DOI:
10.1039/D2LC00933A
(Perspective)
Lab Chip, 2023,
23, 1389-1409
Design of functional nanoparticles by microfluidic platforms as advanced drug delivery systems for cancer therapy
Received
5th October 2022
, Accepted 28th November 2022
First published on 17th January 2023
Abstract
Nanoparticle systems are functional carriers that can be used in the cancer therapy field for the delivery of a variety of hydrophobic and/or hydrophilic drugs. Recently, the advent of microfluidic platforms represents an advanced approach to the development of new nanoparticle-based drug delivery systems. Particularly, microfluidics can simplify the design of new nanoparticle-based systems with tunable physicochemical properties such as size, size distribution and morphology, ensuring high batch-to-batch reproducibility and consequently, an enhanced therapeutic effect in vitro and in vivo. In this perspective, we present accurate state-of-the-art microfluidic platforms focusing on the fabrication of polymer-based, lipid-based, lipid/polymer-based, inorganic-based and metal-based nanoparticles for biomedical applications.
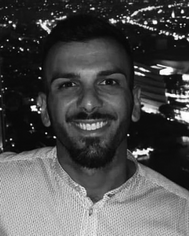 Antonio Fabozzi | Dr. Antonio Fabozzi is a researcher at Altergon ITALIA Srl. He received his master's degree and then PhD degree in Chemical Sciences from the University of Naples “Federico II”. He currently works in collaboration between the Institute of Polymers, Composites and Biomaterials (IPCB) of National Research Council (CNR) of Naples (Italy) and Altergon ITALIA Srl. His research interests are focused on the preparation of polymer-based nanomaterials by means of microfluidics and their physicochemical characterization for the release of active principles in the drug delivery field. |
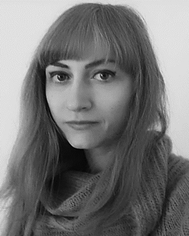 Francesca Della Sala | Dr. Francesca Della Sala obtained her master's degree in Medical Biotechnology from the University of Naples “Federico II”, and then obtained her PhD degree in Biomolecular Sciences from the University of Campania “Luigi Vanvitelli”. She currently works as a researcher at the Institute of Polymers, Composites and Biomaterials (IPCB) of National Research Council (CNR) of Italy. Her research interests are focused on biomaterials for tissue engineering and drug delivery application. In particular, she is interested in the study of nano–micro particles for targeted delivery and innovative polymeric substrates for regenerative medicine and cell delivery. |
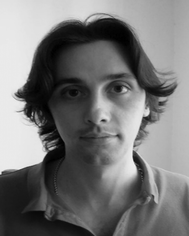 Mario di Gennaro | Mario di Gennaro received his master's degree in Science and Technologies of Industrial Chemistry from the University of Naples “Federico II”. Currently, he is a PhD student in Biomolecular Sciences at the University of Campania “Luigi Vanvitelli” under the supervision of Dr. Ing. Assunta Borzacchiello. His current research interests are focused on the preparation and physical, chemical and rheological characterization of polysaccharide-based nanostructured materials for the release of active principles in the biomedical applications field. |
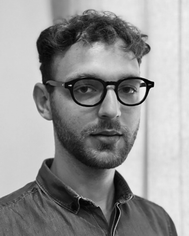 Marco Barretta | Marco Barretta received his master's degree in Materials Engineering cum laude from the University of Naples “Federico II”. Currently, he is a post graduate student at IPCB-National Research Council of Italy under the supervision of Dr. Ing. Assunta Borzacchiello. His current research is based on the preparation and physicochemical characterization of polysaccharide-based nanostructured materials for the release of active principles in the biomedical applications field. |
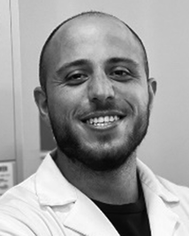 Gennaro Longobardo | Gennaro Longobardo obtained his master's degree in Materials Engineering cum laude from the University of Naples “Federico II”. Currently, he is a PhD student in “Industrial Products and Process Engineering” at the University of Naples “Federico II” under the supervision of Dr. Ing. Assunta Borzacchiello. His current research interests are focused on the preparation and characterization of biomaterials for tissue engineering and controlled drug release. |
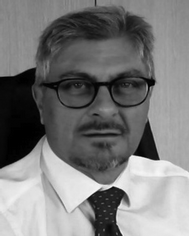 Nicola Solimando | Dr. Nicola Solimando received his master's degree in Biology cum laude from University la Sapienza (Rome) in 1995 and he did research activity as a research fellow at the same university in the field of genetic engineering and chemistry of fermentation processes. Since 2010 he has been a Production and R&D manager at Altergon ITALIA S.r.l and his research interests are related to fermentation plants of injectable grade polysaccharides and development of microneedles and microfluidics technology on the pilot scale. During more than 20 years of working in pharmaceutical companies, he has acquired knowledge in project management and technology transfer. |
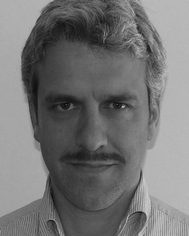 Maurizio Pagliuca | Dr. Maurizio Pagliuca earned his master's degree in Biological Sciences cum laude from the University of Naples “Federico II”. From 2003 to 2009 he worked as a Site & Technical Director at Altergon Italia, and since 2009 he has held the office of Chief Operating Officer – Technical Director at Altergon Italia. With over 30 years in the pharmaceutical industry, Dr. Pagliuca's experience includes the biotech production of ultrapure hyaluronate and ultrapure GAGs, and the research and development and the industrial scale up of customized drug delivery systems. |
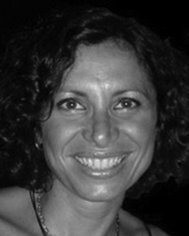 Assunta Borzacchiello | Assunta Borzacchiello earned her master's degree in Chemical Engineering cum laude (1994) and PhD degree in Material Technologies (1998) from the University of Naples “Federico II”. She was a Research Scientist at QMWC University of London (1996) and at the University of Connecticut, USA (1997). She was a Professor of Biomaterials at the University of Naples “Federico II” from 2002–2011. She was a Visiting Professor at McGill University, Canada (2018, 2019). She is currently a Senior Scientist at IPCB-National Research Council of Italy. The Borzacchiello group is currently focusing on biomaterials for tissue engineering and drug delivery applications, microfluidic techniques for biomedical applications and rheology and microrheology of complex fluids. |
1. Introduction
In the last few decades, functional structured nanoparticles (NPs) have attracted significant attention in the biomedical field thanks to their use as vehicles for the controlled delivery of different types of drugs.1,2 The therapeutic efficacy of NPs is strictly connected to their physicochemical properties such as size and morphology; however, to obtain NPs of the desired size and shape with high batch-to-batch reproducibility remains technically challenging in the conventional synthetic batch methods.3,4 These features are the key points for the treatment of many forms of cancer. In particular, NPs can increase the drug concentration in cancer cells and help to overcome some limitations of conventional chemotherapy such as the low specificity to cancer cells and the toxic effects on healthy cells.5,6 NPs can take advantage of the enhanced permeability and retention effect (EPR) that facilitates the NP accumulation into cells and tissues of solid tumors.7,8 The EPR effect is generally displayed by tumor vessels, which show some characteristics such as an anomalous rate of growth, a disordered vascular architecture and high permeability.8,9 Unfortunately, the EPR efficacy/efficiency is limited by some drawbacks such as a possible shortage of NP cell uptake, an undesired release of the drug before the NPs are internalized and a low percentage of the administered dose.10 A possible strategy to overcome these limitations is aimed at providing the NPs with active targeting moieties by means of appropriate modifications of NP surfaces such as nucleic acids,11,12 antibodies,13 proteins,14 and folate.15 Indeed, functionalized NPs can be recognized and subsequently bind tumor cells and can even be internalized via receptor-mediated endocytosis.15,16 However not all modifications of the NP surface represent an effective strategy to overcome the limitations of EPR, for instance recently, folic acid-functionalized liposomes do not enhance the distribution of liposomes in FR-α-overexpressed tumors.17 For all these reasons, the NP fabrication methods represent an important tool for the reproducibility of biological assays. In particular, there are a variety of NP synthetic conventional methods such as nanoprecipitation,18 solvent evaporation,19,20 microemulsions,21 sol–gel,22,23 emulsion polymerization,24 layer-by-layer self-assembly,25 thin-film hydration,26 bulk mixing by extrusion,27 pipette mixing,28 electrodeposition and thermal decomposition.29,30 In these methods, the formation of the NPs can be divided into three stages, a) nucleation, b) growth and c) aggregation, which occur concurrently leading to some differences, in terms of physicochemical properties, from batch-to-batch of synthesized NPs.31 The inability to control the physicochemical properties of each synthetic batch of NPs leads to low reproducibility of both in vitro and consequently in vivo biological tests.32 The new continuous-flow microfluidic systems are largely investigated for their properties such as rapid mass transfer, precise control, large reaction interfaces, and mixing efficacy.33 In particular, the nucleation, growth, and aggregation steps of the NPs can be separated as a function of distance from the position where the solution occurs in order to achieve absolute control of the physicochemical properties, including particle size and morphology.34,35 Thus, compared to conventional methods, the microfluidic technique allows for overcoming all limitations for the production of NPs, increasing the reproducibility of each synthetic batch and favoring the industrial scale-up, and represents a new strategy capable of overcoming challenges in the clinical field of NP drug carriers36 (Table 1). In this perspective, we discuss and highlight the developments and the applications of NPs fabricated by microfluidics as advanced delivery systems focusing on: a) polymer-based NPs, b) lipid-based NPs, c) lipid/polymer-based NPs, d) inorganic-based nanoparticles, and e) metal-based nanoparticles.
Table 1 Examples of NPs fabricated by microfluidics for biomedical applications
Nanomaterials |
Microfluidic mechanisms |
Active principles |
Drug encapsulation efficiency (%) |
Ref. |
Polymer-based NPs |
Natural polymers |
L/CSHA/CS |
Self-assemblyNanoprecipitation |
Docetaxel/curcuminEverolimus |
95/9988 |
85 and86 |
Synthetic polymers |
PLGAPTMC-PCL/PEG-b-PDLLA |
EmulsificationNanoprecipitation |
5-FluorouracilDoxorubicin |
9551 |
87 and88 |
Lipid-based NPs |
Lipid NPs |
C12–200/DOPE cholesterol lipid–PEG |
Self-assembly |
siRNA and/or mRNA |
83/81 |
98
|
Tf-lipid NPs |
Self-assembly |
siRNA |
78 |
99
|
Solid lipid NPs |
Cetyl palmitate |
Nanoprecipitation |
PaclitaxelSorafenib |
5479 |
119
|
Lipid/polymer-based NPsCore–shell NPs |
PLGA/DSPE-PEG2000 |
Nanoprecipitation |
Sorafenib |
88–95 |
125
|
PCL-PEI/cholesterol,DSPE-PEG2000, DOPE |
Three step self-assembly |
siRNA |
79–98 |
26
|
PLGA-lipid/PEG |
Nanoprecipitation |
— |
— |
129
|
Inorganic-based NPs |
Mesoporous silica NPs |
Dox-loaded/MSN/PTX/polystyrene sulfonate |
Nanoprecipitation |
Doxorubicin/paclitaxel |
70/94 |
141
|
Ellipsoidal mesoporous silica |
Dox-loaded EMSNs |
Nanoprecipitation |
Doxorubicin |
70 |
142
|
Quantum dots |
AIE QDs Dspe-PEG2k |
Nanoprecipitation |
— |
— |
146
|
MOF |
BioZIF-8 |
Self-assembly |
siRNA/BSA/DOX |
53/59/47 |
149
|
Metal-based NPs |
Cu-based NPs |
BSA/Cu(DDC)2MONs |
Nanoprecipitation |
Cu(DDC)2 |
66–95 |
162
|
Polymer–metal NPs |
Chitosan/silver NPs |
Emulsion |
Calotropis procera extract |
77 |
163
|
2. Flow systems for nanoparticle synthesis
Nowadays, NPs are synthesized by means of traditional methods from the corresponding precursors employing different approaches, described above, in which it is difficult to control their physicochemical properties.37 In the last decade, the microfluidic flow systems employed for NP fabrication have been largely studied due to their advantages such as high control of physicochemical properties and high reproducibility of synthetic batches.38,39 The microfluidic flow control systems depend on the Reynolds number (Re) and the Péclet number (Pe) that allow high levels of control over the production of NPs. Re describes the flow properties, as a ratio between inertial and viscous forces, and can be calculated by eqn (1). |  | (1) |
where ρ represents the density of the fluid, ν is the average velocity of the fluid, L represents the diameter of the pipe, and μ is the viscosity of the fluid.40,41 For a microfluidic setup, a high Re number can be obtained by increasing the flow rate and/or the density of the liquids or by decreasing the viscosity of the fluids. However, a Re number >2300 produces a turbulent flow with random streamlines (Fig. 1a).42 In contrast, a Re number <2000 produces a laminar flow with distinct streamlines parallel to the fluid direction in order to achieve high control of monodisperse droplets (Fig. 1b).43
 |
| Fig. 1 Schematic illustration of different types of representative flow patterns in FEM microchannels simulated using COMSOL Multiphysics® (velocity magnitude (m/s)); the arrows represent the streamline velocity field: a) turbulent flow and b) laminar flow. | |
The Pe number describes the diffusion, based on the random thermal motion of molecules in their surrounding environment, and convection phenomena of the molecules, based on the movement of molecules from the bulk motion of a fluid.44 The Pe number range, 250–2500, shows an upper limit that is strictly connected to the Re constraint45 and can be calculated by eqn (2).
|  | (2) |
where
Ua represents the average velocity of the flow,
H is related to the length of the system, and
D represents the mass diffusion coefficient of the NPs. In a microfluidic droplet, the transport of molecules is slow and it occurs mostly by diffusion because of the laminar flow and small volume.
