterial activity of graphene oxide and reduced graphene oxide in Pseudomonas aeruginosa, Int. J. Nanomed., 2012, 7, 5901–5914 CrossRef CAS PubMed.
- C. R. Minitha and R. T. Rajendrakumar, Synthesis and characterization of reduced graphene oxide, Adv. Mater. Res., 2013, 678, 56–60, DOI:10.4028/www.scientific.net/AMR.678.56.
- J. R. do Nascimento, M. R. D’Oliveira, A. G. Veiga, C. A. Chagas and M. Schmal, Synthesis of reduced graphene oxide as a support for nano copper and palladium/copper catalysts for selective NO reduction by CO, ACS Omega, 2020, 5, 25568–25581 CrossRef CAS PubMed.
- A. Kumar, A. M. Sadanandhan and S. L. Jain, Silver doped reduced graphene oxide as a promising plasmonic photocatalyst for oxidative coupling of benzylamines under visible light irradiation, New J. Chem., 2019, 43, 9116–9122 RSC.
- S. Nasir, M. Z. Hussein, N. A. Yusof and Z. Zainal, Oil palm waste-based precursors as a renewable and economical carbon sources for the preparation of reduced graphene oxide from graphene oxide, Nanomaterials, 2017, 7, 182 CrossRef PubMed.
- N. N. Rabin, S. Ida, M. R. Karim, M. S. Islam, R. Ohtani, M. Nakamura, M. Koinuma, L. F. Lindoy and S. Hayami, Super dielectric materials of two-dimensional TiO2 or Ca2Nb3O10 nanosheet hybrids with reduced graphene oxide, ACS Omega, 2018, 3, 2074–2083 CrossRef CAS PubMed.
- X. Yuan, H. Wang, Y. Wu, X. Chen, G. Zeng, L. Leng and C. Zhang, A novel SnS2–MgFe2O4/reduced graphene oxide flower-like photocatalyst: solvothermal synthesis, characterization and improved visible-light photocatalytic activity, Catal. Commun., 2015, 61, 62–66 CrossRef CAS.
- S. El Shabrawy, C. Bocker and C. Russel, Crystallization of MgFe2O4 from a glass in the system K2O/B2O3/MgO/P2O5/Fe2O3, Solid State Sci., 2016, 60, 85–91 CrossRef CAS.
- Y. Wang, G. Hua, Y. Cao, Z. Peng and K. Du, One-pot synthesis of pre-reduced graphene oxide for efficient production of high-quality reduced graphene oxide and its lithium storage application, Mater. Chem. Phys., 2021, 265, 124523 CrossRef CAS.
- Y. Zhou, Q. Bao, L. A. L. Tang, Y. Zhong and K. P. Loh, Hydrothermal dehydration for the green reduction of exfoliated graphene oxide to graphene and demonstration of tunable optical limiting properties, Chem. Mater., 2009, 21, 2950–2956 CrossRef CAS.
- J. Feng, Y. Ye, M. Xiao, G. Wu and Y. Ke, Synthetic routes of the reduced graphene oxide, Chem. Pap., 2020, 74, 3767–3783 CrossRef CAS.
- N. Díez, A. Śliwak, S. Gryglewicz, B. Grzyb and G. Gryglewicz, Enhanced reduction of graphene oxide by high-pressure hydrothermal treatment, RSC Adv., 2015, 5, 81831–81837 RSC.
- N. Akiya and P. E. Savage, Roles of water for chemical reactions in high-temperature
water, Chem. Rev., 2002, 102, 2725–2750 CrossRef CAS PubMed.
- T. Kavinkumar, S. Seenivasan, A. T. Sivagurunathan, Y. Kwon and D. H. Kim, Three-dimensional hierarchical core/shell electrodes using highly conformal TiO2 and Co3O4 thin films for high-performance supercapattery devices, ACS Appl. Mater. Interfaces, 2021, 13, 29058–29069 CrossRef CAS PubMed.
- M. Lashgari, S. Afshari, M. Ghanimati and J. Seo, SO2 pollutant conversion to sulfuric acid inside a stand-alone photoelectrochemical reactor: a novel, green, and safe strategy for H2SO4 photosynthesis, J. Ind. Eng. Chem., 2023, 121, 529–535 CrossRef CAS.
- P. Xu, J. Zheng, F. Jing and W. Chu, Influence of support precursor on FeCe–TiO2 for selective catalytic reduction of NO with ammonia, Mol. Catal., 2021, 508, 111586 CrossRef CAS.
- L. Li, P. Ma, S. Hussain, L. Jia, D. Lin, X. Yin, Y. Lin, Z. Cheng and L. Wang, FeS2/carbon hybrids on carbon cloth: a highly efficient and stable counter electrode for dye-sensitized solar cells, Sustainable Energy Fuels, 2019, 3, 1749–1756 RSC.
