DOI:
10.1039/D2MA00909A
(Paper)
Mater. Adv., 2023,
4, 504-514
Alkoxy phosphonic acid-functionalized conjugated microporous polymers for efficient and multi-environmental proton conduction†
Received
19th September 2022
, Accepted 9th November 2022
First published on 15th November 2022
Abstract
Proton-conducting materials are key components of proton exchange membrane fuel cells that can convert chemical fuel into electrical energy directly, efficiently, and without pollution. Despite the creation of various proton-conducting polyelectrolytes over the past decade, there is still a lack of robust electrolytes that can simultaneously satisfy high proton conductivity and multi-environmental conditions. Herein, we designed and synthesized a series of alkoxy phosphonic acid-functionalized conjugated microporous polymers (CMPs) through side-chain engineering and Sonogashira–Hagihara cross coupling. With superior stability, hydrophilic nature and high side-chain mobility, the phosphonated CMPs further serve as robust hosts to accommodate a large number of H3PO4 molecules through hydrogen-bonding, and exhibit high proton conductivity in pelletized powder samples under multi-environment conditions, such as wide temperature range anhydrous proton conductivities of 2.15 × 10−2 and 1.15 × 10−5 S cm−1 at 130 and −40 °C, respectively, and a wide humidity range proton conductivity of 1.87 × 10−2 and 9.93 × 10−2 S cm−1 at 30 °C under 32% relative humidity and 90 °C under 98% relative humidity, respectively. To the best of our knowledge, this is the first example of constructing multi-environmentally adaptable porous organic polymer proton-conducting materials for both humid and anhydrous conditions within a wide temperature range (−40 to 130 °C).
Introduction
As the core component of proton exchange membrane fuel cells (PEMFCs), proton conducting materials play a vital role in the realization of green and advanced energy conversion. Typically, proton conduction can be divided into water-mediated, that is, proton conduction under relative humidity (RH), and anhydrous proton conduction. Commercially available Nafion™ has been able to achieve efficient proton conduction (10−2 to 10−1 S cm−1) under a high relative humidity (98% RH).1–5 The disadvantage, however, is that proton conduction with the maximum effect can only be achieved in a narrow range of operating temperature below 80 °C. At a higher operating temperature, it is always accompanied by the leakage of inner water medium and other guests, which leads to significantly lower proton conductivity, higher cost, and insufficient lifetime.1,6–10 Similarly, serious damage to the hydrated PEMs will be induced by freeze/thaw cycles on operation below −5 °C.11 For anhydrous proton conduction, operating above 100 °C has the advantage of fast kinetics.1,12–14 In the meantime, on-board fuel cells not only require high proton conductivity at medium and high temperatures, but also require appropriate functioning for start-up at room temperature, even in subzero climates, such as in high-altitude drones.11,15,16 Therefore, designing universal proton-conducting materials that can perform well over a wide temperature range including at subzero temperatures, and that are versatile under both humid and anhydrous conditions, is a matter of great urgency.17–19
In recent years, porous materials such as metal–organic frameworks (MOFs),20–23 covalent organic frameworks (COFs),24–26 hydrogen-bonded organic frameworks (HOFs),27 and porous organic polymers (POPs),28–30 shown to possess flexibly functionalized frameworks together with concurrent control of pore size, shape, and interface, have gradually been branching out into the field, whether as a support for humid proton conduction or for anhydrous proton conduction. Conjugated microporous polymers (CMPs), due to their synthetic simplicity, solid structure, and tunable porosity, have attracted much attention and been applied in various fields, such as gas storage and separation,31,32 adsorption and encapsulation of chemicals,33,34 heterogeneous catalysis,35,36 photoredox catalysis,37,38 light emittance,39,40 and so on. So far, for proton conduction, among POPs doped with an additional proton source such as acid, their highest humid and anhydrous proton conductivities are revealed as ∼10−1 S cm−1 for SPAF-1 and ∼10−3 S cm−1 for H3PO4@CMP-F6-60%, respectively.27,28,30,41–45 For operation above 80 °C and below −5 °C, the research and development of guest-induced anhydrous proton-conductors is the top priority. Thus far, PA@TpBpy-MC and H3PO4@TPB-DMeTP-COF have been reported to achieve high anhydrous proton conductivities of ∼10−4 and ∼10−1 S cm−1 at −40 °C and 160 °C, respectively.18,25 Under humid conditions, the proton conductivities of some composite membranes have reached up to 10−1 S cm−1.46–48 However, there are rare reports on conductive materials with high efficiency that simultaneously achieve applications under humid and anhydrous conditions. In the reported works, PA@Tp-Azo exhibits an anhydrous proton conductivity of 9.9 × 10−4 S cm−1 at 67 °C and a humid proton conductivity of 6.70 × 10−5 S cm−1 at 59 °C under 98% RH, and MPOPS-1 displays proton conductivities of 1.49 × 10−5 and 3.07 × 10−2 S cm−1 at 77 °C under anhydrous and humid conditions, respectively.30,49 Notably, there is no further report on novel universal proton-conducting materials that are applicable to a wide temperature range under both humid and anhydrous conditions.
Typically, phosphoric acid is extensively used as a proton carrier due to its high dissociation constant, and non-volatile, and non-toxic properties.50 However, avoiding the leaching of phosphoric acid under humid conditions and maintaining its efficient proton transfer in a wide temperature range are the real problems to be solved. Herein, we successfully synthesized a series of hydrophilic phosphonic acid group-functionalized CMPs. The phosphonated CMP frameworks show satisfactory acid resistance and thermal stability, and exhibit high porosity. Upon doping with H3PO4, the composited CMPs, denoted as CMP-P-H and CMP-Cx-P-H, reveal stable and efficient proton conduction under complex harsh conditions, including anhydrous 130 and −40 °C, and high humidity (98% RH) 90 °C. The fast proton transfer is mainly attributed to the multi-point triggered formation of hydrogen-bonding interactions between the phosphonic acid groups anchored within CMP frameworks and the doped guest H3PO4 molecules. The hydrogen-bonding interactions have been illustrated using the XPS and FT-IR spectra.
Experimental
Materials and methods
All the chemicals and solvents used in the syntheses were commercially purchased and of reagent grade, and can be used without further purification. 2,5-Dibromohydroquinone and 1,3,5-triethynylbenzene were purchased from Heowns Biotech LLC (Tianjin, China). 1,2-Dibromoethane, 1,4-dibromobutane and 1,6-dibromohexane were purchased from Dibo Biotech Co., Ltd (Shanghai, China). Triethyl phosphite and Pd(PPh3)2Cl2 were purchased from Wanqing Chemical Glassware Instrument Co., Ltd (Nanjing, China). All the solvents such as acetonitrile, triethylamine, dichloromethane, acetone, methanol, and N,N-dimethylformamide were purchased from Sinopharm (Beijing, China).