41 For this reason, the transport of molecules from the dispersed to the continuous phase is minimized, and consequently, a high drug encapsulation efficiency is obtained.
42,44 The microfluidic devices can be categorized into two classes, single-phase continuous (SPCF) flow and multi-phase flow microfluidic systems (MPF) even known as segmented flow.
2.1. Microfluidic systems based on single-phase continuous flow
The SPCF system is the most commonly employed in microfluidics because it is a homogeneous system that provides simplicity and versatility of a variety of features such as control of the flow, reaction time, temperature and reagents (Scheme 1a). In particular, the fabrication of NPs in SPCF microfluidic systems can be carried out using single or multiple miscible solvents and the reagents are mixed by means of diffusion in laminar flows.46,47 For the SPCF microfluidic systems, the main mixing mechanism is based on molecular interdiffusion obtained by means of laminar flows.48 Indeed, most single-phase microfluidic mixers are designed to operate with a Reynolds number <2000, in order to achieve the absence of turbulent flows.49
 |
| Scheme 1 Representative illustration of a) a single-phase flow system, b) a gas–liquid flow system and c) a liquid–liquid-flow system. | |
The mixing time (τmix) quadratically depends on the channel width and the flow rate ratio of the miscible flows and can be estimated by the following equation:
| 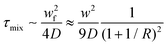 | (3) |
where
D is the diffusivity of the reagents,
wf is the width of the focused stream,
w is the channel width, and
R is the ratio of the flow rate between the reagent stream and the total flow rate of the solvent.
50 In order to improve the mixing performances, a transverse component of the flow can be incorporated and chaotic advancement can be activated in the microchannels through repeated cycles based on the stretching and bending of the flows. This can be achieved through the use of a staggered herringbone mixer in order to obtain exponentially thin flow, leading to the exponential growth of the interface between two fluids to obtain rapid mixing.
51 Furthermore, the micrometric dimensions of a microchannel permit the reduction of the mixing time to milliseconds during the nucleation stage in the NP synthesis.
52 Moreover, the SPCF system offers the advantage of modifications during NP synthesis by adding new reagents during the reaction.
53 From an industrial point of view, the SPCF system can be scalable through multiple parallel reactions in order to synthesize a large amount of NPs.
54
2.2. Microfluidic systems based on multiphase flow
MPF microfluidic-based systems are composed of two isolated phases of two or more immiscible fluid phases, in which the addition of a new phase activates a recirculation motion generating the stretching and bending of a solution that improves the mixing efficiency.55 Furthermore, the MPF synthesis strategy offers some advantages such as reducing the deviation of the residence time, because the reactions occur in well-defined droplets, and the reduced risk of channel clogging thanks to the minimization of the direct contact of the liquid with the microchannel.56 Moreover, MPF synthesis can be split into two sub-classes: gas–liquid flows (GlF) (Scheme 1b) and liquid–liquid flows (LlF) (Scheme 1c). The LlF consists of two phases, such as water-in-oil or oil-in-water dispersions, in which surfactants can be added in order to inhibit the coalescence of the dispersed droplets.57–59 The morphology of the droplets is strictly connected to two parameters: a) the flow rate and b) the chip design.60,61 Thanks to the appropriate mixing efficacy, mass transfer and quick heat in LlF systems, NPs with a variety of shapes can be synthesized in these systems.62,63 The reacting solutions are encapsulated within liquid droplets, preventing contamination and channel clogging, leading to the higher reproducibility of NP synthesis.64 The GlF systems are composed of a bubbly flow dispersed in a continuous liquid phase. The employment of a gas phase can significantly enhance the mixing efficacy thanks to the creation of recirculation.65,66 One of the most interesting characteristics of gas–liquid flow reactors is based on the separation of the gas from the liquid to achieve the desired NPs. Unfortunately, in the GlF systems, possible clogging can be obtained which can be avoided with the employment of liquid–liquid flow reactors.67
The microfluidic industrial scale-up technologies offer the opportunity for the fabrication on a large scale of NP-based systems widely used for cancer treatments.68 The high-throughput fabrication of NPs with control of physicochemical properties such as size and shape is one of the critical points for their effective biomedical applications.69 In particular, a microfluidic industrial scale-up device can be composed of thousands of micromechanical valves that permit the production of NPs in an automated device.70 Generally, two strategic approaches for the industrial scale-up of microfluidic drop generators have been developed and are based on a single chip in which there is an integration based on multiple parallel drop generation units71 or multiple chips each containing parallel drop generation units as described elsewhere.72 In particular, the two main microfluidic device configurations used for the industrial scale-up, employed to dispense fluids from a single manifold into multiple parallel drop generation units, are the tree-type and ladder-type.73,74 Although, the ladder-type configuration shows some advantages such as small random variation in the channel size and compact design, even if a clogging issue can inhibit the NP production,73 the tree-type is more energy efficient.74 For these reasons, a combination of two configurations based on multiple chips offers the possibility of synthesizing NPs even if a clogging issue can be detected. Although microfluidic technology is widely used for the synthesis of NPs, its industrial applicability is still an ongoing challenge due to the eventual clogging of both microchannels and microchips. For this reason, some enhancements need to be applied for the production of tons of nanomaterials. Moreover, a microfluidic method for both small and large-scale NP production is the use of a NanoAssemblr™. The microfluidic NanoAssemblr™ platform is employed for the rapid and controlled synthesis of a variety of NPs such as polymer-based75 NPs and polymer–lipid-based NPs in the nanomedicine field.76 In particular, the microfluidic mixer is designed to achieve optimization for precise control of the physicochemical properties requiring nanoparticle self-assembly in the nanomedicine field.77,78 NPs are synthesized on fast time scales minimizing the process variability. The NanoAssemblr™ synthesizes NPs in <1 min and kilograms of formulations per day; in particular, NPs developed by means of the NanoAssemblr™ are scaled without employing a microfluidic mixer parallelization and continuous flow pumping system. A single microfluidic mixer can work at N × 24 mL min−1 and can generate NPs higher than 24 mL min−1 of N-parallelized mixers.79
3. Application of nanoparticles fabricated by microfluidics as delivery systems
3.1. Polymer-based nanoparticles
Polymer-based NPs, composed of natural and synthetic polymers, have been widely fabricated by means of microfluidics and investigated in the drug delivery field thanks to their unique properties such as biodegradability and biocompatibility.80–82 The advantages of microfluidic fabrication of polymer-based NPs are based on the enhancement of the control of physicochemical properties such as size, size distribution, and morphologies.83,84 Some examples, reported in the literature in the last two years, based on polymeric NPs have been selected and described as follows.
Docetaxel (Dtx) and curcumin (Cur) loaded lignin/chitosan (L/CS) NPs were fabricated in a simple and scalable microfluidic system for flash nanoprecipitation to test their delivery potential and cytotoxicity. Briefly, L, used as the organic phase, and CS, solubilized in 0.1 M acetic acid, used as the aqueous phase, solutions were rapidly mixed in a torus-shaped mixing chamber and quickly co-assembled into L/CS NPs in a valve-assisted mixer equipped with a medium-pressure constant flow pump (Fig. 2).
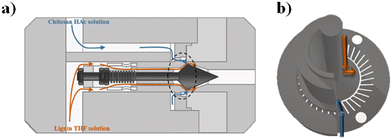 |
| Fig. 2 (a) Schematic representation of the valve-assisted mixer structure. (b) Fluid guidance via the mixing plate. Reprinted with permission from ref. 85. Copyright 2021. American Chemical Society. | |
The hydrophobic anticancer drugs such as Cur or Dtx were solubilized in the organic phase of L. The morphological analysis of L/CS NPs showed an average particle size of about 180 nm. The drug release amounts in acidic solutions simulating the tumor environment were 51% for Dtx-L/CS NPs and 50% for Cur-L/CS NPs, respectively, which were higher than the release amounts at pH 7.4, showing a killing effect, in both cases, on HeLa cells. Furthermore, L/CS NPs exhibited low cytotoxicity, demonstrating good cell compatibility.85 The microfluidic platform was employed for the one-pot fabrication of hyaluronic acid–chitosan (HA–CS) NPs, based on the polyelectrolytic interactions between CS and HA. Briefly, a staggered herringbone micromixer was used for the fabrication of HA–CS NPs in which a CS aqueous solution and a mixture composed of HA and sodium tripolyphosphate (TPP) aqueous solutions were injected into different microchannels (Fig. 3a).
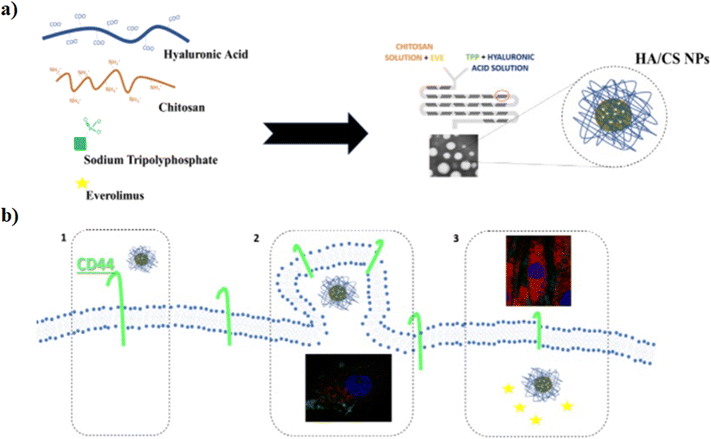 |
| Fig. 3 a) Mechanism of HA/CS NPs in the microfluidic chip; b) ideal mechanism of action: 1) interaction between HA/CS NPs and CD44, 2) NP internalization, and 3) everolimus release. Reprinted from ref. 86. | |
The microfluidic on-chip fabrication allowed the fabrication of HA/CS NPs with controlled size and appropriate for both loco-regional, 350 nm, and parenteral, 117 nm, administration. Everolimus (EV), a water-insoluble anticancer drug able to reduce angiogenesis and promote apoptosis, was encapsulated in the HA/CS NPs. In vitro cellular uptake tests, carried out on human mesenchymal stem cells, demonstrated a blockage of the CD44 receptor by the primary anti-CD44 antibody compared to CS-based NPs thanks to the high selectivity of HA/CS NPs with CD44 (Fig. 3b).86 Among all synthetic polymers, poly(D,L-lactide-co-glycolide) (PLGA) based NPs, fabricated by means of microfluidic methods, are the most employed in the drug delivery field. Indeed, during the NP synthetic process, a variety of drugs such as docetaxel, doxorubicin and curcumin can be effectively encapsulated inside the hydrophobic core composed of PLGA. An example, based on the fabrication of 5-fluorouracil (5-FU)-loaded PLGA NPs via the emulsification mechanism through a fork-shaped chip by means of a microfluidic technique, with diameters of ∼101 nm and a drug encapsulation efficiency of ∼95 for the potential use in colorectal cancer therapy, has been reported (Fig. 4a). Briefly, a mixture composed of 5-FU and poly(vinyl alcohol) was injected into the middle microchannel as a dispersed phase and PLGA solution as a continuous phase was inserted into the external microchannels.
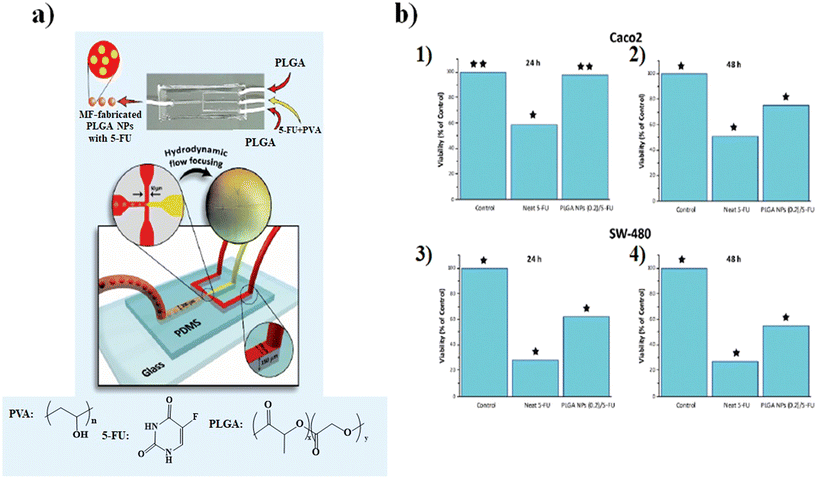 |
| Fig. 4 a) Representation of the microfluidic chip, b) MTT cytotoxicity assay results: Caco2 (1) 24 h and (2) 48 h and SW-480 (3) 24 h and (4) 48 h. Reprinted from ref. 87. | |
In particular, the biological assays on the Caco2 and SW-480 colon cancer cell lines demonstrate that the cell viability was decreased by increasing the exposure time of neat 5-FU and MF-fabricated PLGA NPs/5-FU (Fig. 4b). Furthermore, the flow cytometry and 4′,6-diamidino-2-phenylin-dole (DAPI) staining showed that the PLGA NPs/5-FU NPs were able to kill cancerous colon cells 1.5-fold higher than the control sample for Caco2 and SW-480 at a gradual rate and safe drug dosage.87 A microfluidic approach was employed for the preparation of (co)polymeric and (co)polymer–tannic acid (TA) NPs in a microfluidic flow-focusing glass-capillary device by means of microfluidic-assisted nanoprecipitation for breast cancer. The water phase was composed of Milli-Q water, while the organic phase was composed of poly(ε-caprolactone) (PCL) and poly(trimethylene carbonate) (PtMc) homopolymers and (co)polymers with different proportions of comonomers which were synthesized by means of enzymatic polymerization. Besides, TA was added to the (co)polymer solution to fabricate NPs. In the flow-focusing device, the (co)polymers, with doxorubicin (DOX) and TA, dissolved in N-methyl-2-pyrrolidone, were delivered to the outer capillary, and the aqueous phase flowed counter-currently by means of the space among the square and inner capillary and the resulting nanosuspension was collected from the inner capillary (Fig. 5a).