- M. Adel, M. A. Ahmed and A. A. Mohamed, Synthesis and characterization of magnetically separable and recyclable crumbled MgFe2O4/reduced graphene oxide nanoparticles for removal of methylene blue dye from aqueous solutions, J. Phys. Chem. Solids, 2021, 149, 109760 CrossRef CAS.
-
M. Lashgari, Fundamental aspects of CO2 transformation into C/H/O based fuels/chemicals, Nanomaterials for CO2 Capture, Storage, Conversion and Utilization, Elsevier, 2021, pp. 283–305 DOI:10.1016/B978-0-12-822894-4.00015-0.
- W. Zhao, Q. Zhong, J. Ding, Z. Deng, L. Guo and F. Song, Enhanced catalytic ozonation over reduced spinel CoMn2O4 for NOx removal: active site and mechanism analysis, RSC Adv., 2016, 6, 115213 RSC.
- Y. J. Heo and S. J. Park, Facile synthesis of MgO-modified carbon adsorbents with microwave-assisted methods: effect of MgO particles and porosities on CO2 Capture, Sci. Rep., 2017, 7, 5653 CrossRef PubMed.
- R. K. N. Kutty, P. R. Kasturi, J. Jaganath, S. Padmanapan, Y. S. Lee, D. Meyrick and R. K. Selvan, Structural and magnetic properties of CoMn2O4 synthesized by auto combustion method, J. Mater. Sci.: Mater. Electron., 2019, 30, 975–981 CrossRef CAS.
- A. F. Cabrera, C. R. Torres, S. G. Marchetti and S. J. Stewart, Degradation of methylene blue dye under dark and visible light conditions in presence of hybrid composites of nanostructured MgFe2O4 ferrites and oxygenated organic compounds, J. Environ. Chem. Eng., 2020, 8, 104274 CrossRef CAS.
-
F. Fiorillo, G. Bertotti, C. Appino and M. Pasquale, Soft magnetic materials, ed. J. G. Webster, in Wiley Encyclopedia of Electrical and Electronics Engineering, John Wiley & Sons, Inc, 2016, pp.1–42 Search PubMed.
- Z. Wu, K. Pei, L. Xing, X. Yu, W. You and R. Che, Enhanced microwave absorption performance from magnetic coupling of magnetic nanoparticles suspended within hierarchically tubular composite, Adv. Funct. Mater., 2019, 29, 1901448 CrossRef.
-
J. M. Galloway, Biotemplating arrays of nanomagnets using the biomineralisation protein Mms6, PhD thesis, University of Leeds, 2012.
- J. Wei, Z. Chen and Z. Tong, Engineering Z-scheme silver oxide/bismuth tungstate heterostructure incorporated reduced graphene oxide with superior visible-light photocatalytic activity, J. Colloid Interface Sci., 2021, 596, 22–33 CrossRef CAS PubMed.
-
J. A. Dean, Lange's Handbook of Chemistry, McGraw-Hill Inc, New York, 1999, Table 8.6 Search PubMed.
- H. L. Tran, M. S. Kuo, W. D. Yang and Y. C. Huang, Hydrogen sulfide adsorption by thermally treated cobalt(II)-exchanged NaX zeolite, Ads. Sci. Technol., 2016, 34, 275–286 CrossRef CAS.
- L. F. Guo, K. L. Pan, H. M. Lee and M. B. Chang, High-temperature gaseous H2S removal by Zn–Mn-based sorbent, Ind. Eng. Chem. Res., 2015, 54, 11040–11047 CrossRef CAS.
- K. Polychronopoulou, F. C. Galisteo, M. L. Granados, J. L. G. Fierro, T. Bakas and A. M. Efstathiou, Novel Fe–Mn–Zn–Ti–O mixed-metal oxides for the low-temperature removal of H2S from gas streams in the presence of H2, CO2, and H2O, J. Catal., 2005, 236, 205–220 CrossRef CAS.
- J. C. Hu, S. Sun, W. Xia, J. Wu, H. Liu and F. Wang, A biomimetic self-assembled cobaloxime@CdS/rGO hybrid for boosting photocatalytic H2 production, Chem. Commun., 2019, 55, 14490–14493 RSC.
- M. Lashgari, N. Shafizadeh and P. Zeinalkhani, A nanocomposite p-type semiconductor film for possible application in solar cells: photo-electrochemical studies, Sol. Energy Mater. Sol. Cells, 2015, 137, 274–279 CrossRef CAS.
Footnote |
† Electronic supplementary information (ESI) available. See DOI: 10.1039/d3ma00668a |
|
This journal is © The Royal Society of Chemistry 2023 |