All samples were characterized and analyzed according to conventional techniques. Powder X-ray diffraction (PXRD) patterns were collected on a Bruker D8 Advance diffractometer using Cu Kα radiation. Fourier transform infrared (FT-IR) spectra were obtained on a Thermo Fisher Scientific Optics NICOLETIS10 FT-IR spectrometer with a Universal ATR accessory within the range of 4000 to 500 cm−1. Liquid-state 1H nuclear magnetic resonance (NMR) spectra were obtained on a Bruker Advance III instrument with a AS500 magnet equipped with a cryoprobe (500 MHz). Liquid-state 13C nuclear magnetic resonance spectra were obtained on a Bruker Advance III instrument with a AS500 magnet equipped with a cryoprobe (125 MHz). Liquid-state 31P nuclear magnetic resonance spectra were obtained on a Bruker Advance III instrument with a AS500 magnet equipped with a cryoprobe (202.41 MHz). Solid-state 13C cross-polarization magic-angle-spinning (CP/MAS) NMR spectra were recorded on a JEOL JNM-ECA 400 MHz, 4.0 mm rotor, MAS of 10 kHz, and recycle delay of 1 s. High-resolution mass spectrometry (HRMS) mass spectra were obtained on a Baird Acquity UPLC/XEVO G2-XS QTOF using CHCl3 as a solvent. Scanning electron microscopy (SEM) images were collected using a JSM-IT500HR system. Transmission electron microscopy (TEM) images were collected using a JEM-2100, JEOL. Thermogravimetric analysis (TGA) was performed using a NETZSCH STA 449F5 under flowing N2 (60 mL min−1) with a 10 K min−1 ramp rate. Samples were heated in a platinum pan from 50 °C to 900 °C (10 °C min−1). N2 adsorption and desorption measurements were performed at 77 K using BEL (MicrotracBEL Corp, Japan), and before the gas adsorption measurements, all the solids were dried at 80 °C under vacuum in a drying oven for 24 h to remove the residual solvent, then all the samples were degassed under vacuum at 100 °C with BELPREP VAC III for 12 h to afford the sample for sorption analysis. The pore size distributions of the samples in this work were estimated using Nonlocal Density Functional Theory (NLDFT) based on the model of N2/77 K on graphitic carbon with slit pores and the method of Tikhonov regularization. Water vapor adsorption and desorption measurements were performed at 298 K using BEL (MicrotracBEL Corp, Japan). The water contact angles (WACs) were measured on a goniometer (JC2000C, Japan) equipped with video capture. X-Ray photoelectron spectroscopy (XPS) spectra were recorded with a kratos axis supra™ of Shimadzu.
Synthesis of CMPs
A series of phosphonic acid group-functionalized CMPs were synthesized by Sonogashira–Hagihara cross coupling of 1,3,5-triethynyltriphenylbenzene with different phosphonic acid group anchored monomers of different lengths. The corresponding CMPs were named CMP-P and CMP-Cx-P (x = 2, 4, and 6, depending on the number of C atoms in the alkoxy phosphonic acid group side chain; P stands for phosphonic acid groups), respectively (Scheme 1a). Note that there is no alkoxy chain between the framework of CMP-P and the phosphonic acid groups. Monomer S3/6/9/12 (0.50 mmol), 1,3,5-triethynyltriphenylbenzene (0.33 mmol), CuI (0.04 mmol) and Pd(PPh3)4 (0.02 mmol) were added into NEt3/DMF (1
:
1, v
:
v), respectively. The mixture was stirred for 60 h at 80 °C under an argon atmosphere and was allowed to cool to room temperature. The crude was washed with water, methanol, dichloromethane and acetone. Then soxhlet extraction from methanol for 48 h was carried out to ensure there was no unreacted monomer, soaked in dry acetone for 12 h and dried at 80 °C under vacuum for 12 h to give CMP-P and CMP-Cx-P as yellow powders. Furthermore, CMP-C2S-P with smaller pores was also obtained by Sonogashira–Hagihara cross coupling of 1,3,5-triethynylbenzene with monomer S3 (see the ESI†). CMP-U, the unfunctionalized parent CMP without the alkoxy phosphonic acid group side chains, was prepared by Sonogashira–Hagihara cross coupling of 1,3,5-triethynyltriphenylbenzene with 1,4-dibromobenzene (see the ESI†).
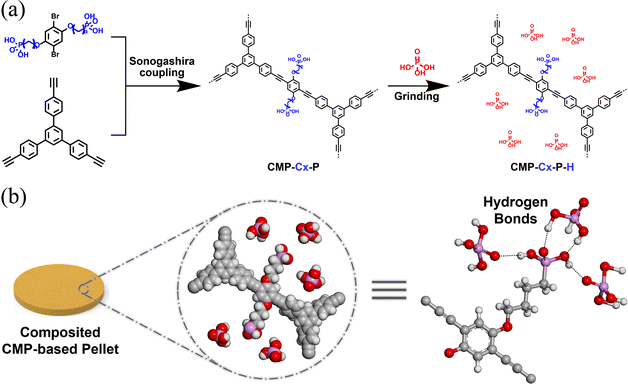 |
| Scheme 1 (a) Illustration of the synthesis of the phosphonated CMPs composited with H3PO4. (b) Demonstrating the assembly of hydrogen-bonding networks. Pink, P; red, O; white, H; grey, C. | |
Synthesis of H3PO4 doped CMPs
Doping non-volatile liquid acids into porous materials is a promising solution for constructing proton-conducting electrolytes, and neat phosphoric acid is the most ideal proton carrier due to its high dissociation constant, non-volatile and green properties. Firstly, the dried powder samples of CMP-P and CMP-Cx-P (20 mg) were weighed into an agate mortar. Then we doped different amounts of H3PO4 into the above mortar. After smooth manual grinding in a mortar with a pestle, the solids were collected and dried at 100 °C under vacuum for 12 h. The H3PO4 doped samples were denoted as CMP-P-H and CMP-Cx-P-H (H represents the percentage of doped H3PO4 in the total mass), respectively (Scheme 1a). CMP-C2S-P-H and CMP-U-H were prepared in the same way.
Proton conductivity measurements
For anhydrous proton conduction, first, the pre-dry CMP powders and H3PO4 doped CMP powders were compressed using a powder tableting machine in the glove box under an argon atmosphere with O2 and H2O less than 0.01 ppm at a pressure of 0.2 MPa for about 30 minutes to obtain composite CMP-based electrolyte cylindrical pellets with a diameter of 5 mm. Then, the above pellets were sandwiched between two stainless steel electrodes, respectively, assembling into coin cells. The operating temperatures were controlled by one oven. Alternating-current (AC) impedance spectroscopy measurements were performed using a Solartron1296A electrochemistry workstation over a frequency range of 1 to 1 MHz and a temperature range of −40 to 130 °C. For humid proton conduction, CMP powders and H3PO4 doped CMP powders were pressed into cylindrical pellets with a diameter of 5 mm at a pressure of 0.2 MPa for about 30 minutes. These pellets were sandwiched between two platinum electrodes, and then were encapsulated in a glass bottle with a certain relative humidity, which was controlled by a different saturated salt solution. The measurements were similar to those of anhydrous proton conduction, except that the operating temperature range was changed to 30–90 °C.
The proton conductivities were calculated by the equation:
where
σ (S cm
−1) is the ionic conductivity,
L (cm) is the thickness of the electrolyte pellet,
S (cm
2) is the area of the electrode, and
R (Ω) is the resistance value that can be directly obtained from the profiles in the recorded AC impedance spectra.
The activation energy is further calculated by a linear fit of the Arrhenius equation:
where
Ea (eV) is the transport activation energy,
k is the Boltzmann constant,
T (K) is the temperature, and
A is a pre-exponential factor.
Results and discussion
Characterization of CMP-Cx-P
A series of stable phosphonic acid group-functionalized CMPs were obtained via Sonogashira–Hagihara cross coupling of 1,3,5-triethynyltriphenylbenzene with four phosphonic acid group anchored monomers of different lengths and named as CMP-P and CMP-Cx-P (x = 2, 4, and 6; P stands for phosphonic acid groups), respectively. The successful polymerization reaction and the formation of CMPs could be proved by the following experiments.