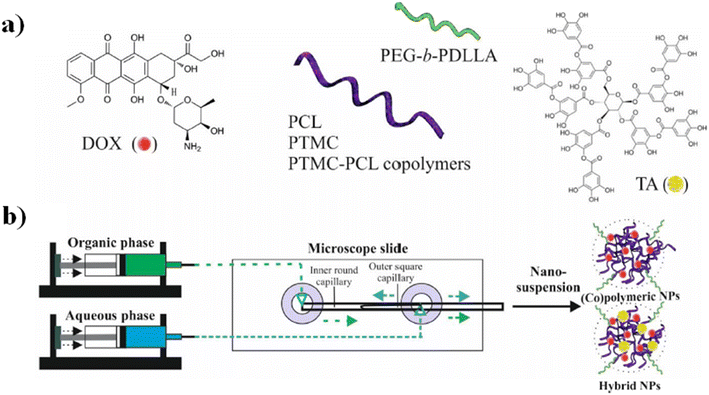 |
| Fig. 5 a) DOX, tannic acid, and the schematic illustration of (co)polymer chemical structures. (b) Schematic representation of NP fabrication in a microfluidic device and examples of the self-assembly into NPs in water. Reprinted with permission from ref. 88. Copyright 2021 Elsevier. | |
Furthermore, poly(ethylene glycol) methyl ether-b-poly(D,L-lactide) (PEgmE-b-PDLLA) was chosen to generate a PEG corona on the resulting NPs, previously added into the organic phase during the NP fabrication (Fig. 5b). The size of NPs was set over the range of 140–230 nm by regulating both compositions and the flow rate of the phases. Furthermore, the drug release investigations of DOX showed that the drug release is influenced by four parameters: a) (co)polymer molecular weight, b) their composition, c) the presence of TA and d) the size of the NPs. In vitro tests toward breast cancer cells (MCF-7) have shown that among all formulations, the NPs composed of Dox-loaded PCL/TA show a higher efficiency to inhibit the cell proliferation of MCF-7 cells.88 Although great efforts have been made to design new chips and synthesize new synthetic and natural polymer-based NPs, not all nanosystems are easily scalable from an industrial point of view, both because they can involve chemical modifications of the raw material and because the design of the chips cannot always be reproduced on a large scale. Furthermore, not all polymer-based NP systems exhibit high drug encapsulation efficiency. For these reasons, in the next few years, microfluidics-related research trends should be focused on the production of chemically unmodified polymer-based NPs fabricated by using a simple chip design in order to achieve a higher drug encapsulation efficiency than those fabricated previously and simple industrial scale-up.
3.2. Lipid-based nanoparticles
Lipid-based NPs, besides liposomes, have a variety of possible applications such as cosmetics, food/nutrition, nutraceuticals, drug delivery, and sustained release of active compounds and represent efficient delivery systems for nucleic acids.89 In particular, small interfering RNAs (siRNAs), a class of double-stranded RNA molecules, typically 20–25 base pair nucleotides in length, are the most commonly investigated nucleic acids for gene therapy.90 Gene therapy involves any procedure intended to treat or alleviate a disease by genetically modifying the cell of a patient. The siRNA acts within the RNA interference (RNAi) pathway, interfering with the expression of specific genes with complementary nucleotide sequences by destroying mRNA (messenger RNA) after transcription, preventing protein synthesis within the target cells. Thus, the appropriate design of siRNA could theoretically allow the silencing of any gene in the body, providing tremendous therapeutic potential in cancer therapy.91 However the low transfection rates of naked RNAs, as well as their rapid degradation by serum endonucleases and anionic repulsions with cell membranes, are only some of the most challenging limitations in their delivery.92 To overcome these limitations, RNAs are encapsulated in LNPs by the microfluidic method (MM) and bulk method (BM), in which a lipid solution in ethanol is mixed with an aqueous solution (e.g. acetate buffer solution) containing the nucleic acid to produce LNPs via self-assembly.93 The development of specialized ionizable amino lipids has attracted scientific attention because they can be set to the delivery needs of the siRNA molecule leading to enhanced activity of LNPs–siRNA.94,95 Furthermore, the advent of rapid-mixing methods has accelerated their commercial success and the clinical translation employment of LNPs.96 In particular, by employing rapid-mixing methods such as a staggered herringbone mixer, an encapsulation efficiency, about ∼100%, and a single and uniform population of LNPs could be achieved from the lab to the industrial scale.76,97 A new microfluidic architecture composed of ladder-designed flow resistors and staggered herringbone micromixers (SHMs) out of the parallelized microfluidic device (PMD) (Fig. 6) was developed and compared with a microfluidic single-channel device and the usual bulk mixing method to produce LNPs encapsulating either siRNA for in vitro screening cell lines or mRNA or siRNA for gene expression or in vivo gene silencing.98
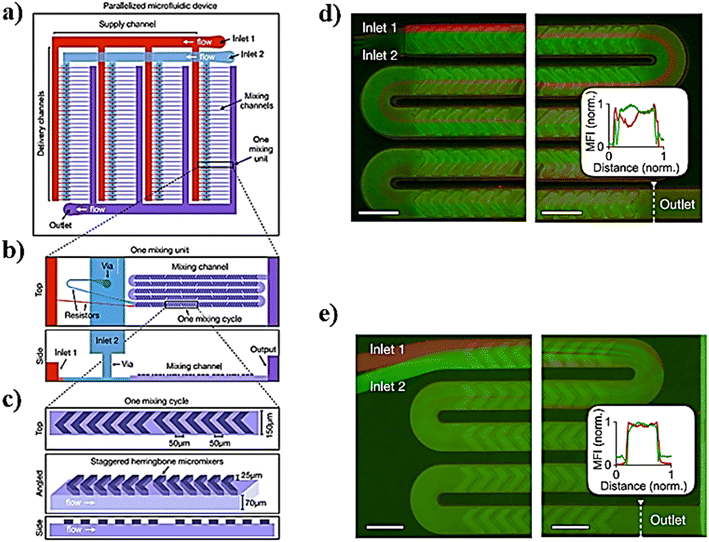 |
| Fig. 6 a) Schematic representation of the parallelized microfluidic device (PMD) for the design of this device, consisting of an array of 4 rows of 32 SHMs, each connected to layers of channels that deliver and collect fluid from each device in the array. b) Example of an individual mixing unit from a different angle. c) Example of an individual mixing cycle constituting the mixing unit. d and e) To validate the parallelized microfluidic design, fluorescence images of mixing in channels were taken. Scale bars: 200 μm. Image adapted from ref. 98. | |
In particular, LNP self-assembly had to be achieved in the device and, therefore, luciferase siRNA was rapidly mixed with a solution of lipids (i.e. C12-200, phospholipid 1,2-dioleoyl-sn-glycero-3-phosphoethanolamine (DOPE), cholesterol, and lipid–PEG). Besides, mRNA LNPs were produced likewise, by mixing luciferase mRNA with the previous solution of lipids. In the end, by comparing both the MM and BM, the results showed how the PMD produced LNPs at a large scale with dimensions of ∼70 nm and capable of achieving substantial in vivo siRNA and mRNA delivery, comparable to results obtained for a single channel microfluidic device. Li et al. developed transferrin-conjugated lipid NPs (Tf-LNPs) by using a staggered herringbone micromixer which were compared with those using a multi-step bulk method. Briefly, Tf–PEG–Chol was synthesized using a method described elsewhere. siRNA-loaded LNPs were fabricated by both the MM and BM. Lipids such as 1,2-di-O-octadecenyl-3-trimethylammoniumpropane (DOTMA), 1,2-dioceyloxy-3-dimethylaminopropane (DODMA), phosphatidylcholine (PC), cholesterol (Chol) and mPEG–Chol were dissolved in ethyl alcohol as the lipid solution, and siRNA was dissolved in HEPES-citrate buffer at pH = 4. Fig. 7 shows the microfluidic chip composed of two sides: in the first section, the internal phase was composed of lipid solution while the external phase was composed of siRNA solution in which chaotic mixing of the solutions was achieved by the staggered herringbone micromixer structure; subsequently, the second section, composed of the Y-junction with the second external phase, was composed of HEPES buffer or Tf–PEG–Chol solution.
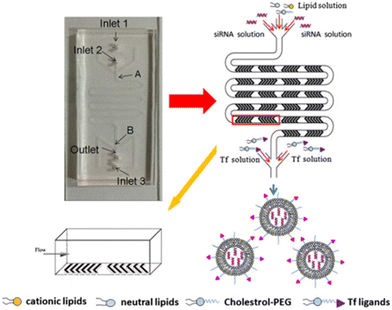 |
| Fig. 7 Schematic representation of Tf-LNP fabrication using the staggered herringbone micromixer. Reprinted with permission from ref. 99. Copyright 2016. Elsevier. | |
Physicochemical characterization demonstrated that Tf-LNPs-MF showed smaller size (∼132 nm) and more uniform structures compared to LNPs produced by the multi-step BM (∼152 nm). Furthermore, the in vitro biological test on HepG-2 cells demonstrated efficient internalization of Tf-LNPs fabricated by the MM as well as greater tumor inhibition in vivo, achieved by injection of Tf-LNPs-siRNA by the MM and free siRNA intravenously into mice, demonstrating that the Tf-LNP MM had higher siRNA delivery efficiency both in vitro and in vivo.99 In the last few decades, LNPs have been also commonly used in drug delivery; however, in LNPs, as well as in liquid nanoemulsions for parenteral nutrition, a controlled drug release is quite difficult to achieve, due to the small size of the carriers, as well as due to their liquid state, leading to a rapid release of the drug.100 Thus, among colloidal systems with characteristic dimensions between 10 and 1000 nm, solid lipid nanoparticles (SLNs) were introduced at the beginning of the 90s as potential alternative carrier systems able to encapsulate with higher efficiency lipophilic drugs such as DOX,101 ciprofloxacin,102 tetracaine, etomidate and prednisolone.103 Furthermore, SLNs can be used like LNPs as delivery systems for immunotherapy, gene therapy, or prophylactic vaccines, by encapsulating free nucleic acids,104 as their electron-dense core of nucleic acid/lipid complexes can easily grant an efficient intracellular delivery of such nucleic acids.105 SNLs can be seen as an evolution from parenteral nutrition emulsions, where the oil phase composed of a liquid lipid has been replaced with a lipid that is solid at room temperature (e.g. fatty acids, purified triglycerides, glyceride mixtures, etc.).106 In fact, they are usually produced with a variety of lipids: from common triglycerides (e.g. tricaprin,107 trilaurin103 and tripalmitin108) to steroids (e.g. cholesterol) and to waxes (e.g. cetyl palmitate).109 Moreover, SLNs take advantage of their solid core in order to maximize the drug release time through diffusion from the lipidic nucleus to the outside. Different from LNPs, a solid nucleus can prevent drugs from clearance by the reticuloendothelial system, maintaining all the benefits of the liquid nanoemulsion.110 Eventually, SNLs have been proven to be capable of controlling/targeting drug release, improving the stability of both lipophilic and hydrophilic drugs, granting effective biodegradability and excellent biocompatibility, in addition to great ease in scaling up and sterilizing.111,112 However, SNLs show some drawbacks, with some of them potentially vitiating their massive use: SNLs present a poor drug load capacity and a frequently occurring drug expulsion, following lipid polymorphic transition.113 Early SNL production involved the precipitation of LNPs through bulk mixing by extrusion, pipette mixing, or other methods.114 Generally BMs fabricate NPs with variable physicochemical and biological properties, showing a wide dimensional range, averagely above 100 nm, which are therefore incompatible with numerous tissue fenestrations, as the size influences in vivo biodistribution, uptake, and clearance.93,115 Meanwhile MM-produced SLNs showed a very narrow PDI, together with generally uniform and controlled physiochemical properties thanks to the intrinsic control over small volumes flowing in the channels,116 granting an efficient tunability of drug encapsulation, loading efficiency and release rate.117 Besides, the microfluidic architecture can be interestingly selected or designed, in order to have an ad hoc reagent flow.118,119 In a recent study, two different microfluidic devices were assembled from borosilicate glass capillaries and glass rods to produce cetyl palmitate-based SLNs.120 In the first one, the end of the cylindrical glass capillary was tapered using a micropipette puller; in contrast, device 2 shows a three-port valve connected after device 1, in which an immiscible fluid such as air can flow to maximize mixing efficiency (Fig. 8).
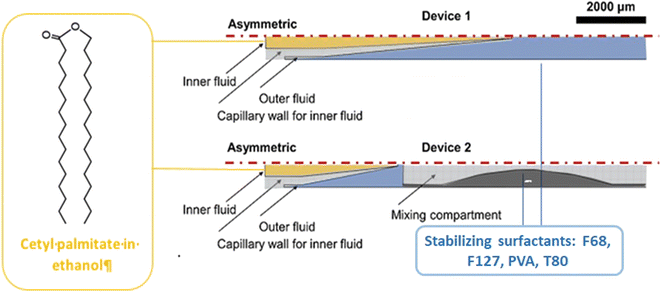 |
| Fig. 8 Schematic representation of microfluidic devices 1 and 2. As for device 1, the outflow is collected and cooled; for device 2, the outflow is further connected to a three-port valve with continuous air pumping (mixing compartment). Image adapted from ref. 120. | |
Briefly, SLN nanoprecipitation with a co-flow geometry was obtained by using cetyl palmitate and DSPE-PEG as lipid inner fluid, dissolved in a 95% ethanol solution, while the outer continuous fluid consisted of an aqueous solution containing stabilizers. The effect of different parameters such as variation in the flow rate, flow speed, type and concentration of surfactants was studied. It was noted that using Pluronic F127, by increasing its concentration, the size of the SLNs improved, while with Pluronic F68 and poly(vinyl alcohol) (PVA), the trend was the opposite. Instead, Pluronic F68-based SLNs formed smaller particles than PVA and Pluronic F127. The best combination of parameters was proved to be the one involving the surfactant F68 at 2% w/v, with a flow rate of 5
:
15, fabricating SLNs with a diameter of ∼180 nm and a PDI value of ∼0.23. Clearly, device 2 can fabricate smaller SNLs by means of its higher mixing efficiency. Moreover, by additionally decreasing the lipid concentration up to 10 mg ml−1, the diameter further decreased to 178 nm, keeping the same PDI. Subsequently, SLNs were loaded with sorafenib (SFN) and paclitaxel (PTX) drugs for cancer therapy. The drug loading (DL) and encapsulation efficiency (EE) were evaluated: the PTX-SLN best drug concentration was seen to be 0.75 mg mL−1, with an EE of 54% and a DL of 1.4%. While for SFN-SLNs, the best concentration was found to be 0.5 mg mL−1 with an EE of 79% and a DL of 1.04%. Besides polymer-based NPs, LNPs and SLNPs have been used as effective carriers for a variety of anticancer drugs. However, there are still some challenges such as the chip design and drug encapsulation efficiency; the latter represents a key issue if the scientific community wants to use microfluidic-based NPs as carriers for cancer treatment. For this reason, in the next few years the direction of research, in our opinion, should be focused on the uses of herringbone staggered micromixer chips that can lead to both high drug encapsulation efficiency and possible industrial scalability.
3.3. Structured nanoparticles
The development of lipid-based and polymer-based nanocarriers was reported to be an advantageous approach of designing novel and more efficient carriers for targeting and controlling drug release.121,122 In this context, the preparation of lipid–polymer hybrid nano-systems (LPHNSs) has emerged as a valuable strategy to overcome some of the drawbacks that lipid nanosystems (high polydispersity, low biphasic release, physicochemical and biological stability) and polymer nanosystems (reduced drug loading and encapsulation efficiency) exhibit taken individually.123 As well as other kinds of nanocarriers, in the last few years the introduction of MMs allowed overcoming some problems that traditionally affect BMs for the preparation of LPHNSs.124 In this paragraph are reported examples of microfluidic chips optimized to design single-step processes for the fabrication of LPHNSs,125 or to prepare LPHNSs with microscopic architectures that would be inaccessible through traditional BMs.126 LPHNSs for drug release based on PLGA and 1,2-distearoyl-sn-glycero-3-phosphoethanolamine-N-[methoxy(polyethylene glycol)-2000] (DSPE-PEG2000) were prepared by co-flow nanoprecipitation.125 The microfluidic chip was fabricated with borosilicate glass capillaries, to obtain a device resistant to organic solvents. LPHNSs were loaded with the anticancer drug sorafenib (SFN), a model drug, and were compared with LPHNSs prepared with the nanoprecipitation BM. A PLGA acetonitrile solution (inner fluid) and a lipid ethanol solution (outer fluid) were injected into the microfluidic system by means of syringe pumps at an inner/outer flow rate ratio from 1
:
5 to 1
:
50 mL h−1. The microfluidic system and the LPHNS preparation process are schematized in Fig. 9.
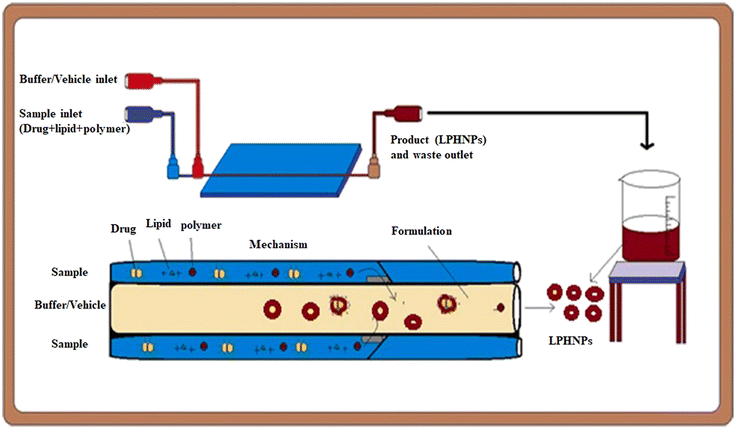 |
| Fig. 9 Scheme of the setting of the microfluidic apparatus and of the LPHNS fabrication. Reprinted with permission from ref. 125. Copyright 2020. Elsevier. | |
The size (320–191.8 nm) and the PDI (0.163–0.1) of LPHNSs prepared with the microfluidic method were found to decrease as the ratio decreased, resulting in more sensitivity to the fabrication conditions compared to the size (∼200 nm) and PDI (∼0.2) of LPHNSs prepared with the BM. The drug encapsulation efficiency of the microfluidic-prepared LPHNSs (88–95%) resulted in higher and more sensitivity to fabrication conditions than that obtained from the BM (88–89%). The size, PDI and ζ potential of the microfluidic-prepared LPHNSs were stable over 10 days in three different media (PBS, RPMI, DMEM). LPHNSs fabricated with the microfluidic system exhibited a controlled release of the drug over 24 h, and were tested in vitro with breast cancer (MDA-MB-231) and prostate cancer (PC3) against which the NPs showed higher anticancer activity compared to the bulk method prepared NPs. Core–shell NPs based on polyethylenimine-graft-polycaprolactone (PCL-PEI) and lipids were prepared by means of a three-stage microfluidic chip for the delivery of small interfering RNA (siRNA).26 siRNA is deeply studied in the literature as a therapeutic agent against different kinds of degenerative and chronic diseases,126–128 but requires a suitable carrier to be administered, as it easily undergoes enzymatic degradation (RNAses) and elimination by kidneys. Traditionally nano-systems for the delivery of siRNA are prepared by means of BMs that involve the compaction of siRNA into nanoplexes by using cationic surfactants. These nanostructures showed a premature release of siRNA into the bloodstream, causing an immunogenic reaction. They also were proved to be directly toxic due to the residual positive charge. A MM was proposed for the production of core–shell NPs in which siRNA is enclosed in PCL-PEI reverse micelles, which are surrounded by a lipid neutral membrane. The microfluidic chip was specially designed for the fabrication of lipid/PCL-PEI/siRNA (LPS) NPs, with five inlets, two straight mixing channels, one double spiral mixing region and one outlet. The injection of all components was controlled by syringe pumps. The siRNA water solution was injected in the middle inlet, and at the first junction of the chip, it was mixed with a solution of PCL-PEI in DMSO, injected from the peripheral inlet. In this stage, PCL-PEI/siRNA complexes, due to the interaction between the cationic PEI moiety and the anionic siRNA, were formed. Two water streams were injected into the two side inlets, and they were mixed with the central flow at the second junction. In this stage, the PCL-PEI/siRNA was forced to arrange in reverse micelles because of the solvent-switching effect of DMSO/water. The water suspension of reverse micelles was stabilized by mixing at the third junction with the lipid solution in ethanol, containing cholesterol, DSPE-PEG2000 and 1,2-dioleoyl-sn-glycero-3-phosphoethanolamine (DOPE). The lipid solution was injected through the middle inlet. The final NP suspension was collected at the exit from the outlet. LPS was compared with lipid/micelle/siRNA (LMS) prepared using the same components. PEI-PCL micelles were prepared by means of the thin-film hydration method, mixed with siRNA and then coated with lipids. The microfluidic process and the comparison of in vivo transport of LPS and LMS are reported in Fig. 10.
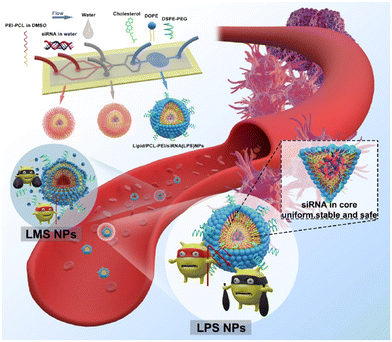 |
| Fig. 10 Representation of the microfluidic process and comparison of in vivo circulation of LPS and LMS. Reprinted with permission from ref. 26. Copyright 2020. American Chemical Society. | |
The size of LPS was smaller (d ∼ 120 nm) than that of LMS (d ∼ 200 nm), with a narrower PDI and greater size stability over 14 days. Furthermore, the microfluidic method produced NPs with a more negative zeta potential (−8.8 mV LPS, −1.2 mV LMS) and higher encapsulation efficiency (LPS 98%, LMS 79%). Finally, LPS exhibited improved biological features, with evident downregulation of the estimated glomerular filtration rate (eGFR) mRNA and of the protein expression level, and improved action against human prostate cancer PC-3, tested in vitro and in vivo. The Tesla mixer is another microfluidic architecture, composed of repeating units of channel diversions and it blends for a rapid mixing widely used to fabricate lipid-based NPs (Fig. 11).
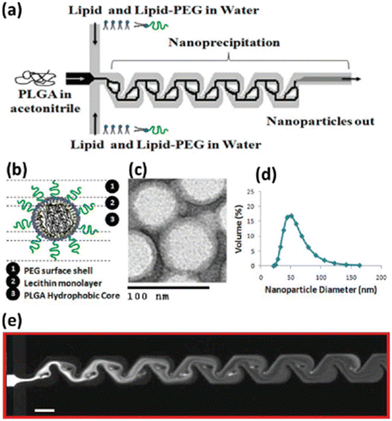 |
| Fig. 11 Nanoprecipitation of lipid–polymeric NPs: (a) schematic representation of Tesla structures for the production of hybrid lipid–polymeric NPs, (b) figure of a NP. (c) TEM image of NPs, (d) average size distribution of NPs d ∼ 40 nm. (e) Solvent mixing in the Tesla micromixing structures with a scale bar of 100 μm. Reprinted with permission from ref. 129. Copyright 2010. American Chemical Society. | |
The Tesla mixer was employed to fabricate in a single step hybrid lipid–polymer NPs by nanoprecipitation; in particular, the central stream consisting of PLGA solubilized in acetonitrile focused between lipid streams consisting of lecithin and lipid–PEG in water. By varying the polymer and lipid, or functionalizing the lipid reagents, different NP sizes (35–180 nm), charge (−10 to +20 mV), and stability were observed which could be applicable for drug delivery in the cancer therapy field.129 Generally, the one-step MMs are favored compared to the two-step method thanks to their simplicity; moreover, the crucial parameter in order to achieve the control of physicochemical properties, drug encapsulation efficiency and the release kinetics of NPs is based on the lipid–polymer ratio and the colloidal stability due to steric hindrance provided by polymer chains. Furthermore, even if the emulsification–solvent evaporation method (ESEM) represents one of the best methods to produce these nanosystems, great efforts have been and will be made to shift to the one-pot nanoprecipitation method that can provide higher drug encapsulation efficiency, as reported in Table 1, and industrial scalability compared to the ESEM.
3.4. Inorganic-based nanoparticles
Inorganic-based NPs are widely used in a variety of fields, such as optoelectronics,130 imaging,131 sensing,132 catalysis133 and especially in the drug delivery field,134 due to their unique physicochemical properties on the nanoscale. Thanks to the advantages mentioned above, microfluidic technologies have been widely employed for the fabrication and surface modification of inorganic nanomaterials, such as metal and metal/metal composite NPs,135 silica NPs,136 metal–organic frameworks (MOFs)137 and quantum dots (QDs).138 In particular, in the synthetic procedure, the monodispersity of inorganic-based NPs is completely dependent on the reaction kinetics, their mixing, the reaction fluids, and the temperature. All these features are precisely controlled in microfluidic devices.139 The inorganic-based NPs are non-toxic, biocompatible, hydrophilic, and highly stable and are widely employed for the design of new drug delivery systems in order to enhance target efficiency to the cancer cells and reduce adverse effects.140 Some examples, reported in the literature in the last two years, based on inorganic-based NPs have been selected and described as follows. A pH/redox-triggered mesoporous silica nanoparticle (MSN)-based nanoplatform has been fabricated for DOX/paclitaxel (DOX/PTX), in which PTX is covalently linked to the DOX-loaded MSNs through a disulfide bond. This modification has been applied for two reasons: i) to improve the PTX loading and ii) PTX and the linker act as a redox-sensitive gateway to control the release profile of both drugs. Afterward, polystyrene sulfonate (PSS) is coated onto DOX-loaded MSN-PTX in a 3D microfluidic co-current focusing device, Fig. 12, using two miscible liquids (ethanol and water) which are injected into the microfluidic device separately, by electrostatic interaction to become acidic pH-responsive and to neutralize the ζ potential in order to reduce the non-specificity endocytosis of healthy cells. The diameter d of DOX-loaded/MSN/PTX/PSS NPs was ∼148.93 nm.