First, the formation of yellow insoluble solids roughly indicates the polymer state of the products. Second, powder X-ray diffraction (PXRD) measurements show that the diffraction peaks of monomer S3 and 1,3,5-triethynyltriphenylbenzene disappear in the as-prepared CMPs (Fig. S1, ESI†). This phenomenon indicates the disordered structure of CMP-C2-P.51 Third, the results of Fourier transform infrared (FT-IR) spectra (Fig. S2–S4, ESI†) also confirm the successful preparation of CMP-Cx-P and CMP-P. In Fig. S2 (ESI†), monomer S3 shows strong peaks at 3265 cm−1 and 537 cm−1, which correspond to the stretching vibrational band of terminal alkynes –C
C–H and C–Br, while a weak peak could be observed at 2108 cm−1, corresponding to alkynes –C
C–.51 For 1,3,5-triethynyltriphenylbenzene, the stretching vibrational band of phosphonic acid groups P
O is located at approximately 940 cm−1. In terms of CMP-C2-P, the disappearance of terminal alkynes –C
C–H and C–Br, the retention of alkynes –C
C– (2168 cm−1) and phosphonic acid groups P
O (919 cm−1) reveal the success of the coupling reaction. Lastly, the presence of C atoms in various chemical environments in the solid-state 13C cross-polarization magic-angle-spinning (CP/MAS) NMR spectra (Fig. 1a and Fig. S5, ESI†) proves the structural integrity of CMP-Cx-P and CMP-P. Moreover, a scanning electron microscope (SEM) and transmission electron microscope (TEM) were utilized to observe the morphology and nanostructure of CMP-C2-P (Fig. 1c and Fig. S6, ESI†). SEM images reveal that CMP-C2-P possesses a stacked bulk bumpy coral-like morphology within a dimension range of 500 to 3000 nm. TEM images reveal that CMP-C2-P exhibits stacked block layered morphology.
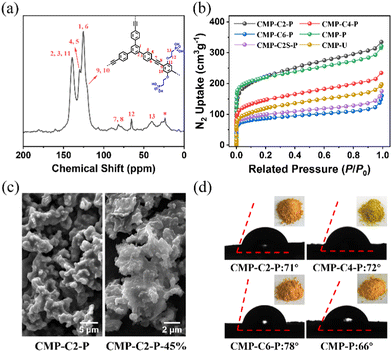 |
| Fig. 1 (a) 13C CP/MAS NMR spectrum of CMP-C2-P. Asterisks denote spinning sidebands. (b) N2 adsorption isotherms of CMPs at 77 K. (c) SEM images of CMP-C2-P and CMP-C2-P-45%. (d) Water contact angles of CMP-C2/C4/C6-P and CMP-P. | |
The thermal stability of CMPs was measured by thermogravimetric analysis (TGA) under a nitrogen atmosphere. As shown in Fig. S7 (ESI†), CMP-Cx-P shows no obvious weight loss before 155 °C, which means that the structure has excellent thermal stability until 155 °C. And the first weightlessness may be attributed to the partial decomposition of the phosphonic acid groups anchored in the CMPs or the release of free H2O molecules. In addition, we further heat-treated CMP-C2-P at 155 °C for 12 h, in which the structural integrity of the heated CMP-C2-P is hardly changed as evidenced by the results in the PXRD pattern and FT-IR spectrum (Fig. S8 and S9, ESI†). The chemical stability of CMP-C2-P in water, 60% HNO3, and 40% HCl for 24 hours was tested by FT-IR and N2 adsorption measurement (Fig. S10–S12, ESI†). The unchanged peaks of alkynes –C
C– and phosphonic acid groups P
O in the FT-IR spectra reveal the steady state of CMP-C2-P. The surface area of CMP-C2-P soaked in 60% HNO3 is 563 m2 g−1, demonstrating its good chemical stability in acid as well.
Nitrogen adsorption measurements for CMPs were performed at 77 K to explore their porosity. The type-I isotherm curves and sharp absorption at low pressure (P/P0 < 0.05) demonstrates the microporous structure of CMP-Cx-P.52 The increased N2 uptake above a partial pressure of 0.2 and the rise in uptake at P/P0 > 0.8 in the isotherm for CMPs may be due to interparticulate porosity associated with the complex meso- and macro-structure of the samples.51 The Brunauer–Emmett–Teller (BET) surface areas of them are 648, 443, 297, 622, 311, and 421 m2 g−1 for CMP-C2/C4/C6-P, CMP-P, CMP-C2S-P and CMP-U, respectively (Fig. 1b). The gradual decrease in surface area of CMP-Cx-P is attributed to the different length of the alkoxy phosphonic acid group side chains. Moreover, nonlocal density functional theory (NLDFT) calculation provides the pore size distribution of CMPs, and the pore sizes of CMP-C2/C4/C6-P, CMP-P, CMP-C2S-P and CMP-U are centred at 0.79–2.14, 0.99–2.04, 1.11–1.96, 1.40–2.34, 1.11–1.88, and 0.79–2.34 nm, respectively (Fig. S12, ESI†). The PXRD data and NLDFT pore size distributions prove that these networks are indeed completely amorphous, consistent with the literature results.51 Water vapor adsorption measurements were utilized to reveal the water vapor adsorption capacity of CMPs (Fig. S14, ESI†). According to the isotherms, the water vapor uptake of CMP-C2/C4/C6-P is 47, 47, and 40 cm3 g−1, and the hydrogen-bonding interaction (P
O⋯H–O) of the oxygen atoms on the wall, promotes the uptake of H2O molecules.24 Water contact angle measurements further confirm the hydrophilicity for CMP-Cx-P (Fig. 1d).
In addition, the electrical conductivity of CMP-Cx-P was measured (Fig. S15, ESI†). The electronic conductivities of CMP-C2/C4/C6-P and CMP-P are 8.74 × 10−6, 8.86 × 10−6, 6.70 × 10−6, and 8.28 × 10−6 S m−1, respectively, implying that these CMPs with disordered structures possess poor electrical conductivity.
Characterization of CMP-Cx-P-H
The successful anchoring of phosphonic acid groups, high porosity and good thermal stability and acid-resistance of CMP-Cx-P enable them to act as anhydrous proton-conducting materials. Afterwards, different qualities of H3PO4 were doped into CMP-Cx-P by manual grinding. The corresponding CMPs were defined as CMP-Cx-P-H (H represents the percentage of doped H3PO4 in the total mass). CMP-Cx-P-H and CMP-P-H show similar PXRD patterns to those of the initial CMP-Cx-P and CMP-P, implying no change in the structures (Fig. S16, ESI†). Besides, the successful doping of H3PO4 in the channel of CMP-Cx-P is further confirmed by FT-IR spectra, TGA and N2 adsorption measurements. The FT-IR spectra reveal that alkynes –C
C– and phosphonic acid groups P
O are well maintained (Fig. S17, ESI†), and furthermore, the band of P
O (∼970 cm−1) became more intense and distinct as the amount of doped H3PO4 increases. The BET surface area of CMP-C2/C4/C6-P-45%, CMP-P-45%, and CMP-U-45% sharply reduced to 13, 3, 12, 5, and 7 m2 g−1, respectively (Fig. S18 and S19, ESI†). Note that after the removal of H3PO4, CMP-C2-P-45%, shows a BET surface area of 458 m2 g−1 (Fig. S12, ESI†), which remains above 70% compared with CMP-C2-P, showing structural stability in the doping of H3PO4. As shown by TGA curves, phosphoric acid, CMP-C2-P, and CMP-C2-P-45% undergo weight loss at 120, 155, and 140 °C, respectively (Fig. S20, ESI†). These results suggest that the pores of CMP-Cx-P-H are occupied by guest H3PO4 molecules. The introduction of H3PO4 leads to a higher water vapor uptake (Fig. S14, ESI†), and the uptake of water vapor for CMP-C2/C4/C6-P-45% is 425, 384, and 417 cm3 g−1, respectively. This trend corresponds to the water contact angle tests (Fig. 1d and Fig. S21, ESI†). After doping with H3PO4, SEM and TEM images show that CMP-C2-P-45% retains the initial morphology and structure (Fig. 1c and Fig. S6, ESI†). These results hint there is no excess H3PO4 on the surface of CMP-C2-P-45%.