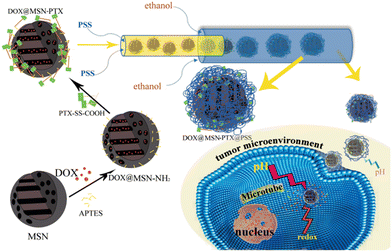 |
| Fig. 12 Fabrication of DOX-loaded MSN-PTX/PSS by means of microfluidics. The PSS layer swells under an acidic tumor microenvironment; thus, the MSN becomes positively charged and can be internalized by tumor cells via endocytosis. Yan J. et al.141 | |
In vitro cytotoxicity assays performed on cancer cell BT549 and healthy breast cell MCF-10A, showed that the NPs can selectively release DOX and PTX and eliminate cancer cells with a negligible effect on the healthy breast cells, thanks to the acidic microenvironment in cancer cells.141 Hao et al. developed a simple strategy to fabricate ellipsoidal mesoporous silica nanomaterials (EMSNs) with ordered parallel channels employing cetyltrimethylammonium bromide and tyrosine as structure-directing agents and tetraethyl orthosilicate as a silica precursor in dilute ammonia solution. In order to produce the hollow counterparts of EMSNs employing phosphate-buffered saline (PBS) as the etching agent and bovine serum albumin (BSA) protein as the surface protective coating, a miniaturized microfluidic device with spiral-shaped channels was used and is reported in Fig. 13.
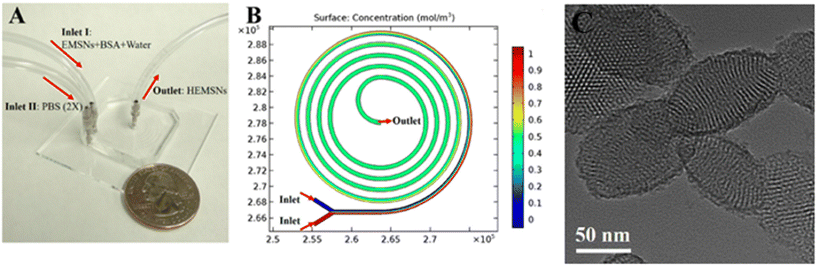 |
| Fig. 13 A) Microfluidic chip for the fabrication of EMSNs. (B) COMSOL simulation result of mixing in the microfluidic spiral channel, and their morphologies. Reprinted from ref. 142. | |
The microfluidic device was composed of a five-run spiral microchannel with two inlets for mixing the reactants and one outlet for collecting the resultant nanomaterials. Briefly, EMSNs and BSA protein were dispersed in water as one inlet flow and an equivalent volume of PBS as the other inlet flow. Subsequently, DOX was loaded by mixing with nanomaterials under stirring under dark conditions. Cytocompatibility assays showed that DOX-loaded EMSNs had low cell viability of SK-BR-3 compared to unloaded EMSNs.142 Li et al. developed sub-10 nm organic aggregation-induced emission (AIE) particles using four different AIE luminogens (AIEgens) with emissions from green to the second near-infrared window (NIR-II). The AIE QDs were synthesized via nanoprecipitation by means of a microfluidic chip composed of three inlets, a mixing channel and a double spiral mixing channel and one outlet (Fig. 14a).
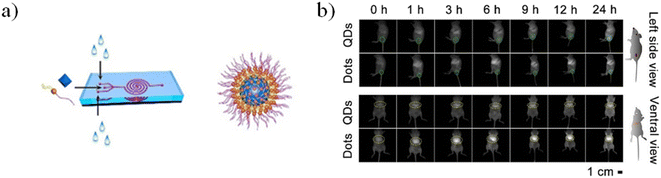 |
| Fig. 14 a) Microfluidic fabrication of AIE QDs and the structure of AIE QDs. b) NIR-II fluorescence images of 4T1 tumor bearing mice treated with Ttb QDs or Ttb dots for 24 h from the side view and the ventral view. Green circles indicate the tumor. Yellow circles indicate the liver. Reprinted with permission from ref. 146. | |
Subsequently, the fluorescence emission of these AIEgens ranges from green to NIR-II, including the four reported AIEgens 4,7-bis[4-(1,2,2-triphenylvinyl)phenyl]benzo-2,1,3-thiadiazole (Bt), 4,7-bis(4-(1,2,2-triphenylvinyl)phenyl)-spiro[benzo-[d]imidazole-2,1′-cyclohexane] (Bi), 2,3-bis(4-(phenyl(4-(1,2,2-triphenylvinyl)phenyl)amino)phenyl)fumaronitrile (Fn), and 4,7-(bis4-(4-octylthiophen-2-yl)-N,N-diphenylaniline)benzo[1,2-c:4,5-c′]bis([1,2,5]thiadiazole) (Ttb), which are synthesized as reported elsewhere.142–145 Thanks to microfluidic mixing, the hydrophobic fatty acid chains of amphipathic Dspe-PEG2k can link to the aggregated hydrophobic AIE core and the hydrophilic PEG chains allow the QDs to be soluble in aqueous solutions. These AIE dots, produced by a microfluidic chip, show quantum size and their name was AIE quantum dots (QDs) in order to distinguish them from the normal AIE dots with a diameter >25 nm. In particular, the quantum size effects, >25 nm, are significant for the biological properties. Indeed, the AIE QDs lead to more efficient cell internalization and imaging without surface modification using any membrane-penetrating peptides than AIE dots. They demonstrated that the AIE QDs with NIR-II fluorescence could improve the tumor-targeting compared to >25 nm AIE dots and evasion from the liver. Moreover, NIR-II AIEgens have been employed to demonstrate that AIE QDs can achieve high contrast at the tumor as small as 80 mm3 (Fig. 13B) and evade the liver more efficiently than AIE dots. AIE QDs hold to offer the possibilities for precise diagnosis of the solid tumor in clinical medicine with much lower off-targeting to the liver than AIE dots.146 Balachandra et al. developed an integrated microfluidic chip to synthesize an aptamer-modified biozeolitic imidazolate framework (BioZIF-8) to target the lymph nodes and tumor. Briefly, the microfluidic chip was composed of a three-spiral mixing channel to synthesize ligand-functionalized BioZIF-8 in two steps (Fig. 14a). The first step the ZIF-8 was synthesized, in which ZIF-8 encapsulating biomolecules (bovine serum albumin, small interfering ribonucleic acid, and DOX), while in the second step, the BioZIF-8 was MOFs surface-functionalized with two different aptamer ligand, RNA aptamer and DNA, in order to target the lymph nodes and tumor. In particular, the anti-CCL21 DNA aptamer targets the chemokine ligand 21 of a cytokine in the T cell-rich region of lymph nodes, while to target the tumor, an A10 RNA aptamer is employed.147 The A10 RNA aptamer recognizes the extracellular domain of the prostate-specific membrane antigen (PSMA) on the surface of prostate cancer cells.148 The preparation of the aptamer functionalized BioZIF-8 MOFs employing a one-step, one-chip microfluidic approach is reported in Fig. 15a.
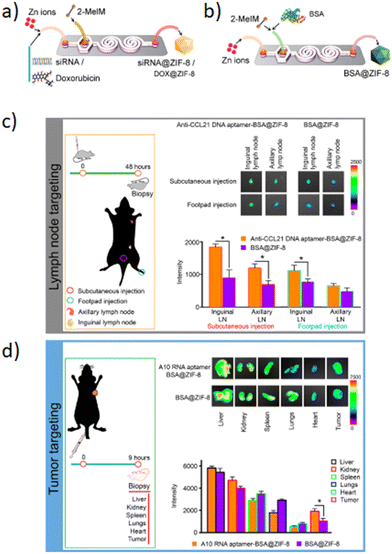 |
| Fig. 15 The microfluidic chip for the fabrication of: a) BioZIF-8, b) siRNA-ZIF-8 and DOX-ZIF-8 MOFs; c) and d) aptamer-functionalized BSA–ZIF-8 targets the lymph node and tumor. Reprinted with permission from ref. 149. Copyright 2021. American Chemical Society. | |
The in vivo evaluation was aimed at evaluating the targeting efficiency of aptamer-functionalized BSA–ZIF-8 MOFs to the lymph nodes (Fig. 15c) and tumor in a mouse model (Fig. 15d). The targeting efficiency from the lymph nodes showed a significant increase for the anti-CCL21 DNA aptamer–BSA–ZIF-8 MOF-treated mice compared to those without an aptamer as well as the zinc concentration (Fig. 15c). Furthermore, also in the case of prostate cancer cells, the targeting efficiency was higher for A10 RNA aptamer-functionalized FITC–BSA–ZIF-8 MOFs than those unfunctionalized. According to the targeting efficiency data, the tumor data show a higher accumulation in the tumor when the mice are treated with A10 RNA aptamer–BSA–ZIF-8 MOFs than those without an aptamer (Fig. 15d). These data are also consistent with the zinc concentration for the A10 RNA aptamer–BSA–ZIF-8 MOF-treated mice.149 Although in recent years scientific research has been focused on different types of inorganic NPs, i.e. QDs, silica, metal/composite NPs and MOFs, the microfluidic-based synthetic strategies of inorganic NPs present some drawbacks such as the low control of the synthetic mechanism, the liquid evaporation related to the high temperature achieved during the synthetic procedures, the low yield that until now stops at grams per hour, the morphology modulation and industrial production. In order to improve the drawbacks mentioned above, computational and predictive analyses for an integrated design methodology of new chips can be an effective strategy to develop inorganic NPs with precise control of the synthetic mechanism and in order to achieve nanocarriers with high performances. Furthermore, a hotplate adapter for the chips was developed with dolomite in which the chip is located between two plates hermetically sealed in order to avoid solvent evaporation. Based on these findings, in the future, with the rapid development of microfluidic technologies, based on the design of the new chips, a wide set of functional inorganic NPs, with controlled synthetic mechanisms and physicochemical properties such as shape and size, are expected to be realized in order to develop new nanocarriers for cancer treatments.
3.5. Metal-based nanoparticles
Metal-based nanoparticles (MNPs) composed of pure metals, such as Fe,150,151 Co,151 and Ni,152 or composed of alloys, such as FePt,153 NiPt,154 and NiPd,155 were fabricated for hyperthermia application thanks to their magnetic properties. Ferromagnetic nano-sized NPs exhibit superparamagnetic behavior, presenting a single magnetic domain.156 Under an external alternating magnetic field, these magnetic NPs quickly reoriented their magnetic moment, leading to a loss of energy that can be used to heat the surrounding tissues and locally destroy tumor cells.157 Once the magnetic field is removed, the NP net magnetic moment vanishes and the NPs act like a non-magnetic material.158 Nevertheless, there are some limitations associated with the use of MNPs, because of their tendency to aggregate and precipitate when they are introduced into the blood vessels, leading to low stability and biocompatibility.159 To overcome these issues, MNPs are often covered with an organic biocompatible coating, generating metal–organic nanoparticles (MONPs).160 Furthermore, MNPs and MONPs can be loaded with anticancer drugs for cancer treatment and used as nanocarriers characterized by high versatility and high drug delivery efficiency.161 Some examples describing MNPs fabricated by microfluidic devices are described as follows. Biomimetic diethyldithiocarbamate copper (Cu(DDC)2) NPs superficially decorated with bovine serum albumin (BSA) were prepared for breast cancer therapy by using a 3D-printed microfluidic device. The precise control of the mixing process and massive production can be achieved, leading to easy clinical translation and future commercialization. The BSA employment on the NP surface is crucial for NP stabilization because it can act as a targeting ligand to bind with some proteins (SPARC receptors) overexpressed by tumor cells. For this experiment, a mixing microfluidic device was prepared with a 3D printer (Creality CR-10) using PLA as a printing material. Briefly, BSA/Cu(DDC)2 NPs were prepared by dissolving sodium diethyldithiocarbamate trihydrate (DDC-Na) and copper chloride (CuCl2) with two BSA containing aqueous solutions at 1% w/v concentration, respectively. Then, DDC-Na and CuCl2 solutions were injected into the microfluidic device through two inlets by a syringe pump (Fig. 16a). Different experiments were conducted at different flow rates (0.5, 1, 2 ml min−1) and the molar ratio between DDC-Na and CuCl2 solutions was fixed to 2
:
1.