High temperature anhydrous proton conduction
We prepared the cylindrical pellets of these samples with a diameter of 5 mm and a thickness of around 0.4–0.6 mm via pressing the pre-dried CMP powders at a pressure of 0.2 MPa for 30 min under a nitrogen atmosphere, and investigated the proton conductivities over various temperatures from 30 to 130 °C by alternating-current (AC) impedance spectroscopy under anhydrous conditions.
As shown in the Nyquist plots, the resistance values of CMP-Cx-P-H and CMP-P-H could be directly obtained to calculate the corresponding proton conductivities. To study the proton conductivities of CMPs at various temperatures, we take CMP-C2-P-H as an example. CMP-C2-P is almost an insulator, exhibiting a high resistance value (R = ∼108 Ω) even at 130 °C (Fig. S23, ESI†). By comparison, the proton conductivity of CMP-C2-P-15% (4.90 × 10−4 S cm−1) is at least 5 orders of magnitude higher than that of CMP-C2-P under similar conditions (Fig. S24, ESI†). Moreover, CMP-C2-P-30%/45%/60% reveals increasingly high proton conductivities of 2.10 × 10−3, 1.12 × 10−2, and 2.15 × 10−2 S cm−1 at 130 °C, respectively (Fig. 2a and Fig. S24, ESI†). Notably, the proton conductivity of CMP-C2-P-45% is nearly 5 times that of our previous reported H3PO4@CMP-F6-45% (2.83 × 10−3 S cm−1), and is competitive among the reported porous materials (Fig. 2e and Table S1, ESI†).11,18,25,26,29,30,42,49,53–57 In our previous work, we introduced functional hydrophobic groups into the channel of CMPs to promote anhydrous proton conductivity.42 By contrast, in this work, the pre-designed hydrophilic phosphonic acid group anchored CMPs exhibit more efficient anhydrous proton conduction. Furthermore, the activation energies of CMP-C2-P-15%/30%/45%/60% obtained within 30–130 °C were 0.73, 0.63, 0.47, and 0.36 eV, respectively (Fig. 2b). Based on these findings, with the increase of doped H3PO4, the proton transfer mechanism of CMP-Cx-P-H changed from Vehicular mechanisms (Ea > 0.4 eV) to Grotthuss mechanisms (Ea < 0.4 eV).58,59 Hence, due to the remarkable hydrogen-bonding networks formed by the anchored phosphonic acid groups and guest H3PO4 molecules, H2O molecules, we solve the leaching of H3PO4 as much as possible, thereby achieving efficient proton hopping.25
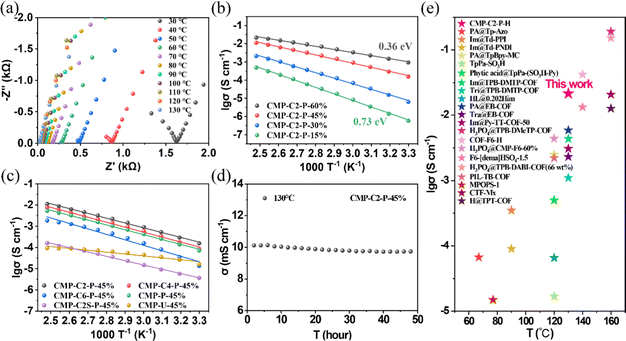 |
| Fig. 2 (a) Nyquist plots for CMP-C2-P-45% at 30–130 °C under anhydrous conditions. (b) Arrhenius plots for CMP-C2-P-H with a different amount of H3PO4 under anhydrous conditions. (c) Arrhenius plots for CMP-C2/C4/C6-P-45%, CMP-P-45%, CMP-C2S-P-45%, and CMP-U-45% under anhydrous conditions. (d) Long-period test for CMP-C2-P-45% at 130 °C under anhydrous conditions. (e) Comparison of anhydrous proton conductivities in the reported materials. | |
Similar to CMP-C2-P-H, the proton conductivities of CMP-C4/C6-P-H decorated with longer alkoxy side chains were studied. For CMP-C4/C6-P-45%, the proton conductivities at 130 °C are 7.76 × 10−3, and 1.87 × 10−3 S cm−1, respectively. It can be concluded that CMP-C4/C6-P-H shows lower proton conductivity compared with CMP-C2-P-H (Fig. 2c and Fig. S25, ESI†), which is due to longer side chains blocking the size-limited nanopores. Meanwhile, at 130 °C, CMP-P-45% (σ = 5.29 × 10−3 S cm−1) reveals a lower proton conductivity than those of CMP-C2/C4-P-45% and a higher proton conductivity than that of CMP-C6-P-45%, which may be due to the lack of flexible alkoxy chains in CMP-P structures. Moreover, under the same conditions, the proton conductivity of CMP-C2S-P-45% reaches 1.62 × 10−4 S cm−1, while CMP-U-45% exhibits the lowest proton conductivity of 9.44 × 10−5 S cm−1 (Fig. 2c and Fig. S25, ESI†), which is nearly 2 orders of magnitude lower than that of CMP-C2-P-45%. Therefore, we draw the conclusions that (i) large decorated pores and suitable length of the side chains are both needed to achieve efficient proton conduction; (ii) the bottom-up synthesized hydrophilic phosphonic acid groups anchored in the frameworks contribute significantly to proton transport.
At last, CMP-C2-P-45% shows an excellent stability in the long-period test and cycling test. After 48 hours at 130 °C, the proton conductivity of CMP-C2-P-45% (9.81 × 10−3 S cm−1) only decreased by 6.2% per day, and the value tended to be constant after 20 hours (Fig. 2d). The proton transport capability of the second cycling test is consistent with the initial test (Fig. S26, ESI†). These results indicate the excellent thermal and electrochemical stability of CMP-Cx-P-H, confirming that guest H3PO4 molecules are anchored inside the structure. Elevating the temperature above 140 °C may cause a decrease in proton conductivity, which is due to the partial decomposition of H3PO4 molecules (Fig. S20, ESI†).
Subzero temperature anhydrous proton conduction
Inspired by the high anhydrous proton conductivities of CMP-C2-P-60% from room temperature to 130 °C (9.29 × 10−4 and 2.15 × 10−2 S cm−1 at 30 and 130 °C, respectively) and the solid hydrogen-bonding networks, we further measured the proton conductivity of CMP-Cx-P-H and CMP-P-H within a low temperature range from −40 to 0 °C. The anhydrous proton conductivity of CMP-C2-P-60% at −40 °C is 1.15 × 10−5 S cm−1, and the activation energy over the temperature range of −40 to 0 °C is 0.55 eV (Fig. 3a and b), which demonstrates a good proton transport efficiency compared to other porous materials (Fig. 3d and Table S1, ESI†).11,18,60,61 Note that in the plot of ln(σT) against 1000 T−1 between −40 and 130 °C, CMP-C2-P-60% exhibits a nonlinear relationship across the entire temperature range (Fig. S27, ESI†). This can be attributed to a weak structural change which was commonly observed in similar porous materials.57,60–63 Additionally, CMP-C2/C4/C6-P-45% and CMP-P-45% obtain proton conductivities of 2.33 × 10−7, 9.61 × 10−8, 1.04 × 10−8, and 2.68 × 10−8 S cm−1 at −40 °C, respectively (Fig. S28, ESI†). For comparison, H3PO4@CMP-F6-45% was also measured under the same conditions (Fig. S29, ESI†). The proton conductivity of H3PO4@CMP-F6-45% (∼10−10 S cm−1) is 3 orders of magnitude lower than that of CMP-C2-P-45%. This result is possibly due to the strong P
O⋯H–O hydrogen-bonds formed inside CMP-Cx-P-H, which makes the hydrogen-bonding network system more stable within the structure. In particular, CMP-C2-P-60% also performs very well in the long-period test (Fig. 3c). In conclusion, CMP-Cx-P-H can be applied within a wide temperature range, subzero temperature—room temperature—high temperature.
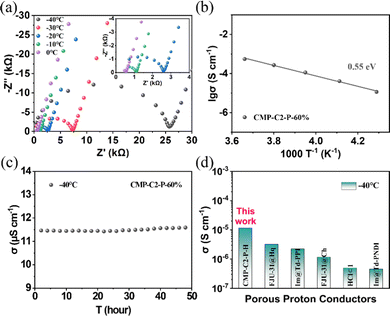 |
| Fig. 3 (a) Nyquist plots for CMP-C2-P-60% at –40 to 0 °C under anhydrous conditions. (b) Arrhenius plots for CMP-C2-P-60% under anhydrous conditions. (c) Long-period test for CMP-C2-P-60% at −40 °C under anhydrous conditions. (d) Comparison of anhydrous proton conductivities in the reported materials measured at −40 °C. | |
Humid proton conduction
Considering the hydrophilic phosphonic acid groups anchored in the pores of CMP-Cx-P, we further measured the proton conductions of CMP-C2-P and CMP-C2-P-H under humidity conditions. Cylindrical pellets of CMP-C2-P and CMP-C2-P-H with a diameter of 5 mm and a thickness of around 0.5–0.7 mm were prepared. The pellets were sealed in a glass bottle with a certain relative humidity, and were then measured from 30 to 90 °C.
As given by Nyquist plots, CMP-C2-P possesses the proton conductivities of 8.95 × 10−9, 2.77 × 10−7, and 2.17 × 10−5 S cm−1 under 32%, 56%, and 84% RH at 30 °C, respectively (Fig. 4b and Fig. S30, ESI†). In contrast, CMP-C2-P-45% exhibits proton conductivities of 1.87 × 10−2, 3.28 × 10−2, and 4.86 × 10−2 S cm−1 under 32%, 56%, and 84% RH at 30 °C, respectively (Fig. 4b and Fig. S31, ESI†). Furthermore, at different temperatures, the proton conductivities of CMP-C2-P are obtained under 98% RH (1.84 × 10−4, 7.59 × 10−4, and 9.89 × 10−3 S cm−1 at 30, 60, and 90 °C, respectively) (Fig. S32, ESI†). Ulteriorly, under 98% RH, the proton conductivities of CMP-C2-45% are 5.87 × 10−2, 7.99 × 10−2, and 9.93 × 10−2 S cm−1 at 30, 60 and 90 °C, respectively (Fig. 4a). It is worth mentioning that (i) CMP-C2-P was capable of possessing a relatively high intrinsic proton conductivity after adsorbing water. Although CMP-C2-P obtains negligible anhydrous proton conductivity, the proton conductivity value increases prominently under humid conditions; (ii) the humid proton conductivity of CMP-C2-45% under 98% RH at 90 °C is 31 times the anhydrous proton conductivity of CMP-C2-45% (3.23 × 10−3 S cm−1 at 90 °C). These observations illustrates that (i) the hydration of the CMPs under high humidity is of importance for proton conduction of these non-intrinsic proton-conducting materials;24,64 (ii) the obvious superiority of CMP-Cx-P-H over the initial CMP-Cx-P can be attributed to the dense hydrogen-bonding networks involving both H3PO4 and H2O molecules that are formed and fixed within the nanostructure.24 In the temperature range of 30–90 °C, the activation energies for the proton transport in CMP-C2-P and CMP-C2-P-45% are 0.65 and 0.11 eV, respectively (Fig. 4c), implying a relatively low energy barrier and easier transfer of proton carriers in CMP-Cx-P-H.
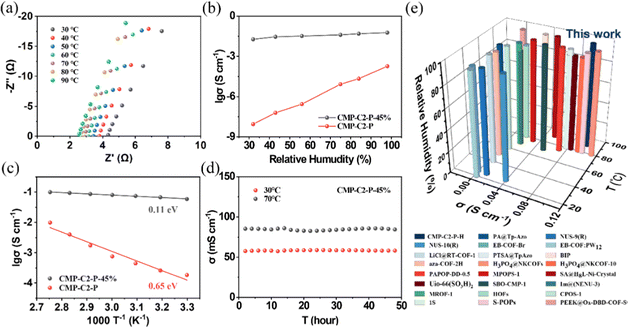 |
| Fig. 4 (a) Nyquist plots of CMP-C2-P-45% under 98% RH at 30–90 °C. (b) Proton conductivities for CMP-C2-P and CMP-C2-P-45% under different relative humidities at 30 °C. (c) Arrhenius plots for CMP-C2-P and CMP-C2-P-45% under 98% RH. (d) Long-period test for CMP-C2-P-45% under 98% RH at 70 °C. (e) Comparison of humid proton conductivities in reported materials. | |
We measured the samples of H3PO4@CMP-F6-45% under 98% RH as well (Fig. S33 and S34, ESI†). The proton conductivity of 5.51 × 10−3 S cm−1 obtained for H3PO4@CMP-F6-45% at 90 °C is 1 order of magnitude lower than CMP-C2-P-45% (σ = 9.93 × 10−2 S cm−1), which was attributed to the significant difference in hydrophilicity and hydrophobicity. According to the water contact angle tests (Fig. 1d and Fig. S22, ESI†), on account of the pre-design multiple hydrophilic groups, phosphonic acid groups, CMP-Cx-P and CMP-P show certain hydrophilicities (71°, 72°, 78°, and 66° for CMP-C2/C4/C6-P and CMP-P, respectively). After further doping with H3PO4, CMP-Cx-P-H and CMP-P exhibit superhydrophilicity (19°, 30°, 34°, and 17° for CMP-C2/C4/C6-45% and CMP-P-45%, respectively). Apparently, the more pronounced property in hydrophilicity of CMP-C2-P-45% than that of the previously reported H3PO4@CMP-F6-45% and notable water vapor uptake mean a better adsorption capacity for H2O molecules, which is essential to humid proton conductivity.
Furthermore, a long-period test for CMP-C2-P-45% was also performed under 98% RH at different temperatures (Fig. 4d and Fig. S35, ESI†), in which, at 30 and 70 °C, CMP-C2-P-45% displays high and persistent proton conductivities of about 5.84 × 10−2 and 8.46 × 10−2 S cm−1 for more than 48 hours, respectively. However, at 90 °C, it shows an average proton conductivity of 7.09 × 10−2 S cm−1 within 48 h, which was 22.1% lower than the initial value. As for the proton conductivity degradation caused by a further increase in temperature above 70 °C, it is attributed to the loss of H3PO4 molecules when the operating temperature is too high. Notably, CMP-C2-P-H exhibits a good proton transfer capability among other porous materials reported in the literature (Fig. 4e and Table S1, ESI†).23,24,27,28,30,41,43–45,49,64–75 According to this result, CMP-Cx-P-H performs well with an excellent electrochemical stability under humid conditions. In the meantime, it demonstrates that CMP-Cx-P-H not only shows high efficiency and stability in anhydrous proton conduction, but is also promising for application in proton conduction under relative humidity.