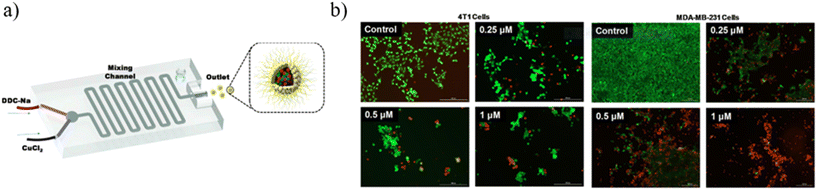 |
| Fig. 16 (a) Schematic illustration of the mixing microfluidic device adopted to fabricate BSA/Cu(DDC)2 MONPs. (b) MTT assay results of 4T1 cells and MDA-MB-231 cells treated with BSA–Cu(DDC)2 for 48 hours. Reprinted with permission from ref. 162. Copyright 2019. Elsevier. | |
The outlet solution containing MONPs was purified by centrifugation and subsequent filtration. The use of the microfluidic mixer device allows for obtaining NPs with a size of around 65 nm, a PDI of around 0.2 and a ζ potential of around −30 mV, because of the presence of BSA covering the NP surface. Furthermore, thanks to the BSA stabilizing action, the NP size remains constant over the first 60 hours. The enhanced mixing efficiency guaranteed by microchannels leads to a high yield of NP production (95%). The loaded drug percentage is a function of the flow rate into the device microchannel. Indeed, by increasing the flow ratio from 0.5 ml min−1 to 2 ml min−1, the drug concentration in the MONPs increases from 1.3 mg ml−1 to 1.9 mg ml−1 and the drug loading percentage increases from 66% to 95%, thanks to the better mixing efficiency that the mixing microdevice has when the flow rate becomes higher. Moreover, the microfluidic device developed for these experiments allows for increasing the NP production rate, managing to fabricate 240 ml of BSA/Cu(DDC)2 NPs (2 mg ml−1) per hour. Finally, in vitro experiments were performed to test the BSA/Cu(DDC)2 antitumor activity. For a drug concentration of 1 μM, after 48 hours MTT tests showed a cell viability of around 20% for MDA-MB-231 cells and of 0% for 4T1 cells (Fig. 16b).162 A polymethyl-methacrylate (PMMA) microfluidic chip was employed to fabricate silver nanocarriers embedded by CS and loaded with a Calotropis procera extract (CpE), which seems to have biological properties, among which is anti-cancer activity. Chitosan/silver NPs (CS NPs) were fabricated by means of a T-junction microreactor device reported in Fig. 17.
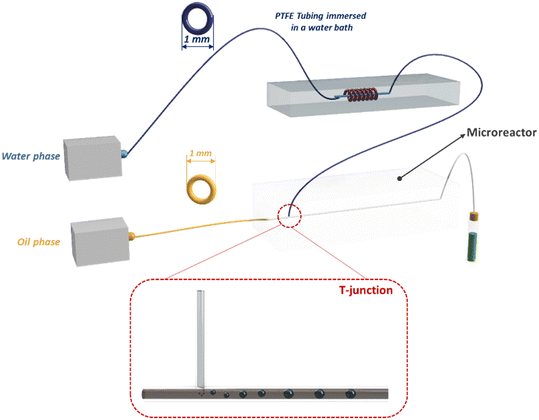 |
| Fig. 17 Experimental setup for fabrication of CpE loaded CS NPs. | |
The continuous oil phase (canola oil) was fluxed through the straight channel by means of a syringe pump. The dispersed aqueous phase (reaction mixture) was prepared by mixing 1.5% of CS solution and a 4 mM AgNO3 solution at a volumetric ratio of 4
:
1, respectively. 40 mg of CpE was dissolved in a polar solvent (2
:
3, ethanol
:
dH2O) and it was added to an aqueous solution at different volumetric ratios (1, 2, 4 and 6). The reaction mixture was heated by flowing through a helically coiled polytetrafluoroethylene (PTFE) tube immersed in a temperature-controlled water bath at 90 °C. The reaction mixture heating is crucial to activate the effective capping efficiency of CS on silver. After the reaction mixture was injected through the perpendicular channel to form the microemulsion, which was collected at the outlet of the chip, for some samples, 10% of NaOH was added into the sample for further reduction. The CS NPs obtained were centrifuged and the resulting NPs were lyophilized for subsequent analysis. The SEM micrographs showed different morphologies between loaded and unloaded CS NPs. Indeed, unloaded CS NPs revealed an irregular shape with a rough surface, probably due to some agglomeration of silver NPs. Meanwhile, silver NPs were quite visible on the smooth surface of CS and CpE, after CpE loading. This difference could be interpreted considering that CpE has a significant role in determining and stabilizing NP surfaces. Therefore, SEM analysis pointed out that samples fabricated without the reaction mixture heating showed a completely different morphology (flower-like), consisting of interconnected nano-needles of Ag2O with a mean diameter of 161 ± 25 nm. The drug encapsulation efficiency was found to be higher for heated samples (77%) than for samples fabricated at room temperature (57%), thanks to the better encapsulation efficiency of CA NPs compared to the Ag2O nano-needles. H2O2 antioxidant assays were conducted to evaluate the CA NP antioxidant activity. The scavenging ability of CpE CS-2 NPs (CpE loaded CS NPs in which CpE was added to the reaction mixture at a volumetric ratio equal to 2) was higher as compared to that of free CpE at low concentration, thus the presence of CS microcarriers increases the CpE scavenging proficiency. Finally, in vitro assays were performed to evaluate the antitumor activity of loaded CS NPs against 4T1 cells and demonstrated that CS NPs increase the cytotoxicity of free CpE. In particular, CpE CS-2 NPs showed better results in terms of cytotoxicity, reducing the cell viability up to 6.34% and 4.63% at concentrations of 80 μg ml−1 and 100 μg ml−1, respectively, after 24 hours.163 Despite the numerous advances made in the fabrication of MNPs through microfluidics,164 great efforts should be made to simplify the MNP fabrication and improve their drug encapsulation efficiency as well as physicochemical property control in order to be efficient nanocarriers used in cancer therapy. An effective strategy, to overcome these drawbacks, could be based on: 1) the design of simple chips, supported by computational modeling and 2) the employment of real-time characterization in order to monitor all variations during the MNP structure formation. Therefore, with the use of new chip designs, it could be possible to achieve nanocarriers with high drug encapsulation efficiency and high morphology control in order to achieve their fast industrial scalability.
4. Conclusions and future perspectives
We have highlighted some of the most important microfluidic technologies employed for the production of NPs; in particular, the microfluidic platform offers a higher level of control of physicochemical properties, such as size, shape and morphology, in the production of nanoparticles than the conventional fabrication methods. The precise control of the physicochemical properties is a key parameter to design and develop new technology platforms for the development of drug delivery systems for cancer therapy. Indeed, the success of microfluidic technology is based on: (i) rapid mixing in microfluidic channels in order to obtain monodisperse nanoparticles with a high yield; (ii) the precise control of physicochemical properties to obtain batch-to-batch reproducibility; (iii) the integration of multiple fabrication procedures in a single microfluidic device to allow for the fabrication of nanoparticles with desired structures in one step. Microfluidic devices, with all these features, represent an ideal system for controllable fabrication and practical applications of a variety of nanoparticles. Although many efforts have been made in the last decade, it is clear from the current state of the art of microfluidic research for NP fabrication that not all drug delivery systems can be used for cancer treatments due to the low drug encapsulation efficiency or due to the not easy up-scalability to the industrial scale. Therefore, in order to face the drug load capacity and frequent drug expulsion, different types of enhancements such as 1) the choice of the raw materials such as the production of nanoparticles without any chemical modifications, 2) the one-pot synthesis and 3) the design of the chips must be considered. Indeed, the design of ad hoc microfluidic device architectures can improve both the drug encapsulation efficiency and the production rates of NPs. For instance, a device composed of parallel channels or several mixing chambers can be used as an effective strategy to improve industrial scalability. However, although the employment of microfluidic devices for nanoparticle fabrication is still under great exploration, research carried out in the last two decades, especially in the last five years, has demonstrated that microfluidics could facilitate the fabrication of nanoparticles for cancer therapies. Currently, the use of many NP-based materials in experimentation on animal models is stopped, but the new opportunities provided by microfluidics represent the possibility to move forward with the clinical translation. Thanks to the new advancements that are occurring in the design of both microfluidic technologies and nanomaterials, fabricated with microfluidics, the research activity, in this field, will inevitably provide new ideas in the future for producing new functional nanomaterials applicable in the cancer therapy field.
Author contributions
All authors contributed to the writing, discussion, and correction of this perspective.
Conflicts of interest
The authors declare no competing financial interest.
Acknowledgements
The authors acknowledge the research project “ADViSE: Antitumor Drugs and Vaccines From The Sea”-Por Campania FESR 2014–2020 with the AXIS 1 OO.SS. 1.2.2/1.1 CUP B43D18000240007.
References
- P. Couvreur, Adv. Drug Delivery Rev., 2013, 65, 21–23 CrossRef CAS PubMed.
- M. J. Mitchell, M. M. Billingsley, R. M. Haley, M. E. Wechsler, N. A. Peppas and R. Langer, Nat. Rev. Drug Discovery, 2021, 20, 101–124 CrossRef CAS PubMed.
- A. Bokare, A. Takami, J. H. Kim, A. Dong, A. Chen, R. Valerio, S. Gunn and F. Erogbogbo, ACS Omega, 2019, 4, 4650–4657 CrossRef CAS PubMed.
- S. Abalde-Cela, P. Taladriz-Blanco, M. G. de Oliveira and C. Abell, Sci. Rep., 2018, 8, 2440 CrossRef PubMed.
- K. Cho, X. Wang, S. Nie, Z. Chen and D. M. Shin, Clin. Cancer Res., 2008, 14, 1310–1316 CrossRef CAS PubMed.
- M. S. Ricci and W.-X. Zong, Oncologist, 2006, 11, 342–357 CrossRef CAS PubMed.
- K. H. Bae, H. J. Chung and T. G. Park, Mol. Cells, 2011, 31, 295–302 CrossRef CAS PubMed.
- J. Wang, D. Mongayt and V. P. Torchilin, J. Drug Targeting, 2005, 13, 73–80 CrossRef CAS PubMed.
-
M. E. Davis, Z. Chen and D. M. Shin, Nanoscience and technology: A collection of reviews from nature journals, 2010, pp. 239–250 Search PubMed.
- K. Y. Choi, H. Chung, K. H. Min, H. Y. Yoon, K. Kim, J. H. Park, I. C. Kwon and S. Y. Jeong, Biomaterials, 2010, 31, 106–114 CrossRef CAS PubMed.
- E. Blanco, A. Hsiao, A. P. Mann, M. G. Landry, F. Meric-Bernstam and M. Ferrari, Cancer Sci., 2011, 102, 1247–1252 CrossRef CAS PubMed.
- O. C. Farokhzad, J. Cheng, B. A. Teply, I. Sherifi, S. Jon, P. W. Kantoff, J. P. Richie and R. Langer, Proc. Natl. Acad. Sci. U. S. A., 2006, 103, 6315–6320 CrossRef CAS PubMed.
- A. N. Lukyanov, T. A. Elbayoumi, A. R. Chakilam and V. P. Torchilin, J. Controlled Release, 2004, 100, 135–144 CrossRef CAS PubMed.
- S. S. Aleksenko, A. Y. Shmykov, S. Oszwałdowski and A. R. Timerbaev, Metallomics, 2012, 4, 1141–1148 CrossRef CAS PubMed.
- J. D. Byrne, T. Betancourt and L. Brannon-Peppas, Adv. Drug Delivery Rev., 2008, 60, 1615–1626 CrossRef CAS PubMed.
- V. P. Torchilin, Adv. Drug Delivery Rev., 2006, 58, 1532–1555 CrossRef CAS PubMed.
- H. Wang, T. Ding, J. Guan, X. Liu, J. Wang, P. Jin, S. Hou, W. Lu, J. Qian and W. Wang, ACS Nano, 2020, 14, 14779–14789 CrossRef PubMed.
- K. H. Lee, G. L. Z. Yang, B. E. Wyslouzil and J. O. Winter, ACS Appl. Polym. Mater., 2019, 1, 691–700 CrossRef CAS.
- Y. Liu, G. Z. Yang, D. Zou, Y. Hui, K. Nigam, A. P. J. Middelberg and C. X. Zhao, Ind. Eng. Chem. Res., 2020, 59, 4134–4149 CrossRef CAS.
- N. M. Pinkerton, L. Behar, K. Hadri, B. Amouroux, C. Mingotaud, D. R. Talham, S. Chassaing and J. D. Marty, Nanoscale, 2017, 9, 1403–1408 RSC.
- X. C. Cui, J. Wang, X. Y. Zhang, Q. Wang, M. M. Song and J. L. Chai, Langmuir, 2019, 35, 9255–9263 CrossRef CAS PubMed.
- S. Maiz-Fernandez, L. Perez-Alvarez, L. Ruiz-Rubio, R. P. Gonzalez, V. Saez-Martinez, J. R. Perez and J. L. Vilas-Vilela, Polymers, 2019, 11(4) DOI:10.3390/polym11040742.
- S. Y. Lee, E. H. Hong, J. Y. Jeong, J. Cho, J. H. Seo, H. J. Ko and H. J. Cho, Biomater. Sci., 2019, 7, 4624–4635 RSC.
- R. Z. Wang, Y. Luo, S. H. Yang, J. Lin, D. M. Gao, Y. Zhao, J. G. Liu, X. Y. Shi and X. L. Wang, Sci. Rep., 2016, 6, 33844 CrossRef CAS PubMed.
- J. Zhao, Z. Y. Wan, C. C. Zhou, Q. Yang, J. X. Dong, X. Song and T. Gong, Pharm. Res., 2018, 35, 196 CrossRef PubMed.
- W. Wei, J. Sun, X.-Y. Guo, X. Chen, R. Wang, C. Qiu, H.-T. Zhang, W.-H. Pang, J.-C. Wang and Q. Zhang, ACS Appl. Mater. Interfaces, 2020, 12, 14839–14854 CrossRef CAS PubMed.
- H. Patil, X. Feng, X. Ye, S. Majumdar and M. A. Repka, AAPS J., 2015, 17, 194–205 CrossRef CAS PubMed.