Involving the doped guest H3PO4 molecules, the abundant P
O and O–H groups on the nanopore walls of CMP-Cx-P show potential sites of hydrogen bonds (Scheme 1b). The interactions between CMP-Cx-P and the doped guest H3PO4 molecules are illustrated by X-ray photoelectron spectroscopy (XPS). The high-resolution O1s spectra of CMP-C2-P are shown in Fig. 5a. Two peaks appear at 531.7 and 533.2 eV, which are assigned to the O atoms of P
O and P–O bonds, respectively.76 The high-resolution O1s spectra (Fig. 5a) of CMP-C2-P-45% show two peaks at 532.2 and 533.7 eV, indicating that the effective binding energy values of the O atoms of the P
O/P–O bonds significantly increase due to the interactions between the anchored phosphonic acid groups in the CMP-C2-P structure and guest H3PO4 molecules. The P 2p3/2/P 2p1/2 doublet is fitted with an energy difference of 0.9 eV in CMP-C2-P and CMP-C2-P-45% (Fig. 5b).77 Besides, BE = 133.6 eV (P 2p3/2) and BE = 134.5 eV (P 2p1/2) change to 134.8 and 135.7 eV, respectively, reflecting the existence of P
O⋯H–O hydrogen-bonds. In addition, a shift of 51 cm−1 for the stretching vibrational band of the P
O bond from 919 cm−1 of CMP-C2-P to 970 cm−1 of CMP-C2-P-45% in the FT-IR spectra (Fig. 5c) indicates the formation of P
O⋯H–O hydrogen-bonds as well.55,78,79 As shown in Fig. 5d, the disordered structure of CMP-Cx-P-H provides stable and in-all-directions channels for proton transport under humid conditions. The abundance of phosphonic acid groups can act as a support and a proton donor, and provide sites for anchoring the H3PO4 network through hydrogen-bonding of P
O⋯H–O. Based on the experimental facts of CMP-C2-P-45%, we believe that some of the H3PO4 molecules are anchored in the pore walls, while most H3PO4 molecules and H2O molecules are linked together and form proton-conducting channels by hydrogen-bonding. Furthermore, alkoxy side chains and H3PO4 molecules are highly dynamic and mobile and integrating with the hydrophilic side chains and the hydrophobic skeleton promotes the microphase separation inside the CMPs, thus side chains prompt phosphonic acid groups to move close to H2O molecules and form hydrated protons, and by the rotation of H3PO4 molecules, protons can hop across the hydrogen-bonding networks, promoting the mobility of protons.26,80,81
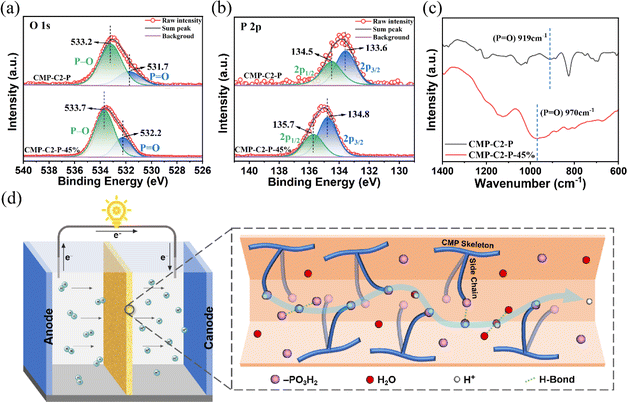 |
| Fig. 5 XPS spectra of (a) O1s bands of CMP-C2-P and CMP-C2-P-45%, and (b) P2p bands of CMP-C2-P and CMP-C2-P45%. (c) FT-IR spectra of CMP-C2-P and CMP-C2-P-45%. (d) Illustration for the proposed humid proton conduction mechanism of CMP-Cx-P-H. | |
Conclusions
In summary, the universal proton-conducting materials—the phosphonic acid group-functionalized CMPs, CMP-Cx-P-H, are demonstrated for the first time by a bottom-up strategy with side-chain engineering. The multiple phosphonic acid side chains that are highly dynamic and anchored within the channels are helpful to form a hydrophilic environment and dense hydrogen bonds with the guest H3PO4 molecules. With the strong hydrogen-bonding networks, CMP-C2-P-H showed excellent proton conductivities under various conditions, including 2.15 × 10−2 and 1.15 × 10−5 S cm−1 at 130 and −40 °C, respectively, under anhydrous conditions, as well as 9.93 × 10−2 S cm−1 at 90 °C under 98% relative humidity. CMP-Cx-P-H are the first porous organic polymer proton-conducting materials proposed to be suitable for both humid and anhydrous dual scenarios, as well as being universal over an ultra-wide temperature range of −40 °C to 130 °C. This work paves the way for realizing multi-environmental applications of polymer electrolyte membrane fuel cells and their use in extreme conditions.
Author contributions
Kaijie Yang: synthesis, methodology, validation, investigation, data curation, and writing. Yuxiang Wang: investigation, data curation, and formal analysis. Zhiyi Ling: investigation, data curation, and formal analysis. Xiaogang Pan: investigation. Gen Zhang: validation, and writing – review & editing. Jian Su: validation, and writing – review & editing.
Conflicts of interest
There are no conflicts to declare.
Acknowledgements
The authors are grateful to Prof. Jing-Lin Zuo at Nanjing University for his help in this work. This work was financially supported by startup funding from the Nanjing University of Science and Technology (AE89991/194, AE89991/259, AD41913, AD41960, and AE89991/376), the National Natural Science Foundation of China (22171136), “the Fundamental Research Funds for the Central Universities” (30921011102, 30922010301, 30922010902), and the Natural Science Foundation of Jiangsu Province (BK20220079, BK20220928). G. Z. acknowledges the support from the Thousand Young Talent Plans.
References
- K. A. Mauritz and R. B. Moore, Chem. Rev., 2004, 104, 4535–4585 CrossRef CAS.
- K. Schmidt-Rohr and Q. Chen, Nat. Mater., 2008, 7, 75–83 CrossRef CAS PubMed.
- R. Devanathan, Energy Environ. Sci., 2008, 1, 101–119 RSC.
- G. L. Jackson, D. V. Perroni and M. K. Mahanthappa, J. Phys. Chem. B, 2017, 121, 9429–9436 CrossRef CAS PubMed.
- S. J. Paddison, Annu. Rev. Mater. Res., 2003, 33, 289–319 CrossRef CAS.
- Y. Wang, K. S. Chen, J. Mishler, S. C. Cho and X. C. Adroher, Appl. Energy, 2011, 88, 981–1007 CrossRef CAS.
- R. K. Ahluwalia, X. Wang, W. B. Johnson, F. Berg and D. Kadylak, J. Power Sources, 2015, 291, 225–238 CrossRef CAS.
- Y. Yin, Z. Li, X. Yang, L. Cao, C. Wang, B. Zhang, H. Wu and Z. Jiang, J. Power Sources, 2016, 332, 265–273 CrossRef CAS.
- E. Quartarone and P. Mustarelli, Energy Environ. Sci., 2012, 5, 6436–6444 RSC.
- S. Y. Kim, S. Kim and M. J. Park, Nat. Commun., 2010, 1, 88 CrossRef PubMed.
- Y. X. Ye, L. Q. Zhang, Q. F. Peng, G. E. Wang, Y. C. Shen, Z. Y. Li, L. H. Wang, X. L. Ma, Q. H. Chen, Z. J. Zhang and S. C. Xiang, J. Am. Chem. Soc., 2015, 137, 913–918 CrossRef CAS PubMed.