- D. Chen, K. T. Love, Y. Chen, A. A. Eltoukhy, C. Kastrup, G. Sahay, A. Jeon, Y. Dong, K. A. Whitehead and D. G. Anderson, J. Am. Chem. Soc., 2012, 134, 6948–6951 CrossRef CAS PubMed.
- R. Ciriminna, A. Fidalgo, V. Pandarus, F. Beland, L. M. Ilharco and M. Pagliaro, Chem. Rev., 2013, 113, 6592–6620 CrossRef CAS PubMed.
- D. Desai, Y. A. Guerrero, V. Balachandran, A. Morton, L. Lyon, B. Larkin and D. E. Solomon, Nanomed.: Nanotechnol., Biol. Med., 2021, 35, 102402 CrossRef CAS PubMed.
- K. K. Zhai, X. P. Pei, C. Wang, Y. K. Deng, Y. Tan, Y. A. Bai, B. C. Zhang, K. Xu and P. X. Wang, Int. J. Biol. Macromol., 2019, 131, 1032–1037 CrossRef CAS PubMed.
- A. Fabozzi, F. Della Sala, M. di Gennaro, N. Solimando, M. Pagliuca and A. Borzacchiello, Polym. Chem., 2021, 12, 6667–6687 RSC.
- N. J. Hao, Y. Nie, Z. Xu, A. B. Closson, T. Usherwood and J. X. J. Zhang, Chem. Eng. J., 2019, 366, 433–438 CrossRef CAS PubMed.
- T. Baby, Y. Liu, A. P. J. Middelberg and C. X. Zhao, Chem. Eng. Sci., 2017, 169, 128–139 CrossRef CAS.
- J. P. Ma and C. W. Li, Sens. Actuators, B, 2018, 262, 236–244 CrossRef CAS.
- D. R. Reyes, H. van Heeren, S. Guha, L. Herbertson, A. P. Tzannis, J. Ducree, H. Bissig and H. Becker, Lab Chip, 2021, 21, 9–21 RSC.
- E. Sah and H. Sah, J. Nanomater., 2015, 2015, 794601, DOI:10.1155/2015/794601.
- Q. Feng, L. Zhang, C. Liu, X. Li, G. Hu, J. Sun and X. Jiang, Biomicrofluidics, 2015, 9, 052604 CrossRef PubMed.
- R. Ran, Q. Sun, T. Baby, D. Wibowo, A. P. Middelberg and C.-X. Zhao, Chem. Eng. Sci., 2017, 169, 78–96 CrossRef CAS.
- A.-S. Yang, F.-C. Chuang, C.-K. Chen, M.-H. Lee, S.-W. Chen, T.-L. Su and Y.-C. Yang, Chem. Eng. J., 2015, 263, 444–451 CrossRef CAS.
- D. Liu, H. Zhang, F. Fontana, J. T. Hirvonen and H. A. Santos, Lab Chip, 2017, 17, 1856–1883 RSC.
- J. P. Martins, G. Torrieri and H. A. Santos, Expert Opin. Drug Delivery, 2018, 15, 469–479 CrossRef CAS PubMed.
- K. V. Sharp and R. J. Adrian, Exp. Fluids, 2004, 36, 741–747 CrossRef CAS.
- J. Atencia and D. J. Beebe, Nature, 2005, 437, 648–655 CrossRef CAS PubMed.
- K. F. Glass, E. Longmire and A. Hubel, Int. J. Heat Mass Transfer, 2008, 51, 5749–5757 CrossRef PubMed.
- P. Kunal, E. J. Roberts, C. T. Riche, K. Jarvis, N. Malrnstadt, R. L. Brutchey and S. M. Humphrey, Chem. Mater., 2017, 29, 4341–4350 CrossRef CAS.
- G. Laffite, C. Leroy, C. Bonhomme, L. Bonhomme-Coury, E. Letavernier, M. Daudon, V. Frochot, J. P. Haymann, S. Rouziere, I. T. Lucas, D. Bazin, F. Babonneau and A. Abou-Hassan, Lab Chip, 2016, 16, 1157–1160 RSC.
- J. Marschewski, S. Jung, P. Ruch, N. Prasad, S. Mazzotti, B. Michel and D. Poulikakos, Lab Chip, 2015, 15, 1923–1933 RSC.
- D. Holzinger and A. Ehresmann, Microfluid. Nanofluid., 2015, 19, 395–402 CrossRef CAS.
- J. P. Ma, S. M. Y. Lee, C. Q. Yi and C. W. Li, Lab Chip, 2017, 17, 209–226 RSC.
- A. D. Stroock, S. K. Dertinger, G. M. Whitesides and A. Ajdari, Anal. Chem., 2002, 74, 5306–5312 CrossRef CAS PubMed.
- A. Olanrewaju, M. Beaugrand, M. Yafia and D. Juncker, Lab Chip, 2018, 18, 2323–2347 RSC.
- T. S. Kaminski and P. Garstecki, Chem. Soc. Rev., 2017, 46, 6210–6226 RSC.
- H. H. Jeong, D. Issadore and D. Lee, Korean J. Chem. Eng., 2016, 33, 1757–1766 CrossRef CAS.
- R. Othman, G. T. Vladisavljevic, H. C. H. Bandulasena and Z. K. Nagy, Chem. Eng. J., 2015, 280, 316–329 CrossRef CAS.
- J. Jung, S. C. Cao, Y. H. Shin, R. I. Al-Raoush, K. Alshibli and J. W. Choi, Microsyst. Technol., 2018, 24, 1071–1080 CrossRef CAS.
- A. L. R. Costa, A. Gomes and R. L. Cunha, Exp. Therm. Fluid Sci., 2017, 85, 167–175 CrossRef CAS.
- A. Fabozzi, R. Vitiello, I. R. Krauss, M. Iuliano, G. De Tommaso, A. Amoresano, G. Pinto, L. Paduano, C. Jones, M. Di Serio and G. D'Errico, J. Surfactants Deterg., 2019, 22, 115–124 CrossRef CAS.
- A. Fabozzi, I. R. Krauss, R. Vitiello, M. Fornasier, L. Sicignano, S. King, S. Guido, C. Jones, L. Paduano, S. Murgia and G. D'Errico, J. Colloid Interface Sci., 2019, 552, 448–463 CrossRef CAS PubMed.
- Z. W. Zhou, H. P. Li, K. K. Wang, Q. Guo, C. Z. Li, H. L. Jiang, Y. Q. Hu, D. Oupicky and M. J. Sun, ACS Appl. Mater. Interfaces, 2017, 9, 14576–14589 CrossRef CAS PubMed.
- D. F. Liu, S. Cito, Y. Z. Zhang, C. F. Wang, T. M. Sikanen and H. A. Santos, Adv. Mater., 2015, 27, 2298–2304 CrossRef CAS PubMed.
- S. K. Dertinger, D. T. Chiu, N. L. Jeon and G. M. Whitesides, Anal. Chem., 2001, 73, 1240–1246 CrossRef CAS.
- Z. Nie, S. Xu, M. Seo, P. C. Lewis and E. Kumacheva, J. Am. Chem. Soc., 2005, 127, 8058–8063 CrossRef CAS PubMed.
- X. Luo, P. Su, W. Zhang and C. L. Raston, Adv. Mater. Technol., 2019, 1900488, DOI:10.1002/Admt.201900488.
- A. Tiwari, A. Maheshwari, V. M. Rajesh and K. B. Singh, Chem. Eng. J., 2019, 377, 120602 CrossRef CAS.
- L. Zha, M. J. Shang, M. Qiu, H. Zhang and Y. H. Su, Chem. Eng. Sci., 2019, 195, 62–73 CrossRef CAS.
- K. Shahzad, W. Van Aeken, M. Mottaghi, V. K. Kamyab and S. Kuhn, Microfluid. Nanofluid., 2018, 22, 104 CrossRef PubMed.
- A. M. Streets and Y. Huang, Biomicrofluidics, 2013, 7, 011302 CrossRef PubMed.
- A. Woźniak, A. Malankowska, G. Nowaczyk, B. F. Grześkowiak, K. Tuśnio, R. Słomski, A. Zaleska-Medynska and S. Jurga, J. Mater. Sci.: Mater. Med., 2017, 28, 1–11 CrossRef PubMed.
- Y. Huang, T. Han, J. Xuan, H. Xu, Y. Wang and L. Zhang, J. Micromech. Microeng., 2018, 28, 105021 CrossRef.
- S. Nawar, J. K. Stolaroff, C. Ye, H. Wu, F. Xin and D. A. Weitz, Lab Chip, 2020, 20, 147–154 RSC.
- Z. Lian, Y. Chan, Y. Luo, X. Yang, K. S. Koh, J. Wang, G. Z. Chen, Y. Ren and J. He, Electrophoresis, 2020, 41, 891–901 CrossRef CAS PubMed.
- G. Tetradis-Meris, D. Rossetti, C. N. Pulido de Torres, R. Cao, G. Lian and R. Janes, Ind. Eng. Chem. Res., 2009, 48, 8881–8889 CrossRef CAS.
- G. T. Vladisavljević, N. Khalid, M. A. Neves, T. Kuroiwa, M. Nakajima, K. Uemura, S. Ichikawa and I. Kobayashi, Adv. Drug Delivery Rev., 2013, 65, 1626–1663 CrossRef PubMed.
- A. Fabozzi, F. Della Sala, M. di Gennaro and A. Borzacchiello, New J. Chem., 2022, 46, 19763–19772 RSC.
- N. M. Belliveau, J. Huft, P. J. Lin, S. Chen, A. K. Leung, T. J. Leaver, A. W. Wild, J. B. Lee, R. J. Taylor and Y. K. Tam, Mol. Ther.–Nucleic Acids, 2012, 1, e37 CrossRef PubMed.
- H. K. Mandl, E. Quijano, H. W. Suh, E. Sparago, S. Oeck, M. Grun, P. M. Glazer and W. M. Saltzman, J. Controlled Release, 2019, 314, 92–101 CrossRef CAS PubMed.
- J. Riewe, P. Erfle, S. Melzig, A. Kwade, A. Dietzel and H. Bunjes, Int. J. Pharm., 2020, 579, 119167 CrossRef CAS PubMed.
- X. Zhu, C. Vo, M. Taylor and B. R. Smith, Mater. Horiz., 2019, 6, 1094–1121 RSC.
- Y. M. Cao, L. Silverman, C. H. Lu, R. Hof, J. E. Wulff and M. G. Moffitt, Mol. Pharmaceutics, 2019, 16, 96–107 CrossRef CAS PubMed.
- M. Russo, P. Bevilacqua, P. A. Netti and E. Torino, Sci. Rep., 2016, 6, 37906 CrossRef CAS PubMed.
- F. Della Sala, A. Fabozzi, M. di Gennaro, S. Nuzzo, P. Makvandi, N. Solimando, M. Pagliuca and A. Borzacchiello, Macromol. Biosci., 2021, 2100304, DOI:10.1002/Mabi.202100304.
- V. P. Galvan-Chacon, L. Costa, D. Barata and P. Habibovic, Acta Biomater., 2021, 128, 486–501 CrossRef CAS PubMed.
- R. Zoqlam, C. J. Morris, M. Akbar, A. M. Alkilany, S. I. Hamdallah, P. Belton and S. Qi, Mater. Sci. Eng. C, 2021, 127, 112243 CrossRef CAS PubMed.
- Y. Y. Chai, Y. F. Wang, B. Q. Li, W. Qi, R. X. Su and Z. M. He, Langmuir, 2021, 37, 7219–7226 CrossRef CAS PubMed.
- E. Chiesa, F. Riva, R. Dorati, A. Greco, S. Ricci, S. Pisani, M. Patrini, T. Modena, B. Conti and I. Genta, Pharmaceutics, 2020, 12(3) DOI:10.3390/pharmaceutics12030260.
- M. Ghasemi Toudeshkchouei, P. Zahedi and A. Shavandi, Materials, 2020, 13, 1483 CrossRef PubMed.
- M. Brzezinski, M. Socka, T. Makowski, B. Kost, M. Cieslak and K. Krolewska-Golinska, Colloids Surf., B, 2021, 201, 111598 CrossRef CAS PubMed.
- M. Danaei, M. Dehghankhold, S. Ataei, F. Hasanzadeh Davarani, R. Javanmard, A. Dokhani, S. Khorasani and M. Mozafari, Pharmaceutics, 2018, 10, 57 CrossRef PubMed.
- W. Xu, X. Jiang and L. Huang, Compr. Biotechnol., 2019, 560 CAS.
-
M. H. Amer, Molecular and cellular therapies, 2014, vol. 2, pp. 1–19 Search PubMed.
- A. Akinc, M. A. Maier, M. Manoharan, K. Fitzgerald, M. Jayaraman, S. Barros, S. Ansell, X. Du, M. J. Hope and T. D. Madden, Nat. Nanotechnol., 2019, 14, 1084–1087 CrossRef CAS PubMed.
- S. Chen, Y. Y. C. Tam, P. J. Lin, M. M. Sung, Y. K. Tam and P. R. Cullis, J. Controlled Release, 2016, 235, 236–244 CrossRef CAS PubMed.
- S. C. Semple, A. Akinc, J. Chen, A. P. Sandhu, B. L. Mui, C. K. Cho, D. W. Sah, D. Stebbing, E. J. Crosley and E. Yaworski, Nat. Biotechnol., 2010, 28, 172–176 CrossRef CAS PubMed.
- M. Jayaraman, S. M. Ansell, B. L. Mui, Y. K. Tam, J. Chen, X. Du, D. Butler, L. Eltepu, S. Matsuda and J. K. Narayanannair, Am. Ethnol., 2012, 124, 8657–8661 Search PubMed.
- P. R. Cullis and M. J. Hope, Mol. Ther., 2017, 25, 1467–1475 CrossRef CAS PubMed.
- I. V. Zhigaltsev, N. Belliveau, I. Hafez, A. K. Leung, J. Huft, C. Hansen and P. R. Cullis, Langmuir, 2012, 28, 3633–3640 CrossRef CAS PubMed.
- S. J. Shepherd, C. C. Warzecha, S. Yadavali, R. El-Mayta, M.-G. Alameh, L. Wang, D. Weissman, J. M. Wilson, D. Issadore and M. J. Mitchell, Nano Lett., 2021, 21, 5671–5680 CrossRef CAS PubMed.
- Y. Li, R. J. Lee, X. Huang, Y. Li, B. Lv, T. Wang, Y. Qi, F. Hao, J. Lu and Q. Meng, Nanomedicine, 2017, 13, 371–381 CrossRef CAS PubMed.
- B. Magenheim, M. Levy and S. Benita, Int. J. Pharm., 1993, 94, 115–123 CrossRef CAS.
- G. P. Zara, R. Cavalli, A. Fundarò, A. Bargoni, O. Caputo and M. R. Gasco, Pharmacol. Res., 1999, 40, 281–286 CrossRef CAS PubMed.
- R. Pignatello, A. Leonardi, V. Fuochi, G. Petronio Petronio, A. S. Greco and P. M. Furneri, Nanomaterials, 2018, 8, 304 CrossRef PubMed.
- A. zur Mühlen, C. Schwarz and W. Mehnert, Eur. J. Pharm. Biopharm., 1998, 45, 149–155 CrossRef PubMed.
- M. M. Billingsley, N. Singh, P. Ravikumar, R. Zhang, C. H. June and M. J. Mitchell, Nano Lett., 2020, 20, 1578–1589 CrossRef CAS PubMed.
- K. T. Love, K. P. Mahon, C. G. Levins, K. A. Whitehead, W. Querbes, J. R. Dorkin, J. Qin, W. Cantley, L. L. Qin and T. Racie, Proc. Natl. Acad. Sci. U. S. A., 2010, 107, 1864–1869 CrossRef CAS PubMed.
- W. Mehnert and K. Mäder, Adv. Drug Delivery Rev., 2012, 64, 83–101 CrossRef.
- A. J. Domb, Int. J. Pharm., 1995, 124, 271–278 CrossRef CAS.
- K. Westesen and B. Siekmann, Int. J. Pharm., 1997, 151, 35–45 CrossRef CAS.
- R. Lander, W. Manger, M. Scouloudis, A. Ku, C. Davis and A. Lee, Biotechnol. Prog., 2000, 16, 80–85 CrossRef CAS PubMed.
- L. B. Peres, L. B. Peres, P. H. H. de Araújo and C. Sayer, Colloids Surf., B, 2016, 140, 317–323 CrossRef PubMed.
-
S. Benita, Submicron emulsions in drug targeting and delivery, CRC Press, 1998 Search PubMed.
- V. Jenning, A. Gysler, M. Schäfer-Korting and S. H. Gohla, Eur. J. Pharm. Biopharm., 2000, 49, 211–218 CrossRef CAS PubMed.
- R. B. P. Moura, L. M. Andrade, L. Alonso, A. Alonso, R. N. Marreto and S. F. Taveira, Eur. J. Pharm. Sci., 2022, 168, 106048 CrossRef CAS PubMed.
- R. L. Ball, K. A. Hajj, J. Vizelman, P. Bajaj and K. A. Whitehead, Nano Lett., 2018, 18, 3814–3822 CrossRef CAS PubMed.
- A. Albanese, P. S. Tang and W. C. Chan, Annu. Rev. Biomed. Eng., 2012, 14, 1–16 CrossRef CAS PubMed.
- X. Zhao, F. Bian, L. Sun, L. Cai, L. Li and Y. Zhao, Small, 2020, 16, 1901943 CrossRef CAS PubMed.
- C. Martins, F. Araújo, M. J. Gomes, C. Fernandes, R. Nunes, W. Li, H. A. Santos, F. Borges and B. Sarmento, Eur. J. Pharm. Biopharm., 2019, 138, 111–124 CrossRef CAS PubMed.
- R. Karnik, F. Gu, P. Basto, C. Cannizzaro, L. Dean, W. Kyei-Manu, R. Langer and O. C. Farokhzad, Nano Lett., 2008, 8, 2906–2912 CrossRef CAS PubMed.
- N. Kimura, M. Maeki, K. Sasaki, Y. Sato, A. Ishida, H. Tani, H. Harashima and M. Tokeshi, RSC Adv., 2021, 11, 1430–1439 RSC.
- I. Arduino, Z. Liu, A. Rahikkala, P. Figueiredo, A. Correia, A. Cutrignelli, N. Denora and H. A. Santos, Acta Biomater., 2021, 121, 566–578 CrossRef CAS PubMed.
- A. Gordillo-Galeano and C. E. Mora-Huertas, Eur. J. Pharm. Biopharm., 2018, 133, 285–308 CrossRef CAS PubMed.
- S. Indoria, V. Singh and M.-F. Hsieh, Int. J. Pharm., 2020, 582, 119314 CrossRef CAS PubMed.
- R. Varghese, S. Salvi, P. Sood, B. Kulkarni and D. Kumar, Colloid Interface Sci. Commun., 2022, 46, 100561 CrossRef CAS.
- A. Dalmoro, S. Bochicchio, S. F. Nasibullin, P. Bertoncin, G. Lamberti, A. A. Barba and R. I. Moustafine, Eur. J. Pharm. Sci., 2018, 121, 16–28 CrossRef CAS PubMed.
- N. Tahir, A. Madni, W. Li, A. Correia, M. M. Khan, M. A. Rahim and H. A. Santos, Int. J. Pharm., 2020, 581, 119275 CrossRef CAS PubMed.
- F. Zahir-Jouzdani, F. Mottaghitalab, M. Dinarvand and F. Atyabi, J. Drug Delivery Sci. Technol., 2018, 45, 428–441 CrossRef CAS.
- K. Dua, R. Wadhwa, G. Singhvi, V. Rapalli, S. D. Shukla, M. D. Shastri, G. Gupta, S. Satija, M. Mehta and N. Khurana, Drug Dev. Res., 2019, 80, 714–730 CrossRef CAS PubMed.
- M. A. Subhan and V. Torchilin, Transl. Res., 2019, 214, 62–91 CrossRef CAS PubMed.
- P. M. Valencia, P. A. Basto, L. Zhang, M. Rhee, R. Langer, O. C. Farokhzad and R. Karnik, ACS Nano, 2010, 4, 1671–1679 CrossRef CAS PubMed.
- P. C. Mondal, D. Asthana, R. K. Parashar and S. Jadhav, Mater. Adv., 2021, 2, 7620–7637 RSC.
- J. Nam, N. Won, J. Bang, H. Jin, J. Park, S. Jung, S. Jung, Y. Park and S. Kim, Adv. Drug Delivery Rev., 2013, 65, 622–648 CrossRef CAS PubMed.
- S. M. Ng, M. Koneswaran and R. Narayanaswamy, RSC Adv., 2016, 6, 21624–21661 RSC.
- Z. Li, T. Zhuang, J. Dong, L. Wang, J. Xia, H. Wang, X. Cui and Z. Wang, Ultrason. Sonochem., 2021, 71, 105384 CrossRef CAS PubMed.
- M. Liong, J. Lu, M. Kovochich, T. Xia, S. G. Ruehm, A. E. Nel, F. Tamanoi and J. I. Zink, ACS Nano, 2008, 2, 889–896 CrossRef CAS PubMed.
- J. Shen, M. Shafiq, M. Ma and H. Chen, Nanomaterials, 2020, 10, 1177 CrossRef CAS PubMed.
- D.-Y. Kim, S. H. Jin, S.-G. Jeong, B. Lee, K.-K. Kang and C.-S. Lee, Sci. Rep., 2018, 8, 1–11 Search PubMed.
- M. Mohammad, A. Razmjou, K. Liang, M. Asadnia and V. Chen, ACS Appl. Mater. Interfaces, 2018, 11, 1807–1820 CrossRef PubMed.
- G.-X. Li, Q. Li, R. Cheng and S. Chen, Curr. Opin. Chem. Eng., 2020, 29, 34–41 CrossRef.
- L. L. Li, X. Li and H. Wang, Small Methods, 2017, 1, 1700140 CrossRef.
- Y. Cao, Y. Xie, L. Liu, A. Xiao, Y. Li, C. Zhang, X. Fang and Y. Zhou, Phytochem. Rev., 2017, 16, 555–563 CrossRef CAS.
- J. Yan, X. Xu, J. Zhou, C. Liu, L. Zhang, D. Wang, F. Yang and H. Zhang, ACS Appl. Bio Mater., 2020, 3, 1216–1225 CrossRef CAS PubMed.
- N. Hao, Y. Nie, A. Tadimety, A. B. Closson and J. X. Zhang, Mater. Res. Lett., 2017, 5, 584–590 CrossRef CAS PubMed.
- D. Ding, C. C. Goh, G. Feng, Z. Zhao, J. Liu, R. Liu, N. Tomczak, J. Geng, B. Z. Tang and L. G. Ng, Adv. Mater., 2013, 25, 6083–6088 CrossRef CAS PubMed.
- K. Li, W. Qin, D. Ding, N. Tomczak, J. Geng, R. Liu, J. Liu, X. Zhang, H. Liu and B. Liu, Sci. Rep., 2013, 3, 1–10 Search PubMed.
- C. Tong, X. Zhong, Y. Yang, X. Liu, G. Zhong, C. Xiao, B. Liu, W. Wang and X. Yang, Biomaterials, 2020, 243, 119936 CrossRef CAS PubMed.
- X. Li, M. Zha, Y. Li, J. S. Ni, T. Min, T. Kang, G. Yang, H. Tang, K. Li and X. Jiang, Angew. Chem., Int. Ed., 2020, 59, 21899–21903 CrossRef CAS PubMed.
- F. Gu, L. Zhang, B. A. Teply, N. Mann, A. Wang, A. F. Radovic-Moreno, R. Langer and O. C. Farokhzad, Proc. Natl. Acad. Sci. U. S. A., 2008, 105, 2586–2591 CrossRef CAS PubMed.
- V. Bagalkot, L. Zhang, E. Levy-Nissenbaum, S. Jon, P. W. Kantoff, R. Langer and O. C. Farokhzad, Nano Lett., 2007, 7, 3065–3070 CrossRef CAS PubMed.
- Y. L. Balachandran, X. Li and X. Jiang, Nano Lett., 2021, 21, 1335–1344 CrossRef CAS PubMed.
- Y. Gu, M. Yoshikiyo, A. Namai, D. Bonvin, A. Martinez, R. Piñol, P. Téllez, N. J. Silva, F. Ahrentorp and C. Johansson, RSC Adv., 2020, 10, 28786–28797 RSC.
- J. Verma, S. Lal and C. J. Van Noorden, Int. J. Nanomed., 2014, 9, 2863 Search PubMed.
- H. Fatima, T. Charinpanitkul and K.-S. Kim, Nanomaterials, 2021, 11, 1203 CrossRef CAS PubMed.
- S. Maenosono and S. Saita, IEEE Trans. Magn., 2006, 42, 1638–1642 CrossRef CAS.
- N. Griffete, J. Fresnais, A. Espinosa, C. Wilhelm, A. Bée and C. Ménager, Nanoscale, 2015, 7, 18891–18896 RSC.
- I. Apostolova and J. Wesselinowa, Solid State Commun., 2009, 149, 986–990 CrossRef CAS.
- J. Wallyn, N. Anton and T. F. Vandamme, Pharmaceutics, 2019, 11, 601 CrossRef CAS PubMed.
- A. Hervault and N. T. K. Thanh, Nanoscale, 2014, 6, 11553–11573 RSC.
- A. Amarjargal, L. D. Tijing, I.-T. Im and C. S. Kim, Chem. Eng. J., 2013, 226, 243–254 CrossRef CAS.
- K. Wu, D. Su, J. Liu, R. Saha and J.-P. Wang, Nanotechnology, 2019, 30, 502003 CrossRef CAS PubMed.
- X. Cai, Q. Zhu, Y. Zeng, Q. Zeng, X. Chen and Y. Zhan, Int. J. Nanomed., 2019, 14, 8321 CrossRef CAS PubMed.
- R. O. Rodrigues, P. C. Sousa, J. Gaspar, M. Bañobre-López, R. Lima and G. Minas, Small, 2020, 16, 2003517 CrossRef CAS PubMed.
- Y. Chang, J. Jiang, W. Chen, W. Yang, L. Chen, P. Chen, J. Shen, S. Qian, T. Zhou and L. Wu, Appl. Mater. Today, 2020, 18, 100492 CrossRef PubMed.
- A. Aftab, S. Bashir, S. Rafique, T. Ghani, R. Khan, M. Bashir, A. Ehsan, M. I. Khan, A. U. Shah and A. Mahmood, Appl. Nanosci., 2020, 10, 2281–2293 CrossRef CAS.
- F. Tian, L. Cai, C. Liu and J. Sun, Lab Chip, 2022, 22, 512–529 RSC.
|
This journal is © The Royal Society of Chemistry 2023 |
Click here to see how this site uses Cookies. View our privacy policy here.