- D. W. Shin, M. D. Guiver and Y. M. Lee, Chem. Rev., 2017, 117, 4759–4805 CrossRef CAS PubMed.
- Y. Chen, M. Thorn, S. Christensen, C. Versek, A. Poe, R. C. Hayward, M. T. Tuominen and S. Thayumanavan, Nat. Chem., 2010, 2, 503–508 CrossRef CAS PubMed.
- L. Vilciauskas, M. E. Tuckerman, G. Bester, S. J. Paddison and K.-D. Kreuer, Nat. Chem., 2012, 4, 461–466 CrossRef CAS.
- S.-Y. Moon, J.-S. Bae, E. Jeon and J.-W. Park, Angew. Chem., Int. Ed., 2010, 49, 9504–9508 CrossRef CAS PubMed.
- W. Luo, Y. Zhu, J. Zhang, J. He, Z. Chi, P. W. Miller, L. Chen and C.-Y. Su, Chem. Commun., 2014, 50, 11942–11945 RSC.
- Y. Wang, D. F. R. Diaz, K. S. Chen, Z. Wang and X. C. Adroher, Mater. Today, 2020, 32, 178–203 CrossRef CAS.
- D. B. Shinde, H. B. Aiyappa, M. Bhadra, B. P. Biswal, P. Wadge, S. Kandambeth, B. Garai, T. Kundu, S. Kurungot and R. Banerjee, J. Mater. Chem. A, 2016, 4, 2682–2690 RSC.
- R. S. Raja Rafidah, W. Rashmi, M. Khalid, W. Y. Wong and J. Priyanka, Polymers, 2020, 12, 1061 CrossRef.
- S. C. Sahoo, T. Kundu and R. Banerjee, J. Am. Chem. Soc., 2011, 133, 17950–17958 CrossRef CAS.
- F. Yang, G. Xu, Y. Dou, B. Wang, H. Zhang, H. Wu, W. Zhou, J.-R. Li and B. Chen, Nat. Energy, 2017, 2, 877–883 CrossRef CAS.
- M. Yoon, K. Suh, S. Natarajan and K. Kim, Angew. Chem., Int. Ed., 2013, 52, 2688–2700 CrossRef CAS PubMed.
- D. Chakraborty, A. Ghorai, A. Chowdhury, S. Banerjee and A. Bhaumik, Chem. – Asian J., 2021, 16, 1562–1569 CrossRef CAS PubMed.
- Z. Meng, A. Aykanat and K. A. Mirica, Chem. Mater., 2019, 31, 819–825 CrossRef CAS.
- S. Tao, L. Zhai, A. D. Dinga Wonanke, M. A. Addicoat, Q. Jiang and D. Jiang, Nat. Commun., 2020, 11, 1981 CrossRef CAS PubMed.
- X. Wu, Y.-l Hong, B. Xu, Y. Nishiyama, W. Jiang, J. Zhu, G. Zhang, S. Kitagawa and S. Horike, J. Am. Chem. Soc., 2020, 142, 14357–14364 CrossRef CAS PubMed.
- D. W. Kang, J. H. Song, K. J. Lee, H. G. Lee, J. E. Kim, H. Y. Lee, J. Y. Kim and C. S. Hong, J. Mater. Chem. A, 2017, 5, 17492–17498 RSC.
- D. W. Kang, K. S. Lim, K. J. Lee, J. H. Lee, W. R. Lee, J. H. Song, K. H. Yeom, J. Y. Kim and C. S. Hong, Angew. Chem., Int. Ed., 2016, 55, 16123–16126 CrossRef CAS PubMed.
- A. Jankowska, A. Zalewska, A. Skalska, A. Ostrowski and S. Kowalak, Chem. Commun., 2017, 53, 2475–2478 RSC.
- P. Bhanja, A. Paui, S. Chatterjee, Y. V. Kaneti, J. Na, Y. Sugahara, A. Bhaumik and Y. Yamauchi, ACS Sustainable Chem. Eng., 2020, 8, 2423–2432 CrossRef CAS.
- A. Modak, M. Nandi, J. Mondal and A. Bhaumik, Chem. Commun., 2012, 48, 248–250 RSC.
- P. Lindemann, M. Tsotsalas, S. Shishatskiy, V. Abetz, P. Krolla-Sidenstein, C. Azucena, L. Monnereau, A. Beyer, A. Goelzhaeuser, V. Mugnaini, H. Gliemann, S. Braese and C. Woell, Chem. Mater., 2014, 26, 7189–7193 CrossRef CAS.
- K. V. Rao, S. Mohapatra, T. K. Maji and S. J. George, Chem. – Eur. J., 2012, 18, 4505–4509 CrossRef CAS PubMed.
- X.-S. Wang, J. Liu, J. M. Bonefont, D.-Q. Yuan, P. K. Thallapally and S. Ma, Chem. Commun., 2013, 49, 1533–1535 RSC.
- A. Modak, J. Mondal and A. Bhaumik, Appl. Catal. A-Gen., 2013, 459, 41–51 CrossRef CAS.
- H. Zhao, G. Yu, M. Yuan, J. Yang, D. Xu and Z. Dong, Nanoscale, 2018, 10, 21466–21474 RSC.
- N. Kang, J. H. Park, K. C. Ko, J. Chun, E. Kim, H.-W. Shin, S. M. Lee, H. J. Kim, T. K. Ahn, J. Y. Lee and S. U. Son, Angew. Chem., Int. Ed., 2013, 52, 6228–6232 CrossRef CAS.
- Z. J. Wang, S. Ghasimi, K. Landfester and K. A. I. Zhang, Chem. Commun., 2014, 50, 8177–8180 RSC.
- Y. Xu, A. Nagai and D. Jiang, Chem. Commun., 2013, 49, 1591–1593 RSC.
- P. Zhang, K. Wu, J. Guo and C. Wang, ACS Macro Lett., 2014, 3, 1139–1144 CrossRef CAS PubMed.
- S.-J. Yang, X. Ding and B.-H. Han, Langmuir, 2018, 34, 7640–7646 CrossRef CAS.
- X. Jiang, K. Zhang, Y. Huang, B. Xu, X. Xu, J. Zhang, Z. Liu, Y. Wang, Y. Pan, S. Bian, Q. Chen, X. Wu and G. Zhang, ACS Appl. Mater. Interfaces, 2021, 13, 15536–15541 CrossRef CAS.
- Z. Li, Y. Yao, D. Wang, M. M. Hasan, A. Suwansoontorn, H. Li, G. Du, Z. Liu and Y. Nagao, Mat. Chem. Front., 2020, 4, 2339–2345 RSC.
- C. Klumpen, S. Goedrich, G. Papastavrou and J. Senker, Chem. Commun., 2017, 53, 7592–7595 RSC.
- T. Zhu, B. Shi, H. Wu, X. You, X. Wang, C. Fan, Q. Peng and Z. Jiang, Ind. Eng. Chem. Res., 2021, 60, 6337–6343 CrossRef CAS.
- P. Li, J. Chen and S. Tang, Chem. Eng. J., 2021, 415, 129021 CrossRef CAS.
- C. Fan, H. Wu, Y. Li, B. Shi, X. He, M. Qiu, X. Mao and Z. Jiang, Solid State Ionics, 2020, 349, 115316 CrossRef CAS.
- B. Zhou, J. Le, Z. Cheng, X. Zhao, M. Shen, M. Xie, B. Hu, X. Yang, L. Chen and H. Chen, ACS Appl. Mater. Interfaces, 2020, 12, 8198–8205 CrossRef CAS.
- S. Chandra, T. Kundu, S. Kandambeth, R. BabaRao, Y. Marathe, S. M. Kunjir and R. Banerjee, J. Am. Chem. Soc., 2014, 136, 6570–6573 CrossRef CAS.
- D. Gui, W. Duan, J. Shu, F. Zhai and S. Wang, CCS Chem., 2019, 1, 197–206 CAS.
- J.-X. Jiang, F. Su, A. Trewin, C. D. Wood, N. L. Campbell, H. Niu, C. Dickinson, A. Y. Ganin, M. J. Rosseinsky, Y. Z. Khimyak and A. I. Cooper, Angew. Chem., Int. Ed., 2007, 46, 8574–8578 CrossRef CAS PubMed.
- K. S. W. Sing, Pure Appl. Chem., 1985, 57, 603–619 CrossRef CAS.
- H. Xu, S. Tao and D. Jiang, Nat. Mater., 2016, 15, 722 CrossRef CAS PubMed.
- S. Chandra, T. Kundu, K. Dey, M. Addicoat, T. Heine and R. Banerjee, Chem. Mater., 2016, 28, 1489–1494 CrossRef CAS.
- J. Li, J. Wang, Z. Wu, S. Tao and D. Jiang, Angew. Chem., Int. Ed., 2021, 60, 12918–12923 CrossRef CAS PubMed.
- Y. Guo, X. Zou, W. Li, Y. Hu, Z. Jin, Z. Sun, S. Gong, S. Guo and F. Yan, J. Mater. Chem. A, 2022, 10, 6499–6507 RSC.
- W. Huang, B. Li, Y. Wu, Y. Zhang, W. Zhang, S. Chen, Y. Fu, T. Yan and H. Ma, ACS Appl. Mater. Interfaces, 2021, 13, 13604–13612 CrossRef CAS.
- P. Ramaswamy, N. E. Wong and G. K. H. Shimizu, Chem. Soc. Rev., 2014, 43, 5913–5932 RSC.
- S. Pili, S. P. Argent, C. G. Morris, P. Rought, V. Garcia-Sakai, I. P. Silverwood, T. L. Easun, M. Li, M. R. Warren, C. A. Murray, C. C. Tang, S. Yang and M. Schroeder, J. Am. Chem. Soc., 2016, 138, 6352–6355 CrossRef CAS PubMed.
- H.-B. Luo, L.-T. Ren, W.-H. Ning, S.-X. Liu, J.-L. Liu and X.-M. Ren, Adv. Mater., 2016, 28, 1663–1667 CrossRef CAS PubMed.
- Y. Ye, X. Wu, Z. Yao, L. Wu, Z. Cai, L. Wang, X. Ma, Q.-H. Chen, Z. Zhang and S. Xiang, J. Mater. Chem. A, 2016, 4, 4062–4070 RSC.
- J. A. Hurd, R. Vaidhyanathan, V. Thangadurai, C. I. Ratcliffe, I. L. Moudrakovski and G. K. H. Shimizu, Nat. Chem., 2009, 1, 705–710 CrossRef CAS.
- B. Hou, S. Yang, B. Li, G. Li, H. Zheng, C. Qin, G. Shan, Z. Su and X. Wang, Inorg. Chem. Front., 2022, 9, 4376–4384 RSC.
- Z. Wang, Y. Yang, Z. Zhao, P. Zhang, Y. Zhang, J. Liu, S. Ma, P. Cheng, Y. Chen and Z. Zhang, Nat. Commun., 2021, 12, 1982 CrossRef CAS.
- Y. W. Peng, G. D. Xu, Z. G. Hu, Y. D. Cheng, C. L. Chi, D. Q. Yuan, H. S. Cheng and D. Zhao, ACS Appl. Mater. Interfaces, 2016, 8, 18505–18512 CrossRef CAS PubMed.
- H. P. Ma, B. L. Liu, B. Li, L. M. Zhang, Y. G. Li, H. Q. Tan, H. Y. Zang and G. S. Zhu, J. Am. Chem. Soc., 2016, 138, 5897–5903 CrossRef CAS PubMed.
- C. Montoro, D. Rodriguez-San-Miguel, E. Polo, R. Escudero-Cid, M. L. Ruiz-Gonzalez, J. A. R. Navarro, P. Ocon and F. Zamora, J. Am. Chem. Soc., 2017, 139, 10079–10086 CrossRef CAS PubMed.
- H. S. Sasmal, H. B. Aiyappa, S. N. Bhange, S. Karak, A. Halder, S. Kurungot and R. Banerjee, Angew. Chem., Int. Ed., 2018, 57, 10894–10898 CrossRef CAS PubMed.
- K. C. Ranjeesh, R. Ilathvalappil, S. D. Veer, J. Peter, V. C. Wakchaure, Goudappagouda, K. V. Raj, S. Kurungot and S. S. Babu, J. Am. Chem. Soc., 2019, 141, 14950–14954 CrossRef CAS PubMed.
- Y. Yang, X. He, P. Zhang, Y. H. Andaloussi, H. Zhang, Z. Jiang, Y. Chen, S. Ma, P. Cheng and Z. Zhang, Angew. Chem., Int. Ed., 2020, 59, 3678–3684 CrossRef CAS PubMed.
- Z. Yang, P. Chen, W. Hao, Z. Xie, Y. Feng, G. Xing and L. Chen, Chem. – Eur. J., 2021, 27, 3817–3822 CrossRef CAS PubMed.
- W. J. Phang, H. Jo, W. R. Lee, J. H. Song, K. Yoo, B. Kim and C. S. Hong, Angew. Chem., Int. Ed., 2015, 54, 5142–5146 CrossRef CAS PubMed.
- Y. Ye, W. Guo, L. Wan, Z. Li, Z. Song, J. Chen, Z. Zhang, S. Xiang and B. Chen, J. Am. Chem. Soc., 2017, 139, 15604–15607 CrossRef CAS PubMed.
- Y.-H. Han, Y. Ye, C. Tian, Z. Zhang, S.-W. Du and S. Xiang, J. Mater. Chem. A, 2016, 4, 18742–18746 RSC.
- D. W. Kang, M. Kang and C. S. Hong, J. Mater. Chem. A, 2020, 8, 7474–7494 RSC.
- P. Y. Shih, S. W. Yung and T. S. Chin, J. Non-Cryst. Solids, 1999, 244, 211–222 CrossRef CAS.
- A. Majjane, A. Chahine, M. Et-tabirou, B. Echchahed, D. Trong-On and P. Mc Breen, Mater. Chem. Phys., 2014, 143, 779–787 CrossRef CAS.
- M. Textor, L. Ruiz, R. Hofer, A. Rossi, K. Feldman, G. Hahner and N. D. Spencer, Langmuir, 2000, 16, 3257–3271 CrossRef CAS.
- X. Chen, M. Addicoat, E. Jin, L. Zhai, H. Xu, N. Huang, Z. Guo, L. Liu, S. Irle and D. Jiang, J. Am. Chem. Soc., 2015, 137, 3241–3247 CrossRef CAS PubMed.
- Z. Lu, C. Yang, L. He, J. Hong, C. Huang, T. Wu, X. Wang, Z. Wu, X. Liu, Z. Miao, B. Zeng, Y. Xu, C. Yuan and L. Dai, J. Am. Chem. Soc., 2022, 144, 9624–9633 CrossRef CAS.
- S. Wang, X. Tang, K. Yang, B. Chen, K. Zhang, H. Xu, W. Wang, G. Zhang and C. Gu, Macromol. Rapid Commun., 2022, e2200678 CrossRef PubMed.
|
This journal is © The Royal Society of Chemistry 2023 |
Click here to see how this site uses Cookies. View our privacy policy here.